CHAPTER 2 Evolution of Life on Earth
No one is certain how life began, but the common ancestor of all living things populated the earth over 3 billion years ago, not long (geologically speaking) after the planet formed 4.5 billion years ago (Fig. 2-1). Biochemical features shared by all existing cells suggest that this primitive microscopic cell had about 600 genes encoded in DNA, ribosomes to synthesize proteins, and a plasma membrane with pumps, carriers, and channels. Over time, mutations in the DNA created progeny that diverged genetically into numerous distinctive species, numbering about 1.7 million known to science. The total number of species living on the earth today is unknown but is estimated to be between 4 million and 100 million. On the basis of evolutionary histories preserved in their genomes, living organisms are divided into three primary domains: Bacteria, Archaea, and Eucarya.
Evolution is the great unifying principle in biology. Research on evolution is both exciting and challenging because this ultimate detective story involves piecing together fragmentary evidence spread over 3.5 billion years. Data include fossils of ancient organisms preserved in stone, ancient DNA (going back about 45,000 years), and especially DNA of living organisms.
Prebiotic Chemistry Leading to an RNA World
But where did the common ancestor come from? A wide range of evidence supports the idea that life began with self-replicating RNA polymers sheltered inside lipid vesicles even before the invention of protein synthesis (Fig. 2-2). This hypothetical early stage of evolution is called the RNA World. This postulate is attractive because it solves the chicken-and-egg problem of how to build a system of self-replicating molecules without having to invent either DNA or proteins on their own. Clearly, RNA has an advantage, because it provides a way to store information in a type of molecule that can also have catalytic activity. Proteins excel in catalysis but do not store self-replicating genetic information. Today, proteins have largely superseded RNAs as cellular catalysts. DNA excels for storing genetic information, since the absence of the 2′ hydroxyl makes it less reactive and therefore more stable than RNA. Readers who are not familiar with the structure of nucleic acids should consult Chapter 3 at this point.
Given a supply of nucleotides, these reactions could have created a heterogeneous pool of small RNAs, the biochemical materials required to set in motion the process of natural selection at the molecular level. The idea is that random sequences of RNA are selected for replication on the basis of useful attributes. This process of molecular evolution can now be reproduced in the laboratory by using multiple rounds of error-prone replication of RNA to produce variants from a pool of random initial sequences. Given a laboratory assay for a particular function, it is possible to use this process of directed evolution to select RNAs that are capable of catalyzing biochemical reactions (called ribozymes), including RNA-dependent synthesis of a complementary RNA strand. Although unlikely, this is presumed to have occurred in nature, creating a reliable mechanism to replicate RNAs. Subsequent errors in replication produced variant RNAs, some having desirable features such as catalytic activities that were required for a self-replicating system. Over millions of years, a ribozyme eventually evolved with the ability to catalyze the formation of peptide bonds and to synthesize proteins. This most complicated of all known ribozymes is, of course, the ribosome (see Fig. 17-6) that catalyzes the synthesis of proteins. Proteins eventually supplanted ribozymes as catalysts for most biochemical reactions. Owing to greater chemical stability, DNA proved to be superior to RNA for storing the genetic blueprint over time.
Each of these events is improbable, and their combined probability is exceedingly remote, but given a vast number of chemical “experiments” over hundreds of millions of years, this all happened. Encapsulation of these prebiotic reactions may have enhanced their probability. In addition to catalyzing RNA synthesis, clay minerals can also promote formation of lipid vesicles, which can corral reactants to avoid dilution and loss of valuable constituents. This process might have started with fragile bilayers of fatty acids that were later supplanted by more robust phosphoglyceride bilayers (see Fig. 7-5). In laboratory experiments, RNAs inside lipid vesicles can create osmotic pressure that favors expansion of the bilayer at the expense of vesicles lacking RNAs.
Another mystery is how l-amino acids and d-sugars (see Chapter 3) were selected over their stereoisomers for biomacromolecules. This was a pivotal event, since racemic mixtures are not favorable for biosynthesis. For example, mixtures of nucleotides composed of l- and d-ribose cannot base-pair well enough for template-guided replication of nucleic acids. In the laboratory, particular amino acid stereoisomers (that could have come from meteorites) can bias the synthesis of D-sugars.
Divergent Evolution from the Last Universal Common Ancestor of Life
Shared biochemical features suggest that all current cells are derived from a last universal common ances-tor about 3.5 billion years ago (Fig. 2-1). This primitive ancestor could, literally, have been a single cell or colony of cells, but it might have been a larger community of cells sharing a common pool of genes through interchange of their nucleic acids. The situation is obscure because no primitive organisms remain. All contemporary organisms have diverged equally far in time from their common ancestor.
