CHAPTER 22 Endocytosis and the Endosomal Membrane System
Regulated entry of small and large molecules into eukaryotic cells occurs at the plasma membrane, the interface between the intracellular and extracellular environments. Small molecules such as amino acids, sugars, and ions traverse the plasma membrane through the action of integral membrane protein pumps (see Chapter 8), carriers (see Chapter 9), or channels (see Chapter 10), but macromolecules can enter cells only by being captured and enclosed within membrane-bound carriers that invaginate and pinch off the plasma membrane in a process known as endocytosis. Cells use endocytosis to feed themselves, to defend themselves, and to maintain homeostasis. Some toxins, viruses, pathogenic bacteria, and protozoa “hijack” this process to enter cells.
Endocytosis was discovered more than a century ago in white blood cells (macrophages and neutrophils), the body’s “professional phagocytes” (see Fig. 28-8). Endocytosis by these cells is very active, as they internalize the equivalent of their entire plasma membrane surface every hour. It was discovered that when macrophages internalize small particles of blue litmus paper, the color changes, revealing that endocytic vacuoles are acidic. Investigators still use molecules tagged with fluorescent dyes, green fluorescent protein, or electron-dense markers to follow endocytosis in living or fixed cells by light or electron microscopy. Subcellular fractionation, sometimes aided by loading cells with tracers that alter the density of the endocytic compartments or with ferromagnetic tags, has enabled the isolation and biochemical characterization of distinct classes of endocytic structures. In vitro reconstitution systems have also helped to decipher the mechanisms governing membrane trafficking along the endocytic pathway.
Cells utilize many different mechanisms for endocytosis (Fig. 22-1). These differ in mode of uptake and in the type and intracellular fate of internalized cargo. The mechanisms include phagocytosis, macropinocytosis, clathrin-mediated endocytosis, caveolae-dependent uptake, and nonclathrin/noncaveolae endocytosis. The protrusions or invaginations of the plasma membrane that are formed during these diverse endocytic processes all require coordinated interactions between a variety of protein and lipid molecules that dynamically link the plasma membrane and cortical actin cytoskeleton.
In phagocytosis and clathrin-mediated endocytosis, cell surface receptors selectively bind macromolecules (ligands) to be internalized. Ligands can be proteins, glycoproteins, or carbohydrates. In phagocytosis, the ligands are usually membrane constituents of other cells, bacteria, or viruses. After ligand-receptor complexes are concentrated into patches in the membrane, the membrane is then either pinched off to form small vesicles (in clathrin-mediated endocytosis) or zippered up around the particle to form a large vacuole inside the cell (in phagocytosis).
Phagocytosis
Phagocytosis is the ingestion of large particles such as bacteria, foreign bodies, and remnants of dead cells (Fig. 22-2). Cells use the actin cytoskeleton to push a protrusion of the plasma membrane to surround these particles.
Some cells, including macrophages, dendritic cells, and neutrophils, are specialized for phagocytosis. The presence of bacteria or protozoa in tissues attracts professional phagocytes from the blood (see Fig. 30-13), where they ingest the microorganisms and initiate inflammatory and immune responses. Other cell types use phagocytosis to remove dead neighboring cells, while amoeba use phagocytosis for feeding.
Phagocytosis proceeds through four steps: attachment, engulfment, fusion with lysosomes, and degradation (Fig. 22-3). These steps are highly regulated by cell surface receptors, phospholipids, and signaling cascades mediated by Rho-family GTPases.
Attachment
Attachment depends on the ability of the phagocytic cell to recognize the particle to be ingested. Such specific interactions trigger ingestion of the particle. Vertebrates use proteins, collectively called “opsonins,” to mark bacteria and other foreign particles for phagocytosis. Opsonins include antibodies, which bind to foreign antigens on bacteria, and complement proteins, which tag infected or dying cells. Phagocytes such as macrophages use plasma membrane receptors to bind particles coated with opsonins. For example, immunoglobulin Fc receptors bind to the constant regions of immunoglobulin G molecules (see H3 and H4 domains in Fig. 3-13B) coating pathogenic bacteria and viruses.
