Chapter 3 Genetic, environmental and infectious causes of disease
CAUSES OF DISEASE
In terms of causation, diseases may be:
Features pointing to a significant genetic contribution to the cause of a disease include a high incidence in particular families or races, or an association with a known inherited feature (e.g. gender, blood groups, histocompatibility haplotypes). Environmental factors are suggested by disease associations with occupations or geography. Ultimately, however, only laboratory investigation can provide irrefutable identification of the cause of a disease. The extent to which a disease is due to genetic or environmental causes can often be deduced from some of its main features (Table 3.1).
Table 3.1 Clues to a disease being caused by either genetic or environmental factors
Disease characteristic | Genetic cause | Environmental cause |
---|---|---|
Age of onset | Usually early (often in childhood) | Any age |
Familial incidence | Common | Unusual (unless family exposed to same environmental agent) |
Remission | No (except by gene therapy) | Often (when environmental cause can be eliminated) |
Incidence | Relatively uncommon | Common |
Clustering | In families | Temporal or spatial or both |
Linkage to inherited factors | Common | Relatively rare |
Infectious agents (e.g. viruses, bacteria) are the most common and important environmental agents of disease.
Predisposing factors and precursors of disease
Many diseases are the predictable consequence of exposure to the initiating cause; host (i.e. genetic) factors make relatively little contribution to the outcome. This is particularly true of physical injury: the results of mechanical trauma and radiation injury are largely dose-related; the effect is directly proportional to the physical force.
Other diseases are the probable consequence of exposure to causative factors, but they are not absolutely inevitable. For example, infectious diseases result from exposure to potentially harmful environmental agents (e.g. bacteria, viruses), but the outcome is often influenced by various host factors such as age, nutritional status and genetic variables.
Some diseases predispose to others; for example, ulcerative colitis predisposes to carcinoma of the colon, and hepatic cirrhosis predisposes to hepatocellular carcinoma. Diseases predisposing to tumours are called pre-neoplastic conditions; lesions from which tumours can develop are called pre-neoplastic lesions. Some diseases occur most commonly in those individuals with a congenital predisposition. For example, ankylosing spondylitis, a disabling inflammatory disease of the spinal joints of unknown aetiology, is much more common in people with the HLA-B27 haplotype (Ch. 25).
Some diseases predispose to others because they have a permissive effect allowing environmental agents that are not normally pathogenic to cause disease. For example, opportunistic infections occur in those patients with impaired defence mechanisms, allowing infection by normally non-pathogenic organisms (Ch. 9).
Prenatal factors
Prenatal factors, other than genetic abnormalities, contributing to disease risk are:
Diseases due to transplacental transfer of environmental agents from the mother to the fetus include fetal alcohol syndrome, congenital malformations due to maternal rubella infection, and vaginal adenocarcinoma due to administration of diethylstilbestrol (DES). Fetal alcohol syndrome is still a serious problem, but malformations due to rubella are much less common now that immunisation is widespread. DES is no longer used during pregnancy.
The notion that disease risk in adult life could be due to fetal nutritional deprivation has gained support from the work of David Barker. The Barker hypothesis is that an adult’s risk of, for example, ischaemic heart disease and hypertension is programmed partly by nutritional deprivation in utero. This is plausible; nutritional deprivation could have profound effects during critical periods of fetal morphogenesis.
Aetiology and age of disease onset
Do not assume that all diseases manifest at birth have an inherited or genetic basis; as noted previously (Ch. 2), diseases present at birth are classified into those with a genetic basis (further subdivided into those in which the genetic abnormality is inherited and those in which the genetic abnormality is acquired during gestation) and those without a genetic basis. Conversely, although most adult diseases have an entirely environmental cause, genetic influences to disease susceptibility and vulnerability to environmental agents are being increasingly discovered.
Multifactorial aetiology of disease
Many diseases with no previously known cause are being shown to be due to an interplay of environmental factors and genetic susceptibility (Fig. 3.1). These discoveries are the rewards of detailed family studies and, in particular, application of the new techniques of molecular genetics. Diseases of adults in which there appears to be a significant genetic component include:
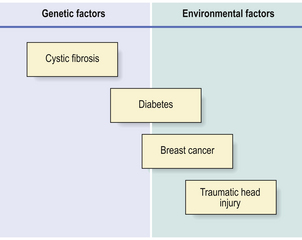
Fig. 3.1 Proportionate risk of disease due to genetic or environmental factors. Some conditions are due solely to genetic (e.g. cystic fibrosis) or environmental (e.g. traumatic head injury) factors. An increasing number of other diseases (e.g. diabetes, breast cancer) are being shown to have a genetic component to their risk, particularly in cases diagnosed at a relatively young age.
One of the reasons why there may be only slow progress in characterising the genetic component of the diseases listed above and others is that two or more genes, as well as environmental factors, may be involved. Pursuing the genetic basis of these polygenic disorders requires complex analyses.
Evidence for genetic and environmental factors
Genetic contributions to disease incidence are exposed when any putative environmental factors are either widely prevalent (most individuals are exposed) or non-existent (no known environmental agents). The epidemiologist Geoffrey Rose exemplified this by suggesting that, if every individual smoked 40 cigarettes a day, we would never discover that smoking was responsible for the high incidence of lung cancer; however, any individual (especially familial) variation in susceptibility to lung cancer would have to be attributed to genetic differences. An environmental cause, such as smoking, is easier to identify when there are significant individual variations in exposure which can be correlated with disease incidence; indeed, this enabled Doll and Hill in the 1950s to demonstrate a strong aetiological link to lung cancer risk.
Family studies
Strong evidence for the genetic cause of a disease, with little or no environmental contribution, comes from observations of its higher than expected incidence in families, particularly if they are affected by a disease that is otherwise very rare in the general population. Such diseases are said to ‘run in families’.
Having identified the abnormality in a family, it is then important to provide genetic counselling so that parents can make informed decisions about future pregnancies. The precise mode of inheritance (p. 36) will determine the proportion of family members (i.e. children) likely to be affected. Because inherited genetic disorders are either sex-linked or autosomally dominant or recessive, not all individuals in one family may be affected even if the disease has no environmental component.
Studies on twins
Observations on the incidence of disease in monozygotic (identical) twins are particularly useful in disentangling the relative influences of ‘nature and nurture’; of greatest value in this respect are identical twins who, through unfortunate family circumstances, are reared in separate environments. Uncommon diseases occurring in both twins are more likely to have a genetic component to their aetiology, especially if the twins have been brought up and lived in different environments.
Studies on migrants
The unusually high incidence of a particular disease in a country or region could be due either to the higher prevalence of a genetic predisposition in the racial or ethnic group(s) in that country or to some environmental factor such as diet or climatic conditions. Compelling evidence of the relative contributions of genetic and environmental factors in the aetiology and pathogenesis of a disease can be yielded by observations on disease incidence in migrant populations (Fig. 3.2). For example, if a racial group with a low incidence of a particular disease migrates to another country in which the disease is significantly more common, there are two possible outcomes leading to different conclusions:
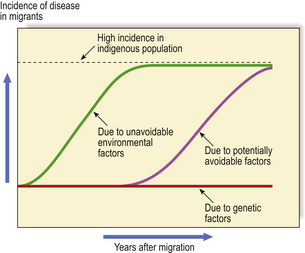
Fig. 3.2 Clues to genetic and environmental causes from disease incidence in migrants. When people with a low incidence of a disease migrate to a country in which the indigenous population has a high incidence, any change in the incidence of the disease in the migrants provides important clues to the role of genetic and environmental factors in causing the disease. A rapid rise in incidence would attribute the disease to unavoidable environmental factors such as climate or widely prevalent micro-organisms. A more gradual rise would be due to factors such as diet, over which there may be some initial cultural resistance to change. No change in disease incidence attributes the high incidence to genetic factors in the indigenous population. The distinctions are rarely as clear-cut as in this graphic example.
Most observations on disease incidence in migrant populations have been made on neoplastic disorders (cancer). This is because cancer is a major illness, likely to be reliably diagnosed by biopsy, and, in many countries, documented in cancer registries.
Association with gene polymorphisms
Within the population there are many normal genetic variations or polymorphisms. The effect of some of these polymorphisms is obvious: examples are skin, hair and eye colour, body habitus, etc. When possessed by large groups of people of common ancestry, a cluster of polymorphic variants constitutes racial characteristics. In other instances the polymorphism has no visible effects: examples are blood groups and HLA types (see below); these are evident only by laboratory testing.
The polymorphisms of greatest relevance to disease susceptibility are:
HLA types
Clinical and experimental observations on the fate of organ transplants led to the discovery of genes known as the major histocompatibility complex (MHC). In humans, the MHC genes reside on chromosome 6 and are designated HLA genes (human leukocyte antigen genes). HLA genes are expressed on cell surfaces as substances referred to as ‘antigens’, not because they normally behave as antigens in the host that bears them, but because of their involvement in graft rejection (Ch. 9). The body does not normally react to these substances, because it is immunologically tolerant of them and they are recognised as ‘self’ antigens.