During evolution genomes have diversified by three processes (Fig. 2-3):
Evolution of Prokaryotes
Since the beginning of life, microorganisms dominated the earth in terms of numbers, variety of species, and range of habitats (Fig. 2-4). Bacteria and Archaea remain the most abundant organisms in the seas and on land. They share many features, including basic metabolic enzymes and flagella powered by rotary motors embedded in the plasma membrane. Both divisions of prokaryotes are diverse with respect to size, shape, nutrient sources, and environmental tolerances, so these features cannot be used for classification, which relies instead on analysis of their genomes. For example, sequences of the genes for ribosomal RNAs cleanly separate Bacteria and Archaea (Fig. 2-4). Bacteria are also distinguished by plasma membranes of phosphoglycerides (see Fig. 7-5) with F-type adenosine triphosphatases (ATPases) that use proton gradients to synthesize adenosine triphosphate (ATP). Archaea have plasma membranes composed of isoprenyl ether lipids and V-type ATPases that can either pump protons or synthesize ATP (see Fig. 8-5).
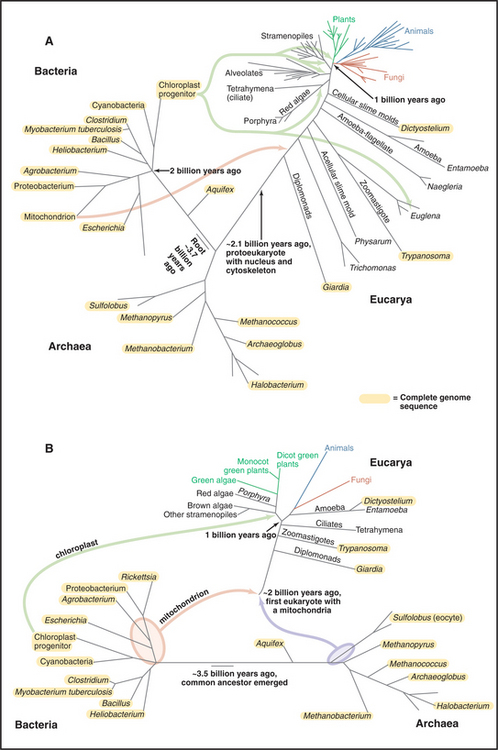
Figure 2-4 comparison of trees of life. A, Universal tree based on comparisons of ribosomal RNA sequences. The rRNA tree has its root deep in the bacterial lineage 3 billion to 4 billion years ago. All current organisms, arrayed at the ends of branches, fall into three domains: Bacteria, Archaea, and Eucarya (eukaryotes). This analysis assumes that the organisms in the three domains diverged from a common ancestor. The lengths of the segments and branches are based solely on differences in RNA sequences. Because the rate of random changes in rRNA genes has not been constant, the lengths of the lines that lead to contemporary organisms are not equal. Fossil records provide estimated times of a few key events. Complete sequences of some genomes (orange; see http://www.tigr.org) verify most aspects of this tree but also show that genes have moved laterally between Bacteria and Archaea and within each of these domains. Multiple bacterial genes moved to Eucarya twice: First, an α-proteobacterium fused with a primitive eukaryote, giving rise to mitochondria that subsequently transferred many of their genes to the eukaryotic nucleus; and second, a cyanobacterium fused with the precursor of algae and plants to give rise to chloroplasts. Organisms formerly classified as algae, as well as organisms formerly classified elsewhere, actually belong to four large branches near the top of the tree: alveolates (including dinoflagellates, ciliates, and sporozoans), stramenopiles (including diatoms and brown algae), rhodophytes (red algae), and plants (including the green algae). B, Composite tree based on analysis of full genome sequences and other data. This hypothesis assumes that eukaryotes formed by fusion of an α-proteobacterium with an Archaean. Chloroplasts arose from the fusion of a cyanobacterium with the eukaryotic precursor of algae and plants.
(A, Original drawing, adapted from a branching pattern from Sogin M, Marine Biological Laboratory, Woods Hole, Massachusetts. Reference: Pace N: A molecular view of microbial diversity and the biosphere. Science 276:734–740, 1997. B, Original drawing, based on multiple sources.)