Engulfment
Binding of receptors such as the Fc receptor to a foreign particle generates localized signals on the cytoplasmic side of the plasma membrane. These signals trigger the assembly of the actin filaments immediately adjacent to the particle to be ingested. Growth of these actin filaments supports the plasma membrane as it zippers tightly around the particle to form a cup-like protrusion, called the phagocytic cup. The signaling pathways that give rise to these events are dependent on polyphosphatidylinositides and phosphatidylinositol (PI) kinases (Box 22-1 and Fig. 22-4). In the phagocytic cup, PI(3) kinase generates PI(3,4,5)P3 (PIP3). Effectors of this lipid include a group of PH-domain containing GEFs for the small GTPases Rac1, Arf6, and Cdc42. Once these GTPases are activated, they stimulate the cytoskeletal rearrangements of actin, leading to phagocytic cup growth. The requirement of PI(3) kinase is restricted to the stage at which the phagocytic cup seals to form a phagosome. Following this, PIP3 levels in the newly formed phagosome decline rapidly owing to the activity of PI phosphatases. The phosphatase activity leads to more PI(4,5)P2 in the phagosome membrane, which promotes assembly of actin filaments that drive the vesicle away from the plasma membrane.
BOX 22-1 Polyphosphatidylinositides in Endocytosis
Phosphatidylinositol (PI) is a glycerolphospholipid with a cyclohexanol head group (Fig. 22-4D) that can be phosphorylated on carbons 3, 4, and 5 either singly or in combination to produce polyphosphoinositides (see Fig. 26-7). Polyphosphoinositides are minor lipids in the cytoplasmic leaflet of the plasma membrane (˜1% of total lipids) and endocytic membranes, but lipid kinases and phosphatases can change polyphosphoinositide levels rapidly at local sites in membranes (Fig. 22-4E). This local synthesis of particular polyphosphoinositides regulates membrane remodeling during exocytosis, endocytosis, and vesicular trafficking by recruiting and/or activating proteins that sense the curvature of the lipid bilayer, form scaffolds on the membrane (e.g., clathrin and dynamin), or regulate actin assembly.
The most important polyphosphoinositide for endocytosis is phosphatidylinositol(4,5)bis-phosphate (PI(4,5)P2) with phosphates on carbons 4 and 5 of the head group. Two lipid kinases synthesize PI(4,5)P2 by adding phosphate first to the hydroxyl on carbon 4 and then on carbon 5 (Fig. 22-4E; also see Fig. 26-7). The second enzyme, phosphatidylinositol-4-P-5 kinase, is activated by another glycerolphospholipid, phosphatidic acid (PA; see Fig. 7-2). Since PI(4,5)P2 activates the phospholipase D (see Fig. 26-7) that makes PA, the two enzymes make a positive feedback loop that enriches PI(4,5)P2 locally in the membrane. Interactions of PI(4,5)P2 with proteins from the cytoplasm retard its mobility in the plane of the membrane, raising its local concentration until it is depleted by removal of the head group or by dephosphorylation (see Fig. 26-7).
PI(4,5)P2 participates in clathrin-mediated endocytosis, phagocytosis, and macropinocytosis (Fig. 22-4A-C). The formation of the clathrin lattice and its tethering to the plasma membrane relies on several proteins that interact with PI(4,5)P2, including AP180/CALM, epsin, and AP2 (Fig. 22-9). The GTPase dynamin, which is essential for the scission of clathrin-coated vesicles, also binds to PI(4,5)P2 (Fig. 22-10). Dephosphorylation of PI(4,5)P2 into PI(4)P is mediated by synaptojanin, which plays an important role in clathrin uncoating.
Whereas PI(4,5)P2 helps to regulate endocytosis, PI(3)P is important for early endosome dynamics. It is found on the limiting and intralumenal membranes of endosomes, where it recruits effector molecules. These include EEA1, which is responsible for endosome-endosome fusion through its interaction with Rab5, and Hrs, which recognizes ubiquitinated endocytic cargo and facilitates the formation of intralumenal endosomal vesicles through the assembly of ESCRT-I, -II, and -III. PI(3)kinase Class II or III is responsible for generating PI(3)P on membranes (Fig. 22-4E).
The plasma membrane alone was originally thought to contribute all of the membrane to make a phagocytic cup, but internal membranes are now known to contribute. Internal membranes from recycling endosomes, late endosomes, and possibly ER contribute to the phagocytic cup by fusing with the plasma membrane in a process called focal exocytosis. When secretory lysosomes fuse at the forming phagocytic cup, they release cytokines that contribute to inflammation. This couples phagocytosis to the immune response. Focal exocytosis relies on the same steps that are involved in other membrane fusion events, including transport of internal membranes along cytoskeletal tracks and their fusion by compartment-specific SNAREs under the control of Rab GTPases (see Fig. 21-12).
Fusion with Lysosomes
After closure, the actin filaments surrounding the phagosome disassemble, and motors direct the phagosome along microtubules deep into the cell during a process termed directed maturation. A series of fusion and fission reactions remove plasma membrane components and replace them with endosome-specific components including proteins (e.g., SNAREs) required for selective fusion with acidic lysosomes containing active hydrolytic enzymes. Fusion with lysosomes creates a hybrid vacuole called a phagolysosome (Fig. 22-3).