HLA types are grouped into classes, principally:
Diseases may be associated with HLA types because:
Diseases associated with HLA types are listed in Table 3.2. They are all chronic inflammatory or immunological disorders. In some instances the association is so strong that HLA testing is important diagnostically: the best example is the association of HLA-B27 with ankylosing spondylitis (Ch. 25).
Table 3.2 Examples of disease associated with HLA types
Disease | HLA type(s) | Comments |
---|---|---|
Allergic disorders (e.g. eczema, asthma) | A23 | Requires environmental allergen |
Ankylosing spondylitis | B27 | Associated in c. 90% of cases |
Coeliac disease | DR3, B8 | Gluten sensitivity |
Graves’ disease (primary thyrotoxicosis) | DR3, B8 | Due to thyroid-stimulating immunoglobulin |
Hashimoto’s thyroiditis | DR5 | Aberrant HLA class II expression on thyroid epithelium |
Insulin-dependent (juvenile onset) diabetes mellitus | DR3, DR4, B8 | Immune injury to beta-cells in pancreatic islets |
Rheumatoid disease | DR4 | Autoimmune disease |
Autoimmune diseases (diseases in which the body’s immunity destroys its own cells) are most frequently associated with specific HLA types. The combination of HLA-DR3 and HLA-B8 is particularly strong in this regard, but it must be emphasised that it is present in only a minority of patients with autoimmune disease. Autoimmune diseases also illustrate a separate feature of the association between HLA types and disease. Normally, class II types are not expressed on epithelial cells. However, in organs affected by autoimmune disease, the target cells for immune destruction are often found to express class II types. This expression enables their immune recognition and facilitates their destruction.
Blood groups
Blood group expression is directly involved in the pathogenesis of a disease only rarely; the best example is haemolytic disease of the newborn due to rhesus antibodies (Ch. 23). A few diseases show a weaker and indirect association with blood groups. This association may be due to genetic linkage; the blood group determinant gene may lie close to the gene directly involved in the pathogenesis of the disease.
Cytokine genes
There is evidence linking the incidence or severity of chronic inflammatory diseases to polymorphisms within or adjacent to cytokine genes. Cytokines are important mediators and regulators of inflammatory and immunological reactions. It is logical, therefore, to explore the possibility that enhanced or abnormal expression of cytokine genes may be relevant.
Associations have been found between a tumour necrosis factor (TNF) gene polymorphism and Graves’ disease of the thyroid (Ch. 17) and systemic lupus erythematosus (Ch. 25). The TNF gene resides on chromosome 6 between the HLA classes I and II loci, linkage with which may explain an indirect association between TNF gene polymorphism and disease. There are also associations between interleukin-1 gene cluster (chromosome 2) polymorphisms and chronic inflammatory diseases. The associations seem to be stronger with disease severity than with susceptibility.
Gender and disease
Gender, like any other genetic feature of an individual, may be directly or indirectly associated with disease. An example of a direct association, other than the absurdly simple (e.g. carcinoma of the uterus and being female), is haemophilia. Haemophilia is an inherited X-linked recessive disorder of blood coagulation. It is transmitted by females to their male children. Haemophilia is rare in females because they have two X chromosomes, only one of which is likely to be defective. Males always inherit their single X chromosome from their mother; if the mother is a haemophilia carrier, half of her male children are likely to have inherited the disease.
Some diseases show a predilection for one of the sexes. For example, autoimmune diseases (e.g. rheumatoid disease, systemic lupus erythematosus) are generally more common in females than in males; the reason for this is unclear. Atheroma and its consequences (e.g. ischaemic heart disease) tend to affect males earlier than females, but after the menopause the female incidence approaches that in males. Females are more prone to osteoporosis, a common cause of bone weakening, particularly after the menopause.
In some instances the sex differences in disease incidence are due to social or behavioural factors. The higher incidence of carcinoma of the lung in males is due to the fact that they smoke more cigarettes than do women.
Racial differences
Racial differences in disease incidence may be genetically determined or attributable to behavioural or environmental factors. Racial differences may also reflect adaptational responses to the threat of disease. A good example is provided by malignant melanoma (Ch. 24). Very strong evidence implicates ultraviolet light in the causation of malignant melanoma of the skin; the highest incidence is in Caucasians living in parts of the world with high ambient levels of sunlight, such as Australia. The tumour is, however, relatively uncommon in Africa, despite its high sunlight levels, because the indigenous population has evolved with an abundance of melanin in the skin; they are classified racially as blacks and benefit from the protective effect of the melanin in the skin.
Some abnormal genes are more prevalent in certain races. For example, the cystic fibrosis gene is carried by 1 in 20 Caucasians, whereas this gene is rare in blacks and Asians. Conversely, the gene causing sickle cell anaemia is more common in blacks than in any other race. These associations may be explained by a heterozygote advantage conferring protection against an environmental pathogen (Table 3.3).
Table 3.3 Associations between disease and race
Disease | Racial association | Explanation |
---|---|---|
Cystic fibrosis | Caucasians | Hypothesised that defective gene increases resistance to intestinal infection by Salmonella bacteria |
Sickle cell anaemia (HbS gene) | Blacks | Sickle cells resist malarial parasitisation |
HbS gene more common in blacks in areas of endemic malaria | ||
Haemochromatosis | Caucasians | Mutant HFE protein may have conferred protection against European plagues caused by Yersinia bacteria |
Other diseases in different races may be due to socio-economic factors. Perinatal mortality rates are often used as an indicator of the socio-economic welfare of a population. Regrettably, the perinatal mortality rate is much higher in certain racial groups, but this outcome is due almost entirely to their social circumstances and is, therefore, theoretically capable of improvement.
Parasitic infestations are more common in tropical climates, not because the races predominantly dwelling there are more susceptible, but often because the parasites cannot complete their life-cycles without other hosts that live only in the prevailing environmental conditions.
GENETIC ABNORMALITIES IN DISEASE

Advances in genetics and molecular biology have revolutionised our understanding of the aetiology and pathogenesis of many diseases and, with the advent of gene therapy, may lead to their amelioration in affected individuals (Table 3.4).
Table 3.4 Landmarks in genetics and molecular biology
Date | Discovery |
---|---|
1940s | Genes encoded by combinations of only four nucleotides in nuclear DNA |
1950s |
1960s
1970s
1980s
1990sGene therapyEarly 21st century
Defective genes in the germline (affecting all cells) and present at birth, because of either inherited or acquired abnormalities, cause a wide variety of conditions, such as:
Most well-characterised inherited abnormalities are attributable to a single defective gene (i.e. they are monogenic). However, some inherited abnormalities or disease predispositions are determined by multiple genes at different loci; such conditions are said to be polygenic.
Genetic damage after birth, for example due to ionising radiation, is not present in the germline and causes neither obvious metabolic defects affecting the entire individual, because the defect is concealed by the invariably larger number of cells with normal metabolism, nor structural abnormalities, because morphogenesis has ceased. The main consequence of genetic damage after birth is, therefore, tumour formation (Ch. 11). There is, however, increasing evidence to suggest that cumulative damage to mitochondrial genes contributes to ageing (Ch. 12).
Gene structure and function
Nuclear DNA
Each of the 23 paired human chromosomes contains, on average, approximately 107 base (nucleotide) pairs arranged on the double helix of DNA; genes are encoded in a relatively small proportion of this DNA. To accommodate this length of DNA within the relatively small nucleus, the DNA is tightly folded. The first level of compaction involves wrapping the double helix around a series of histone proteins; the bead-like structures thus formed are nucleosomes. At the second level of compaction, the DNA strands are coiled to form a chromatin fibre and then tightly looped. During metaphase, when the duplicated chromosomes separate before forming the nuclei of two daughter cells, the DNA is even more tightly compacted.
During DNA synthesis (S phase) the bases are copied by complementary nucleotide pairing. Any copying errors are at risk of being inherited by the daughter cells and may result in disease. Copying during DNA synthesis starts in a co-ordinated way at approximately 1000 places along an average chromosome.
Nuclear genes
Genes are encoded by combinations of four nucleotides (adenine, cytosine, guanine, thymine) within DNA. Nuclear DNA is double-stranded with complementary specific bonding between nucleotides on the sense and anti-sense strands—adenine to thymine, guanine to cytosine—the anti-sense strand thereby serving as a template for synthesis of the sense strand. Most of the DNA in eukaryotic (nucleated, e.g. mammalian) cells is within nuclei; a relatively smaller amount resides in mitochondria.
The nuclear DNA in human cells is distributed between 23 pairs of chromosomes: 22 are called autosomes; 1 pair are sex chromosomes (XX in females, XY in males). Only approximately 10% of nuclear DNA encodes functional genes; the remainder comprises a large quantity of anonymous variable and repetitive sequences distributed between genes and between segments of genes. These non-coding sequences include satellite DNA which is highly repetitive, located at specific sites along the chromosomes and probably important for maintaining chromosome structure. A crucial site of repetitive non-coding DNA is the telomere at the ends of each chromosome. Its integrity is essential for chromosomal replication. In cells lacking telomerase (i.e. most somatic cells) the telomeres shorten with each mitotic division, until eventually the cells are incapable of further replication.