Chlorophyll-based photosynthesis originated in Bacteria around 3 billion years ago. Surely, this was one of the most remarkable events during the evolution of life on the earth, because photosynthetic reaction centers (see Fig. 19-8) require not only genes for several transmembrane proteins but also genes for multiple enzymes to synthesize chlorophyll and other complex organic molecules associated with the proteins. Chapter 19 describes the machinery and mechanisms of photosynthesis.
Even more remarkably, photosynthesis was invented and perfected not once but twice in different bacteria. A progenitor of green sulfur bacteria and heliobacteria developed photosystem I, while a progenitor of purple bacteria and green filamentous bacteria developed photosystem II. About 2.5 billion years ago, a momentous lateral transfer event brought the genes for the two photosystems together in cyanobacteria, arguably the most important organisms in the history of the earth. Cyanobacteria (formerly misnamed blue-green algae) use an enzyme containing manganese to split water into oxygen, electrons, and protons. Sunlight energizes photosystem II and photosystem I to pump the protons out of the cell, creating a proton gradient that is used to synthesize ATP (see Chapters 8 and 19). Using sunlight as the energy source, this form of photosynthesis is the primary source of energy to synthesize the organic compounds that many other forms of life depend on for energy. In addition, beginning about 2.4 billion years ago, cyanobacteria produced most of the oxygen in the earth’s atmosphere as a by-product of photosynthesis, bioengineering the planet and radically changing the chemical environment for all other organisms as well.
Origin of Eukaryotes
Therefore, researchers must analyze genome se-quences to test hypotheses about the origins of eukaryotes. The mathematical methods required to analyze the genomic data are still being perfected, and the events are so ancient that their reconstruction is challenging. The bacterial ancestor donated genes for many metabolic processes carried out in the cytoplasm. The archaeal ancestor provided many distinctive genes for informational processes such as transcription of DNA into RNA and translation of RNA into protein. This explains why eukaryotes and Archaea are neighbors on molecular phylogenies based on rRNA sequences (Fig. 2-4).
One attractive hypothesis is that cells from the two domains of prokaryotes joined in a symbiotic relationship to form the first eukaryote (Fig. 2-5). The identities of the Bacterium and Archaean that merged to form this hybrid cell are not known, since these were cells that lived 2 billion years ago. Such a fusion with massive lateral transfer of genes into the new organism provides a simple explanation for how both types of prokaryotes contributed to eukaryotic genomes well after their forebears diverged from the common ancestor. If two prokaryotes literally fused, then their genomes would have been in the same cytoplasm. Later, the hybrid genome was surrounded by membranes to become the nucleus, and another proteobacterium was engulfed to form the precursor of the mitochondrion.
Origin and Evolution of Mitochondria
Overwhelming molecular evidence has established that eukaryotes acquired mitochondria when an α-proteobacterium became an endosymbiont. Modern-day α-proteobacteria include pathogenic Rickettsias. When the two formerly independent cells established a stable, endosymbiotic relationship, the Bacterium contributed molecular machinery for ATP synthesis by oxidative phosphorylation (see Fig. 19-5). The host cell might have supplied organic substrates to fuel ATP synthesis. Together, they had a reliable energy supply for processes such as biosynthesis, regulation of the internal ionic environment, and cellular motility. Given that some primitive eukaryotes lack full-fledged mitochondria, the singular event that created mitochondria was believed to have occurred well after eukaryotes branched from prokaryotes.
An alternative idea is that the recipient of the α-proteobacterium was an archaean cell rather than a eukaryote (Fig. 2-5). If so, this union could have created not only the mitochondrion but also the first eukaryote! This parsimonious hypothesis is consistent with some but not all of the available data, so it is currently impossible to rule out other scenarios.
The mitochondrial progenitor brought along its own genome and biosynthetic machinery, but over many years of evolution, most bacterial genes either moved to the host cell nucleus or were lost. Like their bacterial ancestors, mitochondria are enclosed by two membranes, with the inner membrane equipped for synthesis of ATP. Mitochondria maintain a few genes for mitochondrial components and the capacity to synthesize proteins. Nuclear genes encode most mitochondrial proteins, which are synthesized in the cytoplasm and imported into the organelle (see Fig. 18-2). The transfer of bacterial genes to the nucleus sealed the dependence of the organelle on its eukaryotic host.
The First Billion Years of Eukaryotic Evolution
What is unique about eukaryotes? For years, it was believed that a membrane-bounded nucleus and a cytoskeleton set eukaryotes apart from prokaryotes. However, some Bacteria and Archaea have genes for homologs of the cytoskeletal proteins, actin, tubulin, and intermediate filaments. Although nuclei are rare in prokaryotes, a family of Bacteria called planctomycetes have rudimentary nuclei that also include all of the ribosomes. Thus, the three kingdoms of life have more in common than was appreciated in the past, as is fitting from our new appreciation for their common origins.