Alternative Fates of Ingested Particles
Many ingested particles are degraded in phagolysosomes to their constituent amino acids, monosaccharides and disaccharides, nucleotides, and lipids by lysosomal hydrolases. These small products of digestion are transported across the phagolysosomal membrane into the cytoplasm, where they can be reused to synthesize new macromolecules. Any undegraded material re-mains within the lysosome, which is called a residual body.
Antigen-presenting phagocytic cells, such as dendritic cells, cleave proteins of ingested microorganisms into small peptides for loading onto membrane receptors called major histocompatibility complex (MHC) class II molecules. This transfer occurs in phagolysosomes called the antigen-presenting compartment in these cells. MHC Class II molecules loaded with peptides recycle back to the surface of phagocytic cells, where they activate CD4+ T-lymphocytes (see Fig. 27-8).
Some pathogens have counterstrategies to avoid destruction by phagocytes. These include mechanisms to inhibit fusion of phagosomes with lysosomes, to resist the low pH environment of the lysosome, and to escape to the cytoplasm by lysing the phagolysosome membrane (Table 22-1). For example, in tuberculosis, macrophages in the lung phagocytose the bacterium Mycobacterium tuberculosis, but the bacterium evades destruction by secreting a phosphatase that dephosphorylates phosphatidylinositol (3P) and thus halts phagosome maturation.
“Escape” |
Secretion of toxins that disrupt phagosomal membrane (Shigella flexneri, Listeria monocytogenes, Rickettsia rickettsii) |
“Dodge” |
Entrance through alternative, pathogen-specific pathway (Salmonella typhimurium, Legionella pneumophila, Chlamydia trachomatis) |
Inhibition of phagosome-lysosome fusion (S. typhimurium, Mycobacterium tuberculosis) |
Inhibition of phagolysosome acidification (Mycobacterium species) |
“Stand and Fight” |
Low pH-dependent replication (Coxiella burnetii, S. typhimurium) |
Enhancement of DNA repair to survive oxidative stress (S. typhimurium) |
Protective pathogen-specific virulence factors (C. burnetii, S. typhimurium) |
Prevention of the processing and presentation of bacterial antigens (S. typhimurium) |
Macropinocytosis
Many cells ingest extracellular fluid in large endocytic structures called macropinosomes. Growth factors or other signals stimulate actin-driven protrusions of the plasma membrane in the form of ruffles (Fig. 22-5). These protrusions close around extracellular fluid, forming a macropinosome, which is then carried along microtubules toward the center of the cell. This allows cells to internalize fluid continuously from their surroundings without concentrating particular molecules, which is useful for bulk nutrient uptake.
Macropinosomes persist inside cells for only about 5 to 20 minutes, during which their membrane components either recycle back to the plasma membrane, potentially bypassing other organelles within the cell, or are delivered to lysosomes (Fig. 22-4). Although the membrane composition of macropinosomes resembles the plasma membrane ruffles from which they were derived, the ruffles themselves are believed to have a different composition from the rest of the plasma membrane by being enriched in both specific polyphosphoinositides and lipid raft markers. Internalization of these membranes during macropinocytosis, therefore, is likely to generate inhomogeneities in the plasma membrane that might influence cellular motility and responses to external stimuli.
Formation of macropinosomes depends on many of the same proteins that are used for phagocytosis. Phosphatidylinositol kinases and GTPases recruit and activate proteins that assemble the actin filaments supporting membrane ruffles. For example, the GTPase Arf6 activates phosphatidyl-4-phosphate-kinase, leading to production of PI(4,5)P2 at plasma membrane sites of macropinocytosis (Fig. 22-4C). PI(4,5)P2 then activates WASp-related proteins and the assembly of actin filaments. Overexpressing a constitutively active form of Arf6 increases ruffling and accumulation of macropinosomes that are enriched in PI(4,5)P2.
Endocytosis Mediated by Caveolae
Caveolae are small (-50nm), flask-shaped invaginα-tions of the plasma membrane enriched in cholesterol, diverse signaling molecules, and membrane transporters (Fig. 22-6). They are especially abundant in endothelial cells (making up more than 10% of the plasma membrane), where they mediate transcellular shuttling of serum proteins and nutrients from the bloodstream into tissues. Caveolae in other cell types are generally static, but tyrosine phosphorylation can trigger internalization of these caveolae. Simian virus 40 (SV40), for example, use caveolae to enter cells by activating a signaling cascade that cross-links surface receptors in caveolae.