The segments of genes encoding for the final product are known as exons; the segments of anonymous DNA between exons are called introns (Fig. 3.3). The exons comprise sequences of codons, triplets of nucleotides each encoding for an amino acid via messenger RNA (mRNA). In addition, there are start and stop codons defining the limits of each gene. Some genes are regulated by upstream promoters. During mRNA synthesis from the DNA template, the introns are spliced out and the exons may be rearranged.
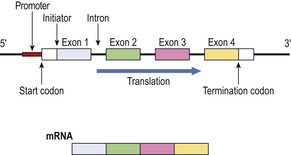
Fig. 3.3 Simplified structure of a gene and its mRNA product. Upstream of the gene is a promoter DNA sequence through which, by specific binding with regulating proteins, the translation of the gene is controlled. Start and termination codons mark the limits of the gene, bounded by untranslated sequences. The encoding portion of the gene is divided into exons, four in this example, interspersed with introns which do not appear in the mRNA product.
Gene linkage and recombination
Linkage and recombination are important processes enabling tracing of genes associated with disease. During meiosis there is exchange of chromosomal material between maternally and paternally derived chromosomes. Adjacent genes on the same chromosome are unlikely to be separated by this process and are said to show a high degree of linkage. When exchange of chromosomal material does occur, the result is called recombination. The distance between genes can be expressed in centimorgans (after a geneticist called T H Morgan); one centimorgan is the distance between two gene loci showing recombination in 1 in 100 gametes.
These processes of linkage and recombination are not only responsible for the balance between familial characteristics and individual diversity but are also important phenomena enabling defective genes to be identified, even when their precise function or sequence is unknown, by tracking the inheritance of neighbouring DNA in affected individuals and families.
Gene transcription and translation
The normal flow of biochemically encoded information is that a messenger RNA transcript is made corresponding to the nucleotide sequence of the gene encoded in the DNA (in RNA, uracil replaces thymine). The RNA transcript comprises nucleotide sequences encoding only the exons of the gene. The RNA is then translated into a sequence of amino acids specified by the code and the protein is assembled.
Under some circumstances, however, the flow of genetic information is reversed. In the presence of reverse transcriptase, an enzyme present in some RNA viruses, a DNA copy can be made from the RNA (Fig. 3.4).

Fig. 3.4 Reverse transcription of DNA from RNA. Normally, the genetic information encoded in DNA is transcribed to RNA and translated into amino acids from which the protein is synthesised. However, some RNA viruses contain reverse transcriptase, an enzyme that produces a DNA transcript of the RNA; this may then be incorporated into the genome of the cell, possibly altering permanently its behaviour and potentially leading to tumour formation (Ch. 11).
Recently, RNA-mediated interference (RNAi) has been discovered as a potentially important mechanism of target gene inhibition. This may have novel therapeutic uses.
Homeobox genes
Homeobox (HOX) genes contain a highly conserved 183 base-pair sequence. They are clustered on chromosomes as a homeotic sequence. Their expression during embryogenesis follows the order in which they are arranged, thereby sequentially directing body axis formation.
HOX genes can be subject to endocrine regulation, for example in the endometrium through the menstrual cycle and pregnancy. They can also be modulated by vitamin A (or its analogues such as retinoic acid), thus accounting for the malformations induced by excess or deficiency.
Mitochondrial genes
Most inherited disorders are carried on abnormal genes within nuclear DNA. There are, however, a small but significant number of genetic abnormalities inherited through mitochondrial DNA. Mitochondrial DNA differs from nuclear DNA in several important respects; it is characterised by:
The structure of mitochondrial DNA resembles that of bacterial DNA. Consequently, it is postulated that eukaryotic cells acquired mitochondria as a result of an evolutionary advantageous symbiotic relationship with bacteria.
Because the head of the fertilising spermatozoon consists almost entirely of its nucleus, the mitochondria of an individual are derived from the cytoplasm of the mother’s ovum. Thus, mitochondrial disorders are transmitted by females, but may be expressed in males and females.
The genes in mitochondrial DNA encode mainly for enzymes involved in oxidative phosphorylation. Therefore, defects of these enzymes resulting from abnormal mitochondrial genes tend to be associated with clinicopathological effects in tissues with high energy requirements, notably neurones and muscle cells. Examples of disorders due to inheritance of defective mitochondrial genes include familial mitochondrial encephalopathy and Kearns–Sayre syndrome.
Mitochondria and ageing
Because mitochondria play a key role in intracellular oxygen metabolism, it is hypothesised that defects of mitochondrial genes and the enzymes encoded by them could lead to the accumulation of free oxygen radical-mediated injury. Such injury could include damage to nuclear DNA, thus explaining not only the phenomenon of ageing (Ch. 12) but also the higher incidence of neoplasia in the elderly (Ch. 11).
Gene therapy
Currently, significant advances are anticipated in the specific treatment of genetic disorders (such as inherited metabolic disorders and cancer). This is the relatively new clinical science of gene therapy.
There are two approaches. First, it may be possible to replace a defective gene with a normal version in the affected tissues. This would be an ideal solution to the problem of inherited disorders such as cystic fibrosis and Duchenne muscular dystrophy. Indeed, attempts are being made to correct the respiratory tract problems in cystic fibrosis by local gene therapy applied by inhalation to the airways. Second, the function of an abnormal gene may be abrogated by administering anti-sense RNA or by RNA-mediated interference, as is being explored for Huntington’s disease.
Techniques for studying genetic disorders
Genetic disorders can be studied at various complementary levels:
At the population level, one is seeking variations in disease that cannot be explained by environmental factors; the study of migrant populations is particularly useful in disentangling the relative contributions made by genetic and environmental factors to the incidence of a disease (p. 31). In families and individuals, one is seeking evidence of the mode of inheritance—whether it is sex-linked or autosomal, whether it is dominant or recessive (Fig. 3.5); in diseases in which the abnormality is poorly characterised, studies of linkage with neighbouring genes (positional genetics) can lead to elucidation of the structure and function of defective and normal proteins. In cells, expression of the protein can be studied. It is, however, chromosomes and genes that have yielded the greatest advances in recent years.
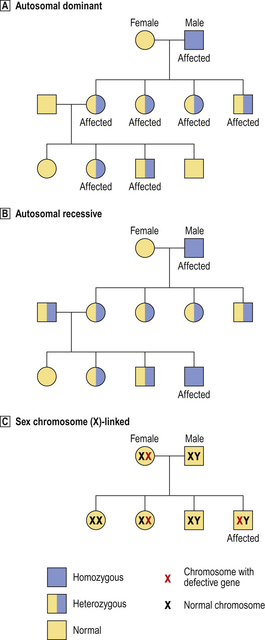
Fig. 3.5 Patterns of inheritance of abnormal genes. Autosomal dominant. Only one abnormal copy of the gene needs to be inherited for the disease to be expressed; thus, both homozygous and heterozygous individuals are affected.
Autosomal recessive. Both copies of the gene must be abnormal for the disease to be expressed; thus, homozygous individuals are affected and heterozygous individuals are asymptomatic carriers.
Sex chromosome-linked. In this example, a defective gene (e.g. for haemophilia) is located on the X chromosome. In females, the other normal X chromosome corrects the abnormality, but females can be asymptomatic carriers. In males, the disease is expressed because there is no normal X chromosome to correct the abnormality.
Modes of inheritance in families
Although some inborn errors are attributable to genetic mutations, most are inherited through parental genes. Genes located on autosomes (chromosomes other than the sex chromosomes) are autosomal; genes on the sex chromosomes are sex-linked. By studying the pattern of inheritance in an affected family (Fig. 3.5), the mode of transmission can be classified as either:
Single gene defects inherited as an autosomal dominant are almost twice as common as autosomal recessive disorders. A minority of single gene defects are sex-linked. Most inborn errors of metabolism are autosomal recessive disorders, whereas inherited disorders resulting in structural defects are autosomal dominant disorders; there are, however, exceptions to these general tendencies. A few inherited disorders are sex-linked; haemophilia (Ch. 23) is a notable example.
Homozygous and heterozygous states
The two genes at an identical place (locus) on a pair of chromosomes are known as alleles. Individuals with identical alleles at a particular locus are said to be homozygous. If the alleles are not identical, the term used is heterozygous. Dominant genes are expressed in heterozygous individuals because only one abnormal copy of the gene is required. However, by definition, recessive genes are expressed only in homozygous individuals because both copies of the gene must be abnormal. The importance of this situation is that a parent carrying only one copy of a recessive abnormal gene (who is, therefore, heterozygous for this gene) appears to be normal. If the other parent is also heterozygous for this abnormal gene, then the disease will be inherited and expressed, on average, by 25% of their children. There is a higher incidence of homologous autosomal recessive heterozygosity in related individuals and, for that reason, there is a greater risk of inherited abnormalities in the children of closely related parents (e.g. cousins). Marriage between close relatives is, therefore, prohibited by law or discouraged by tradition in many communities.