Molecular phylogenies (Fig. 2-4) indicate that modern eukaryotic lineages began to diverge during the period between 2 billion and 1 billion years ago. Since modern organisms from the earliest branches have nuclei, membrane-bounded organelles, and complex structures, including cilia for locomotion, much of what it takes to be a eukaryote evolved very early. These features require hundreds of genes that are absent from prokaryotes, but no fossils or other direct evidence are available about these early events. Organisms on early branches lack a few basic functions, such as the full machinery required for actin-based locomotion and cytokinesis, so the required genes likely appeared after their divergence.
Heterotrophic prokaryotes that obtain nutrients from a variety of sources appear to have carried out the first evolutionary experiment with compartmentalization (Fig. 2-6A). However, these prokaryotes are compartmentalized only in the sense that they separate digestion outside the cell from biosynthesis inside the cell. They export digestive enzymes (either free or attached to the cell surface) to hydrolyze complex organic macromolecules (see Fig. 18-10). They must then import the products of digestion to provide building blocks for new macromolecules. Evolution of the proteins required for targeting and translocation of proteins across membranes was a prokaryotic innovation that set the stage for compartmentalization in eukaryotes.
More sophisticated compartmentalization might have begun when a primitive prokaryote developed the capacity to segregate protein complexes with like functions in the plane of the plasma membrane. This created functionally distinct subdomains. Present-day Bacteria segregate their plasma membranes into domains specialized for energy production or protein translocation. Invagination of such domains might have created the endoplasmic reticulum (ER), Golgi apparatus, and lysosomes, as speculated in the following paragraphs (Fig. 2-6):
The outcome of these events (Fig. 2-7) was a vacuolar system consisting of the ER, the center for protein translocation and lipid synthesis; the Golgi complex and secretory pathway, for posttranslational modification and distribution of biosynthetic products to different destinations; and the endosome/lysosome system, for uptake and digestion.
Production of oxygen by photosynthetic cyanobacteria raised the concentration of atmospheric oxygen about 2.2 billion years ago. This provided sufficient molecular oxygen for eukaryotic cells to synthesize cholesterol (see Fig. 20-14). Incorporation of cholesterol might have strengthened the plasma membrane without compromising fluidity and enabled early eukaryotic cells to increase in size and shed their cell walls. Having shed their cells walls, they could engulf entire prey organisms rather than relying on extracellular digestion. The increase in oxygen also precipitated most of the dissolved iron in the world’s oceans, creating ore deposits that are being mined today to extract iron.
Origins and Evolution of Chloroplasts
The acquisition of plastids, including chloroplasts, began when a cyanobacterial symbiont brought photosynthesis into a primitive algal cell that already had a mitochondrion (Fig. 2-8). The cyanobacterium provided both photosystem I and photosystem II, allowing the sunlight to provide energy to split water and to drive conversion of CO2 into organic compounds with O2 as a by-product (see Fig. 19-8). Symbiosis turned into complete interdependence when most of the genes that are required to assemble plastids moved to the nucleus of host cells that continued to rely on the plastid to capture energy from sunlight. This still-mysterious transfer of genes to the nucleus gave the host cell control over the replication of the former symbiont.
Many animals and protozoa associate with photosynthetic bacteria or algae, but the conversion of a bacterial symbiont into a plastid is believed to have been a singular event. The original photosynthetic eukaryote then diverged into three lineages: green algae, red algae, and a minor group of photosynthetic unicellular organisms called glaucophytes (Fig. 2-8). Green algae, such as the experimentally useful model organism Chlamydomonas (see Fig. 38-20), are still plentiful. Green algae also gave rise through divergence to about 300,000 species of land plants.
Evolution of Multicellular Eukaryotes
Since the origin of life on the earth, most living organisms have consisted of a single cell. Single-celled prokaryotes, protists, algae, and fungi still dominate the planet. Colonial bacteria initiated evolutionary experiments in living together over 2 billion years ago. About 1 billion years ago, the major branches of eukaryotes—fungi; cellular slime molds; red, brown, and green algae; and animals—independently evolved strategies to form multicellular organisms (Fig. 2-9).