Caveolae are unique microdomains of the plasma membrane that are enriched in cholesterol and stabilized by the major protein caveolin (Fig. 22-7). Caveolin inserts as a loop into the inner leaflet of the plasma membrane, where it binds tightly to cholesterol in a 1:1 ratio. Caveolin also self-associates to form a striated coat on the cytoplasmic surface of the membrane invagination. The caveolin coat is believed to stabilize the membrane and to define the size and shape of caveolae. Caveolin is immobilized in caveolae and does not diffuse laterally in the plasma membrane. This contrasts with the transient recruitment and regulated assembly of coat proteins involved in the formation of clathrin-coated pits (Fig. 22-11) and COP-coated buds (see Figs. 21-7 and 21-9). Cholesterol is also important, because depletion of cholesterol causes caveolae to flatten and caveolin to become mobile.
Association of transmembrane cargo proteins with caveolae on the cell surface involves interaction with caveolin and/or with components of the cholesterol-enriched membrane. Internalization of caveolae requires rearrangements of the actin cytoskeleton as well as the action of the GTPase dynamin (Fig. 22-8). The vesicles that form during this process are small (˜60nm in diameter) and interact transiently with endosomes or fuse with each other (forming a caveosome) while retaining their caveolae coat. In endothelial cells, where caveolae constitute a major portion of the cell surface, this permits extensive uptake of nutrients from the bloodstream. Endothelial cells in mice lacking caveolin I are unable to bind or take up serum albumin from the blood. Nonetheless, these mice are remarkably normal (except for excess cellular proliferation in some tissues and abnormal vasodilation of some blood vessels), since other pathways compensate for transport across endothelial cells.
Clathrin-Mediated Endocytosis
Clathrin-dependent endocytosis occurs at specialized patches on the plasma membrane, called coated pits, formed by a protein lattice of clathrin and adapter molecules on their cytoplasmic surface (Fig. 22-8). Eukaryotic cells use clathrin-mediated endocytosis to obtain essential nutrients, such as iron and cholesterol, and to remove activated receptors from the cell surface. Clathrin-coated vesicles also retrieve synaptic vesicle membrane at synapses following neurotransmitter release. In addition to its role in endocytosis at the plasma membrane, clathrin also participates in cargo sorting and membrane budding at other sites in cells, including endosomes and the trans-Golgi net-work (TGN).
Structure of the Clathrin Coat
Clathrin forms a three-legged structure termed a triskelion (Fig. 22-8) consisting of three 190-kD heavy chains, each associated with one of two light chains of approximately 30 kD (LCαor LCβ). This hexameric complex can self-assemble empty cages under special conditions. The assembled empty cage is like a soccer ball, with clathrin forming the ribs or seams between adjacent faces. Each rib of the cage incorporates portions of four different triskelions, which are, in turn, arranged in pentagons and hexagons (Fig. 22-9A). (See Fig. 5-4 for an explanation of how pentagons and hexagons form closed shells.) The clathrin heavy chain contains an N-terminal β-propeller domain that binds several different cargo adapters and membrane attachment proteins (Fig. 22-10). Together with clathrin, these molecules help to drive curvature of the underlying membrane and promote vesicle formation.
While clathrin cages can assemble from clathrin alone in vitro, under physiological conditions, they require assembly proteins (APs), which are the other main clathrin coat constituent. Two classes of structurally and functionally distinct APs exist: the monomeric assembly protein AP180/CALM and heterotetrameric adapter protein complexes (AP1-4). AP2 is the only heterotetrameric AP that is involved in clathrin-coated vesicle formation at the plasma membrane, with the other heterotetrameric APs involved in vesicle formation at other distinct subcellular locations. The four subunits of the AP2 complex have distinct functions: the large α-adaptin subunit recruits accessory/regulatory proteins from the cytoplasm; the large β2 subunit binds to D/ExxxLL internalization signals (e.g., dileucine-based sorting motifs) of transmembrane receptors; the medium-sized μ2 subunit binds clathrin, β-arrestins, and the YxxF (where F is any bulky hydrophobic group) internalization signals (e.g., tyrosine-based sorting motifs) of transmembrane receptors; and a small s chain stabilizes the AP complex. Multiple factors recruit AP2 to the plasma membrane. They include PI(4,5)P2, AP180/CALM, tyrosine, and dileucine-based endocytic sorting motifs in the cytoplasmic tails of receptors, synaptotagmin, and other proteins.