One problem in tracing genetic disorders through families is that the gene may show variable expression or penetrance. Although an abnormal gene is present, it may not necessarily always manifest itself and, when it does, the abnormality may be only slight.
Chromosomal analysis
The chromosomal constitution of a cell or individual is known as the karyotype. The 46 chromosomes in human nuclei can be seen more clearly during mitosis, especially in metaphase, when they separate. To obtain a sufficient number of cells in metaphase, colchicine can be added to the culture medium in which they are growing; this inhibits polymerisation of tubulin, preventing formation of the mitotic spindle along which the chromosomes migrate and thus blocking cell division in metaphase. The chromosomes can be:
Counting reveals disorders associated with abnormal numbers of chromosomes (e.g. trisomy in, for example, Down’s syndrome). Banding is a technique revealing, at a fairly gross level, the structure of a chromosome (Fig. 3.6). The most widely used technique is G-banding; the chromosomes are first partially digested with trypsin and then treated with Giemsa stain. This reveals alternating light and dark bands characteristic to each chromosome; the light bands comprise euchromatin (gene-rich DNA); the dark bands comprise heterochromatin (rich in repetitive sequences).
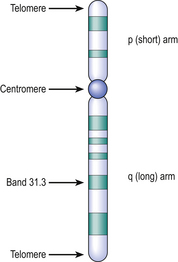
Fig. 3.6 Structure of a chromatid after banding. The centromere is a constriction at which the chromatids are joined. The short arm is designated ‘p’ (petit) and the long arm is ‘q’. The arms terminate in telomeres rich in repetitive sequences. The dark bands are numbered in order from the centromere to the tip of each arm; sub-bands are preceded by a decimal point (for example, the cystic fibrosis gene locus is on chromosome 7 and designated 7q31.3).
The size, characteristic banding, and position of the centromere enable each pair of chromosomes to be identified according to a scheme in which they are numbered from 1 to 22 (the sex chromosomes, X or Y, are not identified by number).
Probing for specific DNA sequences (either genes or repetitive sequences) can be done by incubating either chromosome spreads or interphase nuclei with complementary DNA sequences labelled with a reporter molecule such as a fluorescent dye (revealed by ultraviolet microscopy). This powerful technique enables individual genes to be mapped to chromosomes.
Molecular analysis of genetic disorders
With the techniques of molecular biology the genetic abnormality in many disorders can be identified precisely. Formerly, this identification could be done only at the level of the gene product (e.g. the defective protein); now it is possible to locate which part of which chromosome is defective and to determine the gene sequence.
The motivation to study these conditions at the genetic level of detail is twofold:
This approach is yielding important advances, but many inherited disorders are not yet completely characterised at the genetic level.
Prenatal detection can be achieved by the molecular analysis of chorionic villus biopsies in cases known to be at risk.
Functional and positional genetics
There are two possible strategies for the elucidation of the genetic abnormality in genetic diseases—functional and positional (Fig. 3.7). Which strategy is used depends on the nature of the genetic disorder and, in particular, whether the key biochemical abnormality is known.
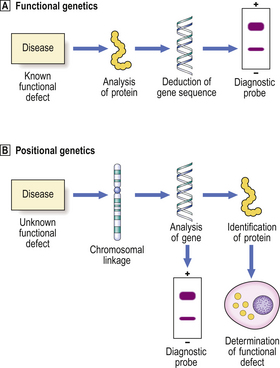
Fig. 3.7 Functional and positional genetics. Functional genetics is the strategy employed to investigate a genetic disorder in which the biochemical defect is known. This enables determination of the amino acid sequence of the abnormal protein and deduction of the DNA sequence. A complementary DNA probe can then be synthesised and used, for example in diagnostic testing for the abnormality.
Positional genetics is employed when the biochemical defect associated with the genetic disorder is unknown. However, the abnormal gene can be located by studying its linkage with neighbouring genes in affected individuals. The gene can then be analysed and the protein encoded by it deduced from the DNA sequence. Complementary DNA can be used as a diagnostic probe and the function of the defective protein can be determined.
If the biochemical abnormality resulting from the genetic defect is known, then the chromosomes or DNA from them can be probed with a complementary DNA sequence corresponding to the gene being investigated. The sequence can be deduced from the amino acid sequence of the known gene product. This is the strategy of functional genetics.
If the biochemical abnormality is not known, it can be determined by an alternative strategy of positional genetics (also called reverse genetics). ‘Positional’ in this context refers to the position of the abnormal gene in relation to well-characterised neighbouring genes with which it is linked on the same chromosome. The neighbouring genes will probably be inherited along with the defective gene, so that by studying the affected and unaffected individuals it may be possible to determine the DNA sequence of the defective gene and deduce the amino acid sequence of the gene product.
Genetic linkages
Immediately prior to meiosis leading to the production of haploid germ cells (ova and spermatozoa) from their diploid precursors, there is a random interchange of DNA segments between the homologous paternally or maternally derived chromosomes to form new, recombinant chromosomes. The process of interchange occurs over such short lengths of DNA that only those genes lying adjacent on chromosomes are likely to remain together and be inherited through successive generations. This phenomenon is useful in positional genetics only if the genes and their products are polymorphic; polymorphic genes show natural (and normal) variations in their base sequences and protein products—HLA types are good examples. This polymorphism enables the gene and its immediate neighbours to be mapped through a family and to the chromosomal level (Fig. 3.8).
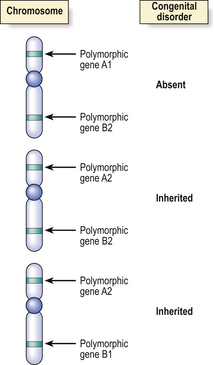
Fig. 3.8 Identification of the chromosome locus for an inherited disease by genetic linkage. Prior to meiosis there is interchange of segments of DNA between homologous chromosomes, but adjacent genes are unlikely to be separated by this process. Polymorphic (variant) DNA sequences for normal genes (e.g. for blood groups) or restriction fragment length polymorphisms in ‘anonymous’ DNA may be used as markers for the inheritance of a congenital disease, if the abnormal gene for the disease is on the same part of the same chromosome as the polymorphic marker. In this simplified example showing homologous chromosomes from three different individuals, two of whom are affected by the disease, the evidence favours the abnormal gene being very close to the polymorphic gene A2.
DNA polymorphisms
Although polymorphic genes are useful for the mapping of abnormalities, it must be remembered that most of the DNA in chromosomes is redundant or anonymous; it does not encode any genes and has no phenotypic manifestations. However, because it lacks any function, this anonymous DNA tolerates a higher frequency of polymorphic variation than the DNA in which genes are encoded. In human nuclear DNA, these random polymorphic variations occur in approximately 1 in 200 base pairs. These variations are inherited and can be used to map the inheritance of neighbouring linked genes, even though the neighbouring genes may not have been fully characterised.
Polymorphic variations arise as a result of:
Variations in anonymous DNA are detected, not by using its polymorphic products (it has none), but by determining the variations in size of the smaller DNA fragments produced by incubation with restriction enzymes. These enzymes, derived from bacteria, break DNA strands at specific points by virtue of the ability of the enzymes to recognise specific sequences of bases. By electrophoretic separation of the broken DNA strands according to their size, it is possible to detect polymorphic differences between individuals (Fig. 3.9).
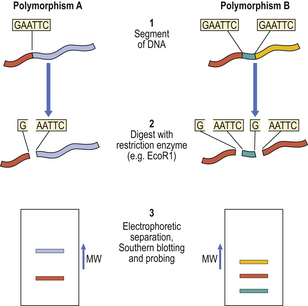
Fig. 3.9 Restriction fragment length polymorphism. Homologous regions of anonymous DNA from two individuals are shown. The polymorphic variations can be detected as follows: Step 1. The DNA is isolated. Step 2. The DNA is incubated with a restriction enzyme (EcoR1 in this example) that specifically recognises and splits DNA at sites only where there is a GAATTC base sequence. One such site exists in polymorphism A; an additional site is present in polymorphism B. Step 3. The enzymatically digested DNA fragments are loaded on to a gel and separated in an electric field according to their molecular size. After absorption on to a sheet of nitrocellulose filter paper (Southern blot), the location of the fragments of the polymorphous region can be visualised by probing with a radioactive complementary DNA strand. (MW, molecular weight.)
Some gene variants of clinical significance are single nucleotide polymorphisms (SNPs or ‘snips’) resulting from substitution of a single nucleotide. Arbitrarily, such variations must occur in at least 1% of the population to qualify as an SNP. SNPs can predispose to disease; for example, the E4 allelic variant of apolipoprotein E is associated with an increased risk of Alzheimer’s disease (Ch. 26).