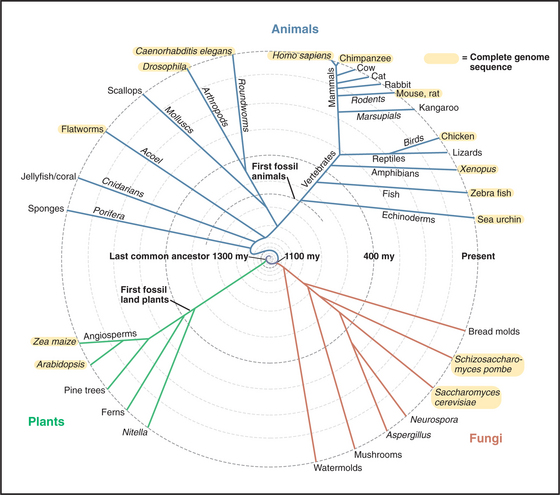
(Original drawing, based on timing for animals, adapted from Kuman S, Hedges SB: A molecular time scale for vertebrate evolution. Nature 392:917–920, 1998; based on timing for plants, adapted from Green Plant Phylogeny Research Coordination Group at http://ucjeps.herb.berkeley.edu/bryolab/greenplantpage.html; based on timing for fungi, adapted from Tree of Life Web Project at http://tolweb.org/tree.)
Algae and plants separated from the cells that gave rise to fungi and animals about 1100 million years ago. This estimate is probably correct, in spite of a general lack of fossils of these lineages older than 550 million years. Early fungi may simply be difficult to distinguish from their progenitors. Molecular phylogenetics have not yet resolved unambiguously the branching of about 5000 species of red, brown, and green algae. More recent branches, such as the evolution of plants from green algae, are better established.
Fossils of early metazoans (multicellular animals) are difficult to find because they are so tiny. The same may be true for early plants. A few well-preserved, 600-million-year-old fossils show that animals already had complex, bilaterally symmetrical bodies at this early date. These tiny (180μm long) animals had three tissue layers, a mouth, a gut, a coelomic cavity, and surface specializations that are speculated to be sensory structures. Formation of such tissues required membrane proteins for adhesion to the extracellular matrix and to other cells (see Chapter 30). Genes for adhesion proteins—including proteins related to cadherins, integrins, and Ig-CAMs—are found in species that branched before metazoans, so their origins are ancient. Other 570-million-year-old fossils are similar to contemporary animal embryos. These spectacular microscopic fossils support the hypothesis that early multicellular animals were small creatures similar to contemporary invertebrate larvae or embryos. Animals appear to have existed much earlier but have not yet been found in the fossil record.
About 600 million years ago, all other animals branched off as three subdivisions of organisms with bilateral symmetry (at some time in their lives), three tissue layers (ectoderm, mesoderm, and endoderm), and complex organs. The three subdivisions are arthropods and nematodes; mollusks, annelid worms, brachiopods, and platyhelmiths; and echinoderms and chordata (including us).
Chen J-Y, Bottjer DJ, Davidson EH, et al. Small bilaterian fossils from 40 to 55 million years before the Cambrian. Science. 2004;305:218-222.
Dawkins R. The Ancestor’s Tale. New York: Houghton Mifflin, 2004;673.
Falkowski PG, Katz ME, Knoll AH, et al. Evolution of modern eukaryotic phytoplankton. Science. 2004;305:354-360.
Gerlt JA, Babbitt PC. Divergent evolution of enzymatic function: Mechanistically diverse superfamilies and functionally distinct suprafamilies. Annu Rev Biochem. 2001;70:209-246.
Harwood A, Coates JC. A prehistory of cell adhesion. Curr Opin Cell Biol. 2004;16:470-476.
Joyce GF. Directed evolution of nucleic acid enzymes. Annu Rev Biochem. 2004;73:791-836.
Knoll AH. Life on a Young Planet: The First Three Billion Years of Life on Earth. Princeton, NJ: Princeton University Press, 2003;277.
Orgel LF. Prebiotic chemistry and the origin of the RNA world. Crit Rev Biochem Mol Biol. 2004;39:99-123.
Rivera MC, Lake JA. The ring of life provides evidence for a genome fusion origin of eukaryotes. Nature. 2004;431:152-155.
True JR, Carroll SB. Gene co-option in physiological and morphological evolution. Annu Rev Cell Dev Biol. 2002;18:53-80.
Vogel C, Bashton M, Kerrison ND, et al. Structure, function and evolution of multidomain proteins. Curr Opin Struct Biol. 2004;14:208-216.
Woese CR. A new biology for a new century. Microbiol Mol Biol Rev. 2004;68:173-186.