Polymerase chain reaction
The polymerase chain reaction (PCR) technique is being used increasingly for the prenatal identification of genetic polymorphisms associated with congenital diseases when the precise base sequence of the polymorphic gene is known (e.g. in cystic fibrosis). The technique is especially applicable to prenatal diagnosis because it enables the abnormal gene to be amplified biochemically from only minute starting samples, even a single cell.
The PCR technique has wide applications in molecular medicine. It is a method of specifically amplifying predetermined segments of DNA from a small sample. The specificity is determined by primers, short DNA sequences complementary to the known flanking regions of the DNA segment being sought. The amplification is achieved by using a type of DNA polymerase enzyme that can withstand the cyclical heating of the reaction mixture necessary to separate the DNA strands and then cooling to permit DNA synthesis. The reaction mixture must also contain free nucleotides for incorporation into the newly synthesised DNA segments. Within a few hours the specific DNA segment, if present in the starting sample, will have been amplified about 1 million-fold. It can then be analysed in a variety of ways including determination of the full DNA sequence.
The PCR technique can be used also to study RNA, first employing reverse transcriptase to produce an amplifiable DNA transcript.
Diseases due to genetic defects
The important role of genetic abnormalities in carcinogenesis and tumour pathology is covered in Chapter 11. Here we deal with non-neoplastic disorders associated with:
Abnormal chromosome numbers
Abnormal chromosome numbers are usually obvious in karyotypic analyses and are frequently associated with grossly evident morphological abnormalities (Table 3.5). If three copies, rather than the normal pair, of a particular chromosome are present, the abnormality is referred to as trisomy. If only one of the normally paired chromosomes is present, this is monosomy. A complete triploid karyotype resulting from fertilisation of the ovum by two haploid sets of paternal chromosomes is often associated with formation of a partial hydatidiform mole (Ch. 19).
Autosomes
The commonest numerical autosomal abnormality is Down’s syndrome; the features are listed in Table 3.5. The risk of a child being affected by Down’s syndrome increases dramatically with maternal age (Fig. 3.10). In most cases, the abnormality is trisomy 21. Some of the consequences may be attributable to an increased level of gene products encoded on chromosome 21; for example, patients with Down’s syndrome develop changes in their brains similar to those seen in Alzheimer’s disease, characterised by deposition of an amyloid glycoprotein, the gene for which resides on chromosome 21 (Ch. 26).
Sex chromosomes
Numerical aberrations of sex chromosomes may be characterised by absence of one of the usual pair, as in Turner’s syndrome (X), or extra sex chromosomes, as in Klinefelter’s syndrome (XXY). These relatively uncommon conditions are usually associated with abnormalities of sexual development and, therefore, may not be obvious until puberty.
Fragile sites and chromosomal translocations
Some individuals have an inherited predisposition to chromosomal translocations (Table 3.5); that is, there is a tendency for chromosomal material to be exchanged between one chromosome and another. These translocations depend on the presence of ‘fragile sites’ at specific locations on the affected chromosomes. Translocations are often involved in the molecular pathogenesis of cancer (Ch. 11); it is, therefore, not surprising that individuals with these rare conditions associated with an increased risk of translocations have a significantly increased risk of developing tumours.
Although they are rare, study of these conditions enables a better understanding of the functional role of the genes involved in translocations and in the tumours and other abnormalities resulting from them.
Single gene defects
Single gene defects usually cause discrete biochemical or structural abnormalities. For example, most of the inherited metabolic disorders (inborn errors of metabolism) are due to single gene defects (Ch. 7).
As a rule (there are exceptions) single gene abnormali-ties resulting in structural manifestations (e.g. tumours) in adult life are inherited in a dominant manner; those resulting in biochemical abnormalities (e.g. enzyme deficiencies) in childhood are inherited in a recessive manner (Table 3.5)
Single gene defects may result from (Fig. 3.11):
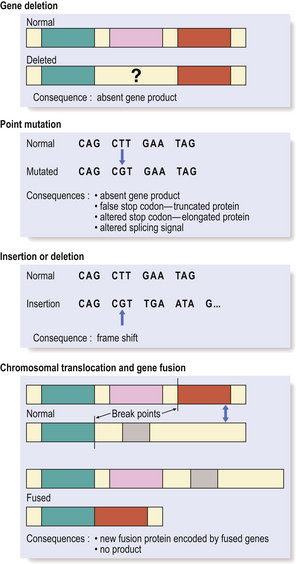
Fig. 3.11 Genetic abnormalities causing disease. The molecular consequence of a genetic abnormality depends on whether the resulting nucleotide sequence corresponds either to a codon for an alternative amino acid (mis-sense mutations) or to a premature stop or non-coding codon (non-sense mutations).
The effect of the genetic alteration may be:
X-linked single gene disorders
In addition to conditions due to abnormal numbers of sex chromosomes (Table 3.5), there are disorders due to defective genes carried on the sex chromosomes. However, because females carry two X chromosomes they only rarely develop disorders due to abnormal X chromosome genes; both X chromosomes would have to carry the same defective gene for the abnormality to appear, and that is relatively improbable. In most instances, the normal X chromosome compensates for the genetic defect on its unhealthy partner.
One of the paired X chromosomes is randomly inactivated in early embryogenesis; this is the Lyon hypothesis (after the geneticist Mary Lyon). Thus, approximately half of the cells of a female express genes on the maternally derived X chromosome, and the other cells express genes on the paternally derived partner. Females inheriting a defective gene on one X chromosome are therefore cellular mosaics: some cells are normal, others are defective.
ENVIRONMENTAL FACTORS
Most diseases are due to environmental causes, rather than to genetic abnormalities. This section deals with non-infectious environmental causes of disease.
Chemical agents causing disease

The study of environmental chemicals causing disease is toxicology. The range of possible harmful chemical agents in the environment is enormous and considerable effort is expended in their identification and safe handling. All new drugs, food additives, pesticides, etc. must be exhaustively tested for safety before they can be introduced for public use.
Mechanisms of chemical injury
The cellular mechanisms of chemical injury are described in Chapter 6.
Corrosive effects
Strong acids (e.g. sulphuric acid) and alkalis (e.g. sodium hydroxide) have a direct corrosive effect on tissues. They cause digestion or denaturation of proteins, and thus damage the structural integrity of the tissue. Powerful oxidising agents, such as hydrogen peroxide, have a similar effect.
If accidentally applied to the skin, corrosive agents cause the epidermis and underlying tissues to become necrotic and slough off, leaving an ulcer with a raw base that eventually heals by cellular regeneration.
Metabolic effects
The metabolic effects of chemicals causing disease are usually attributable to interaction with a specific metabolic pathway. However, the harmful metabolic effects of some chemicals affect many organs. Alcohol (ethanol) is a good example: it causes drowsiness and impaired judgement, liver damage, pancreatitis, cardiomyopathy, etc.
The widespread effects of some chemicals are due either to the ubiquity of a particular metabolic pathway or to the multiple effects of a single agent on different pathways.
Some chemicals are directly toxic. Others are relatively harmless until they have been converted into an active metabolite within the body.
Membrane effects
If cells had an Achilles heel, it would be the membrane that invests them. The cell membrane is not merely a bag to prevent spillage of the cytoplasm; it has numerous specific functions. In particular, it bears many receptors and channels for the selective binding and transport of natural substances. These structures are vulnerable to injurious chemicals and their damage can severely disrupt the function of the cell.
Mutagenic effects
Chemical agents or their metabolites that bind to or alter DNA can result in genetic alterations (e.g. base substitutions) called mutations. Chemicals acting in this way are classified as mutagens. Mutagens have two serious consequences:
Allergic reactions
Large molecules (e.g. peptides and proteins) may induce immune responses if the body’s immune system recognises them as foreign substances. Very small molecules are unlikely to be antigenic, but they may act as haptens; that is, they are too small to constitute antigens on their own, but do so by binding to a larger molecule such as a protein. The allergic reaction to chemicals may be mediated by antibodies or by cells, such as lymphocytes (Ch. 9), causing tissue damage.
Important chemical agents
There is insufficient space comprehensively to list all chemicals known to be harmful, but major examples are summarised here.
Smoking
Tobacco smoking is, without doubt, a major cause of illness and premature death. In 1604, it was condemned by King James I of England as ‘loathsome to the eye, hateful to the nose, harmful to the brain, dangerous to the lungs, and in the black stinking fume thereof, nearest resembling the horrible Stygian smoke of the pit that is bottomless’! Epidemiological studies during the latter half of the 20th century provide irrefutable evidence of the causal relationship between smoking and a range of neoplastic and non-neoplastic disorders including:
Paradoxically, the addictive component of tobacco smoke (nicotine) is probably the least harmful constituent. Carcinogens within the polycyclic aromatic hydrocarbon fraction of the smoke are responsible for the increased incidence of tumours of the respiratory tract and other sites in smokers. The carbon monoxide in the inhaled smoke is probably responsible for endothelial hypoxia accelerating the development of atheroma.
Alcohol
Alcohol (ethyl alcohol) in moderation appears to have beneficial effects on health. Some epidemiological studies suggest that regular consumption of one or two units per day can slightly reduce the risk of premature death from ischaemic heart disease. This apparent relationship between mortality and alcohol consumption is referred to as the J-shaped curve (the line plotted to show the relationship graphically is roughly J-shaped). However, on balance, alcohol consumption exceeding this modest allowance is probably responsible for more harm than good.
Alcohol is incriminated in the aetiology of diseases including:
Alcohol is also a factor in many road traffic accidents and in physical injury by assault.
Dusts
Not all dusts cause harm by reacting chemically with cells and body tissues. Some evoke a reaction simply because they are ‘foreign’ particles and elicit a granulomatous response (Ch. 10). Most illness resulting from dusts is due to their inhalation; this may cause:
Drugs
Many of the drugs used in therapy have a risk of adverse effects. Some of these drugs and others are also used (abused) for ‘recreational’ purposes.
Adverse effects of drugs are a major problem in modern medicine. Many of the drugs and other treatments (e.g. surgery, radiotherapy) commonly used have adverse as well as beneficial effects. The mechanism of the adverse effect varies according to the chemistry of the drug, its metabolism, and the condition of the patient (Ch. 2).
Drug abuse is a major social and medical problem in developed countries. The medical harm that results may be due directly to the abused drug or to coincidental problems. For example, intravenous drug abusers are harmed not only by the effects of the self-administered drugs but also by viruses transmitted by sharing equipment with infected addicts. Human immunodeficiency virus (HIV, causing AIDS) and hepatitis C virus (HCV, causing chronic liver disease) are particularly common.
Physical agents causing disease

Tissue damage by mechanical injury is obvious and direct. The mediation of thermal or radiation injury is more complex.
Mechanical injury
Mechanical injury to tissues is called trauma (although by common usage this word has acquired a wider meaning, e.g. ‘psychological trauma’). Cells and tissues are disrupted by trauma, causing cell and tissue loss. Depending on the tissue, regeneration may be possible. The reaction of different tissues to trauma is described in Chapter 6.
Thermal injury
The body is more tolerant of reductions in body temperature than of increases. Indeed, cooling of tissues and organs is commonly used for their short-term preservation prior to transplantation. For major cardiac surgery the body is often cooled to reduce the metabolic requirements of vital organs, such as the brain, when the circulation is temporarily arrested. Accidental hypothermia is a common medical emergency in the elderly during winter in countries such as the UK; however, recovery is usually possible unless the body temperature has fallen below 28°C.
Increased body temperature is known as pyrexia. In infections, it is usually mediated by the action of interleukins on the hypothalamus. Body temperatures above 40oC are associated with increasing mortality. Enzyme systems are severely disturbed, with severe metabolic consequences.
Local heating of the skin causes increasing local damage. Heat coagulates proteins and thereby disrupts the structure and function of cells. As the temperature rises, burns occur in the following ascending order of severity:
Thermal injury is commonly used in surgery to coagulate tissues and arrest bleeding; this is the technique of diathermy.
Radiation injury
Potentially harmful radiant energy is a source of considerable alarm because it is invisible and there is no immediate sensation of its presence.
The effects depend upon the type of radiation, the dose and the type of tissue. Cell and tissue injury from radiation is described in detail in Chapter 6.
Ionising radiation
The harmful effects of ionising radiation are:
The injury to rapidly dividing cells is immediate and becomes evident clinically within a few days or weeks (anaemia, bleeding, etc.). Fibrosis takes longer to appear—usually months or even years. Neoplasia occurs only decades after irradiation; leukaemias tend to occur earlier than solid tumours.
Despite these serious adverse effects of radiation, carefully controlled doses are used widely in medicine for diagnostic imaging of tissues (e.g. chest X-ray, radionuclide scanning) and for therapy (e.g. irradiation of tumours).
INFECTIVE AGENTS
The main classes of infective agent are:
Infective agents often demonstrate tissue specificity. Some organisms selectively infect particular organs or body systems. For example, the hepatitis viruses usually infect and harm only the liver and no other organ; they are said to be hepatotropic viruses. In contrast, Staphylococcus aureus is capable of producing injury in almost any tissue. Tissue specificity is attributable to:
The mode of transmission often reflects the tissue environmental preferences of the micro-organisms. For example, venereal infections are acquired through intimate foreplay or sexual intercourse and are caused by a relatively small group of organisms that thrive in the warm, moist micro-environment prevailing in the genital regions. Anaerobic bacteria, such as clostridia and bacteroides, have a preference for the hypoxic environment of tissue with an impaired blood supply. Infections due to agents acquired from non-human animals are called zoonoses.
Another aspect of the mode of transmission is whether it is vertical (i.e. from mother to infant) or, more commonly, horizontal (i.e. between unconnected individuals) (Fig. 3.12).
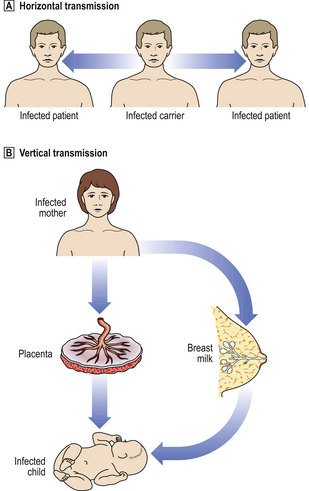
Fig. 3.12 Horizontal and vertical transmission of infections. Horizontal transmission. The micro-organism is spread between individuals through droplet infection (i.e. coughing, sneezing), venereal transmission, faecal–oral transmission, etc.
Vertical transmission. The micro-organism is spread from the mother to her child, either in utero through transplacental infection, or by contact with her body fluids (e.g. breast milk).
Bacteria
Not all bacterial infections are of immediate environmental origin; they all come from the environment but may have colonised the body harmlessly long before they cause disease in that particular individual. Soon after birth the surface of the skin, gut and vagina become colonised by a range of bacteria that are beneficial to the host; these normally present bacteria are commensals. However, if the body’s resistance is impaired, these commensal bacteria can enter the tissues, causing disease. Other bacteria causing disease are not normally present in the body.
Not all bacteria are capable of causing disease. Those that are capable are called pathogenic bacteria and their ability to do so is related to their virulence.
Bacteria usually cause disease through the production of enzymes and toxins that injure host tissues. They may also cause tissue damage indirectly by prompting a defensive reaction in excess of that justified by their innate capacity to injure. For example, most of the tissue destruction seen in tuberculosis is due to the body’s reaction to the causative bacterium rather than to any bacterial enzymes or toxins.
Bacterial lesions are often localised within a particular tissue. However, if bacteria are found within the blood, the patient is said to have bacteraemia. If the bacteria within the blood are proliferating and producing a systemic illness, then the patient is said to have septicaemia; this is a very serious condition with a high mortality.
Bacteria constitute a very large group of organisms subdivided according to their characteristics (Table 3.8) and causing a wide variety of diseases. The correct classification of a bacterium causing a clinical infection is important so that the most appropriate antibiotic can be administered without delay and so that the epidemiology of the infection can be monitored. The major classification of bacteria is according to shape—e.g. bacilli (rods) and cocci (spheres)—and staining characteristics—e.g. Gram-negative and Gram-positive; thus there are Gram-negative bacilli and cocci and there are Gram-positive bacilli and cocci. In addition, there are other major categories, such as spirochaetes and mycobacteria. Some bacteria are capable of surviving hostile conditions by forming endospores (often referred to as just spores).
Table 3.8 Examples of bacteria causing diseases
Bacterium | Classification | Diseases |
---|---|---|
Staphylococci | Gram-positive cocci | |
S. aureus |
S. epidermidis Skin commensal causing disease only in immunosuppressed hosts
S. pneumoniae (pneumococcus)Alpha-haemolyticPneumonia, otitis mediaS. viridansAlpha-haemolyticMouth commensal causing bacterial endocarditis on previously damaged valvesNeisseriaGram-negative cocci N. gonorrhoeae Venereally transmitted genital tract infectionN. meningitidis MeningitisCorynebacteriaGram-positive bacilli C. diphtheriae Pharyngitis with toxin production causing myocarditis and paralysisClostridiaAnaerobic Gram-positive bacilli C. tetani Wound infection producing an exotoxin causing muscular spasm (tetanus)C. perfringens (welchii) Gas and toxin-producing infection of ischaemic wounds (gas gangrene)C. difficile Toxin causes pseudomembranous colitisBacteroidesAnaerobic Gram-negative bacilliWound infectionsEnterobacteriaGram-negative bacilli Shigella (e.g. S. sonnei) Colitis with diarrhoeaSalmonella (e.g. S. typhi) Enteritis with diarrhoea sometimes complicated by septicaemiaParvobacteriaGram-negative bacilli Haemophilus influenzae Pneumonia, bronchitis, meningitis, otitis mediaBordetella pertussis Bronchitis (whooping cough)PseudomonasGram-negative bacilli P. aeruginosa Pneumonia, wound infections, and septicaemia in immunosuppressed hostsVibriosGram-negative bacilli V. cholerae Severe diarrhoea due to exotoxin activating cAMP (cholera)MycobacteriaAcid/alcohol-fast bacilli M. leprae Chronic inflammation, the precise character and outcome determined by the host immune response (leprosy)M. tuberculosis Chronic inflammation, the precise character and outcome determined by the host immune response (tuberculosis)SpirochaetesSpiral bacteria Treponema pallidum Venereally transmitted genital tract infection, leading to secondary and tertiary lesions in other organs (syphilis)Borrelia burgdorferi Lyme diseaseLeptospira interrogans (serotype icterohaemorrhagiae) Weil’s diseaseHelicobacterSpiral flagellate bacteria H. pylori Gastritis, peptic ulcers and gastric lymphomaCampylobacterSpiral flagellate bacteria C. jejuni Enteritis with diarrhoeaActinomycesGram-positive filamentous bacteria A. israelii Mouth commensal causing chronic inflammatory lesions of face, neck or lungsChlamydiaeObligate intracellular bacteria C. psittaci Causes psittacosis, from infected birds; pneumoniaC. trachomatis Various subtypes causing trachoma (keratoconjunctivitis), urethritis, salpingitis, Reiter’s syndrome and lymphogranuloma venereumRickettsiaeObligate intracellular bacteria Coxiella burnetii Causes Q (‘query’) fever, from infected animals; pneumonia, endocarditisMycoplasmaBacteria without cell wall M. pneumoniae Pneumonia, often described as atypical
cAMP, cyclic adenosine monophosphate
Although bacteria are widely prevalent, the prevention and therapy of bacterial infections have been great triumphs of modern medicine. Successful preventive measures have included general improvements in sanitation (drinking water, drainage, etc.) as well as the development of specific vaccines and a range of antibiotics. Coincident with the major advances in medical microbiology, immunisation and antimicrobial chemotherapy, there has been an increased incidence of troublesome endemic hospital-acquired (nosocomial) infections. The organisms causing these infections (e.g. meticillin-resistant Staphylococcus aureus) are often resistant to a wide range of antibiotics and are particularly difficult to eradicate.
The harmful effects (pathogenicity) of bacteria are mediated by (Fig. 3.13):
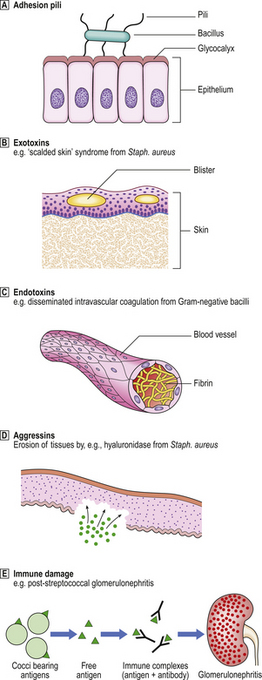
Fig. 3.13 Pathogenesis of diseases caused by bacteria. Various factors may be responsible for the local and remote effects of a bacterial infection. Not all factors are relevant to every bacterial infection. Adhesion pili.
Exotoxins.
Endotoxins.
Aggressins.
Immune damage.
Bacterial pili and adhesins
Pili, or fimbriae, are slender processes on the surface of some bacteria. They are coated with recognition molecules called adhesins. Pili and their adhesin coats serve two functions:
Adhesion pili are the means by which bacteria stick to body surfaces. These processes enable them to become fixed and thereby infect that site. Pili are a feature predominantly of Gram-negative bacteria (e.g. enterobacteria causing gastrointestinal infections, neisseriae causing meningitis and genital infections). A few Gram-positive bacteria also possess pili, notably beta-haemolytic streptococci, enabling them to adhere to the pharyngeal mucosa.
Host factors rendering some individuals more susceptible to certain types of infection include polymorphisms of the glycoproteins on cell surfaces to which the adhesin-coated pili stick. These include blood group substances.
Bacterial toxins
There are two categories of bacterial toxin:
These toxins are responsible for many of the local and remote effects of bacteria. The toxins can be neutralised by specific antibodies.
Exotoxins
These are enzymes secreted by bacteria and have local or remote effects. Their effects tend to be more specific than those of endotoxins. Examples of exotoxin-mediated effects of bacteria include:
The genes directing the synthesis of exotoxins are usually an intrinsic part of the bacterial genome. In a few instances, however, bacteria acquire the gene in the form of a plasmid, a loop of DNA that can convey genetic information from one bacterium to another; this is also a mechanism by which bacteria can acquire resistance to an antibiotic. Genes encoding for exotoxins can also be transmitted by phages: these are viruses that affect bacteria. The toxin produced by Corynebacterium diphtheriae is encoded on a gene conveyed to the bacterium by a phage; strains of this and other organisms synthesising exotoxins are known as toxigenic.
Occasionally, disease results from the ingestion of preformed exotoxin; this is the mechanism in some cases of food poisoning. A typical, but fortunately rare, example is botulism due to contamination of food with a neurotoxin from Clostridium botulinum. Toxins acting upon the gut are often referred to as enterotoxins.
Endotoxins
These are lipopolysaccharides from the cell walls of Gram-negative bacteria (e.g. Escherichia coli). They are released on death of the bacterium. The most potent is lipid A, a powerful activator of:
When these effects are severe, as in an overwhelming infection, the patient is said to suffer from endotoxic shock. The patient is feverish and hypotensive; cardiac and renal failure may ensue. Disseminated intravascular coagulation may be evinced by bruising and prolonged bleeding from venepuncture sites, as well as more serious internal manifestations. Bilateral adrenal haemorrhage, particularly associated with overwhelming meningococcal infection (Waterhouse–Friderichsen syndrome, Ch. 17), is a dramatic consequence of endotoxic shock.
Aggressins
These are bacterial enzymes with predominantly local effects, altering the tissue environment in a way that favours the growth or spread of the organism. Thus, aggressins inhibit or counteract host resistance. Examples include:
Some bacterial enzymes have brought great benefit to medicine through therapeutic uses. For example, streptokinase is used to dissolve thrombi in patients with blood vessel thrombosis.
Undesirable consequences of immune responses
Bacteria can indirectly cause tissue injury by inducing an immune response that harms the host. Fortunately, this mechanism is rare and most immune responses to bacteria are helpful to the host.
Immune responses can harm host tissues by three possible mechanisms:
Viruses
Viruses are submicroscopic infectious particles consisting of a nucleic acid core and a protein coat. They can be broadly divided into RNA and DNA viruses according to the type of nucleic acid core, but there are many further subdivisions (Table 3.9).
Table 3.9 Examples of diseases caused by viruses
Disease | Virus classification | Features |
---|---|---|
AIDS (acquired immune deficiency syndrome) | HIV (human immunodeficiency virus) (RNA retrovirus) | Infects CD4 T-helper lymphocytes causing lymph node enlargement, immune suppression and opportunistic infections |
Acute viral nasopharyngitis (common cold) | Rhinovirus (RNA) | Inflammation of nasal mucosa |
Genital herpes | Herpes simplex type 2 virus (DNA) | Sexually transmitted infection causing inflammation of genitalia |
Herpetic stomatitis | Herpes simplex type 1 virus (DNA) | Latent infection in nerve ganglia re-activated to cause vesicles in skin around mouth |
Infectious mononucleosis (glandular fever) | Epstein–Barr (EB) virus (herpes group; DNA) |
MeaslesParamyxovirus (RNA)
MumpsParamyxovirus (RNA)Fever, salivary gland inflammation and, occasionally, pancreatitis and orchitisPoliomyelitisEnterovirus (RNA)Enteric infection initially, then viraemia, from which anterior horn cells become infected, causing paralysisRabiesRhabdovirus (RNA)Acute encephalomyelitisRotavirus diarrhoeaReovirus (RNA)Fever, vomiting and diarrhoeaRubella (German measles)Togavirus (RNA)Fever, lymph node enlargement, skin rash, rhinitis; usually mildMaternal rubella associated with high risk of fetal malformationsSARS (severe acute respiratory syndrome)Coronavirus (RNA)Fever, severe respiratory infection; significant mortalitySquamous epithelial tumours (e.g. warts, carcinoma of cervix)Human papillomavirus (DNA)Transformation of cells causing their uncontrolled growthVaricella (chickenpox)Herpes group (DNA)Fever, vesicular skin rashLatent infection of dorsal nerve root ganglia; can be re-activated later causing herpes zoster (shingles)
Viruses can survive outside cells, but they always require the biochemical machinery of cells for their multiplication. Viruses show more evidence of tissue specificity than do bacteria. The ability to infect a cell type depends upon the virus binding to a substance on the cell surface; for example, human immunodeficiency virus (HIV)—the AIDS virus—selectively infects a subpopulation of T-lymphocytes expressing the CD4 (CD = cluster of differentiation) substance on their surface because viral gp120 specifically binds to it.
Some viruses circulate in the blood to reach other organs from their portal of entry; this process is called viraemia. For example, the polio virus enters the body through the gastrointestinal tract, eventually causing a viraemia to reach spinal motor neurones, resulting in their destruction and the patient’s paralysis.
The possible effects of viruses are:
The clinical manifestations of viral infections are, therefore, protean. Slow virus infections are a known or postulated cause of several neurodegenerative disorders (Ch. 26). The ability of some viruses to transform normal cells into cells capable of forming tumours is covered in Chapter 11. For many diseases where the cause is still unknown, a viral aetiology is inevitably being considered.
DNA and RNA viruses
The properties and behaviour of viruses differ according to their nucleic acid content. Unlike cells (e.g. bacteria, plant and animal cells), viruses contain either DNA or RNA, never both; the viral nucleic acid can be either single- or double-stranded.
Viruses with a DNA core are capable of surviving in the nucleus of the cell they infect, utilising the biochemical machinery there to maintain the DNA of the host cell. The DNA of some viruses can become integrated into the DNA of the host cell. These properties enable DNA virus infections to become latent, re-activated under certain circumstances, and possibly result in neoplastic transformation of the cell (Ch. 11).
RNA viruses have high mutation rates because their RNA polymerase, which copies the viral genome, is incapable of detecting and repairing replication errors. These mutations lead to changes in antigenicity, enabling RNA viruses often to evade host immunity. Some RNA viruses, called retroviruses, contain reverse transcriptase (p. 35); this enzyme produces a DNA transcript of the virus which can then become integrated in the genome of the host cell and transform its behaviour.
Tissue specificity
Unlike bacteria, viruses are incapable of replication outside cells. Therefore, a key factor in determining whether an individual becomes infected is the ability of the virus to enter the cells of the body after it has become specifically attached to their surface. There are two alternative mechanisms:
Many viruses show a high degree of tissue specificity, infecting a limited range of organs or cell types. This is known as tropism, and invariably results from the fact that the virus must bind first to a specific receptor present on a limited range of cells. Some receptors are, however, widely distributed and enable a virus to infect a wide variety of cell types.
Pathogenesis of cell injury
Viruses can produce tissue injury by a variety of mechanisms (Fig. 3.14):
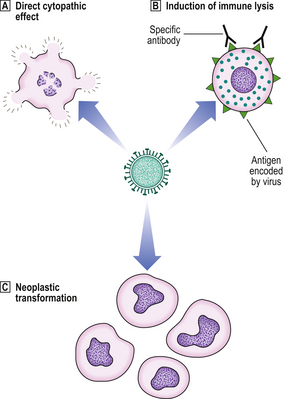
Fig. 3.14 Pathogenesis of diseases caused by viruses. Directly cytopathic viruses, injuring or killing cells infected by them.
Immune destruction of virus-infected cells. However, in the absence of an effective immune response, the cell may tolerate the virus infection.
Incorporation of viral genes into host cell genome. This incorporation may transform the cell into a neoplastic state.
Effective therapeutic remedies against many viral infections are emerging from intensive research. There are vaccines for immunisation against particularly serious or common viral infections. One of the body’s own antiviral mechanisms—interferon production—can be used in some instances. Interferons are produced by virus-infected cells and, in vitro, can be shown to interfere with or inhibit viral replication. Interferons can now be produced in large amounts and are being used in the treatment of potentially serious viral infections.
Yeasts and fungi
Yeasts and fungi constitute a relatively heterogeneous collection of micro-organisms causing disease (Table 3.10). The diseases caused by yeasts and fungi are known as mycoses.
Table 3.10 Examples of yeasts and fungi causing diseases
Organism | Classification | Disease |
---|---|---|
Aspergillus species | Fungus | Common environmental fungus causing: |
Candida albicansYeastOral and vaginal commensal causing local disease (thrush) or systemic disease (septicaemia) in immunosuppressed hosts, diabetics, and when local bacterial flora are altered by antibioticsCryptococcus neoformansFungus (yeast-like)
Histoplasma capsulatumFungus (yeast-like)
Pneumocystis jiroveciFungus (yeast-like)
Fungal infections are less common than bacterial or viral infections. However, they assume a special importance in patients with impaired immunity; in these patients, otherwise harmless fungi take advantage of the opportunity to infect a defenceless host. This situation is known as opportunistic infection and is shared by a few viruses and bacteria.
The usual tissue reaction to yeasts and fungi is inflammation, often characterised by the presence of granulomas and sometimes also eosinophils.
Mycotoxins
Mycotoxins are toxins produced by fungi. The mycotoxins of greatest medical relevance are the aflatoxins produced by Aspergillus flavus. Food stored in warm humid conditions can become infected with this fungus, thus contaminating the food with aflatoxins. Animals ingesting sufficiently high doses will develop acute hepatic damage. In humans the greatest problem is the increased risk of hepatocellular carcinoma from ingestion of relatively small doses.
Parasites
Parasites differ from other infectious agents in that they are nucleated unicellular or multicellular living organisms deriving sustenance from their hosts. Some parasites are situated on the skin (e.g. lice) and are designated ectoparasites, but most are internal residents (e.g. intestinal worms) and are referred to as endoparasites.
Parasites are the most heterogeneous group of infectious agents (Tables 3.11–3.14). Due to their requirement for particular environmental conditions and, in some instances, other hosts for their life-cycle, parasitic infections are generally more common in particular regions or countries.
Parasites are subdivided structurally into:
Parasites, particularly helminths, have complex and exotic life-cycles requiring more than one host (Fig. 3.15). Furthermore, within one host there may be successive involvement of more than one organ. Humans may be either definitive hosts or inadvertent intermediate hosts.
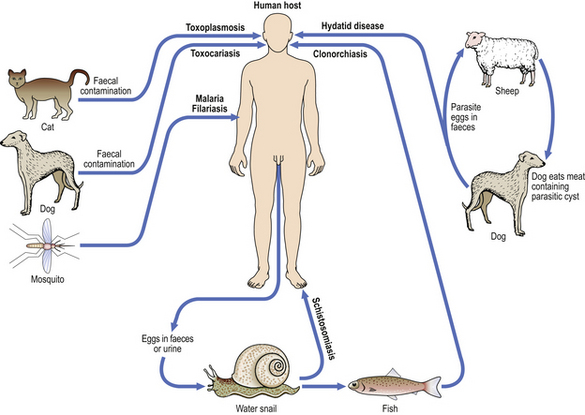
Fig. 3.15 Examples of parasite life-cycles. Simplified diagrammatic summary of the roles of hosts and vectors in the life-cycle of some parasitic diseases.
The tissue reactions to parasites are extremely variable (Fig. 3.16). If an inflammatory reaction is prompted, it is often characterised by the presence of eosinophils and granulomas. Some parasites are associated with an increased risk of tumours: Schistosoma haematobium is associated with bladder cancer, and Clonorchis sinensis is associated with bile duct cancer.
Prions
Prions (proteinaceous infective particles) are recently discovered causes of transmissible spongiform encephalopathies, the most topical of which are Creutzfeldt–Jakob disease (including the variant form) and bovine spongiform encephalopathy (‘mad cow’ disease). Susceptible individuals have an endogenous homologous protein which accumulates in excessive quantities in the brain when the exogenous prion is ingested, although other factors are involved in determining whether disease results.
Baxter P .J., Adams P.H., Aw T.-C., et al. Hunter’s diseases of the occupations, 9th edn. London: Arnold, 2000.
Connor M., Ferguson-Smith M.. Essential medical genetics. Oxford: Blackwell Science, 1997.
Cowman A.F., Crabb B .S.. Invasion of red blood cells by malaria parasites. Cell. 2006;124:755-760.
Daftary G .S., Taylor H.S.. Endocrine regulation of HOX genes. Endocrine Reviews. 2006;27:331-355.
Greenwood D., Slack R.C.B., Pentherer J.F.. Medical microbiology: a guide to microbial infections. Edinburgh: Churchill Livingstone, 2007.
Khaw K.T.. Genetics and environment: Geoffrey Rose revisited. Lancet. 1994;343:838-839.
Mims C.A., Dimmock N .J., Nash A., Stephen J.. Mims’ pathogenesis of infectious disease. London: Academic Press, 1995.
Mueller R .F., Young I .D.. Emery’s elements of medical genetics. Edinburgh: Churchill Livingstone, 2001.
Peter W., Gilles H .M.. A colour atlas of tropical medicine and parasitology, 3rd edn. London: Wolfe Medical, 1989.
Prusiner S .B ., editor. Prion biology and diseases, 2nd edn, New York: CSHL Press, 2004.
Scott J .R., Zähner D.. Pili with strong attachments: Gram-positive bacteria do it differently. Molecular Microbiology. 2006;62:320-330.
Shi Y.. Mammalian RNAi for the masses. Trends in Genetics. 2003;19:9-12.
Trent R.J.. Molecular medicine: an introductory text, 3rd edn. London: Academic Press, 2005.
Human Genome Project: http://www.sanger.ac.uk/HGP/
Online Mendelian Inheritance in Man: http://www.ncbi.nlm.nih.gov/sites/entrez?db=omim