APs belong to a general class of endocytic proteins called adapters that include epsin, amphiphysin, Hrs/Vps27p, and β-arrestin. These proteins help to coordinate clathrin coat formation by linking it with the selection and binding of cargo and with the recruitment of other proteins involved in creating and disassembling the clathrin coat. The adapter proteins all consist of one or more folded domains connected by long, flexible linkers (Fig. 22-9C-E). This “string and knots” design allows for multiple weak-binding sites on the long flexible polypeptide, which can sweep through a large volume of cytoplasm hunting for binding partners and recapturing dissociated ligands. Fast on/off rates for binding of ligands to the string-like region, furthermore, allow multiple ligands to be held in the same locale by continual and rapid exchange between free and bound states. Cooperative networks of weak interactions between multivalent binding partners (Fig. 22-10) create a positive amplification cascade that, once initiated, drives clathrin-coat formation. The network dissociates in a self-propagating fashion once these interactions are disrupted, leading to clathrin uncoating. In this manner, adapter proteins coordinate the assembly/disassembly cycle of clathrin lattices on membranes.
Formation of Clathrin-Coated Vesicles
The formation of clathrin-coated vesicles consists of several steps (Fig. 22-11). Clathrin first binds to AP2 complexes on the cytoplasmic surface of the plasma membrane and assembles into polyhedral lattices. In this process, the α-adaptin subunit of AP2 binds clathrin, whereas the b2 subunit mediates clathrin assembly. Once polyhedral lattices have begun to form, the m2 subunit of AP2 interacts with sorting motifs on cargo molecules, resulting in the concentration of cargo molecules in the clathrin-coated region of the plasma membrane. These steps are facilitated by the ability of clathrin and AP2 to act as a binding scaffold for several other components that assist or regulate coated vesicle invagination (including Eps15, amphiphysin, and intersectin [Fig. 22-10]).
Once the coated pit becomes deeply invaginated, the neck narrows to form a constricted pit, which pinches off the plasma membrane as a clathrin-coated vesicle. The large (100-kD) GTPase dynamin coordinates the invagination, fission and internalization of clathrin-coated vesicles. In addition to a GTPase domain, dynamin has a PI(4,5)P2 binding domain, a pleckstrin-homology domain (see Fig. 25-11), a GTPase effector domain, and a proline-rich domain. PI(4,5)P2 recruits dynamin to coated pits, where it binds GTP and assembles into a helical “collar” around the necks of deeply invaginated coated pits. The proline-rich domain of dynamin binds a number of proteins with SH3 domains (see Fig. 25-11), including endophilin, cortactin, and amphiphysin. These proteins, together with dynamin, help to orchestrate coated pit invagination and budding. For example, the BAR domains of endophilin and amphiphysin (Fig. 22-9E) induce membrane curvature during coated pit constriction and coated vesicle release by dimerizing into crescent-shaped structures, which bind to highly curved, negatively charged membranes (Fig. 22-8). Epsin’s lipid-binding domain (ENTH) and extended tail serve to retain clathrin and AP2 on membranes (Fig. 22-9D). Finally, actin-binding proteins such as cortactin promote assembly of actin filaments to drive internalization, whereas intersectin regulates actin assembly by recruiting the GTPase Cdc42 and N-WASP (Fig. 22-10).
Disassembly of Clathrin Coats
Soon after a clathrin-coated vesicle pinches off the plasma membrane, the clathrin coat begins to disassemble (Fig. 22-11). This uncoating reaction recycles coat components and frees the vesicle to fuse with other vesicles to form endosomes. An important protein that is involved in this uncoating reaction is synaptojanin, a lipid phosphatase. On being recruited to clathrin-coated membranes via endophilin, synaptojanin dephosphorylates PI(4,5)P2, which weakens the attachment of coat proteins such as dynamin, Aps, and clathrin. Other proteins that are involved in clathrin uncoating include Hsc70, a member of the heat shock protein family of chaperones, and auxilin. Once the clathrin coat is removed from the vesicle, it undergoes rapid fusion with other similar vesicles or with early endosomes.
Nonclathrin/Noncaveolar Endocytosis
The mechanism of this nonclathrin/noncaveolar endocytosis pathway is not well characterized. Rather than using coat complexes to recruit cargo and to bud from the membrane, this pathway is believed to exploit heterogeneity in the lipid and protein composition of the plasma membrane to form lipid microdomains with cargo that bud into the cell (Fig. 22-7A).
The nonclathrin/noncaveolar pathway takes up proteins found in lipid rafts, detergent-resistant re-gions of the plasma membrane enriched in cholesterol, glycosphingolipids, glycosylphosphatidylinositol (GPI) anchored proteins, and some membrane proteins (Fig 22-8; also see Fig. 7-9). Thus, the pathway might circulate membrane lipids and markers for lipid rafts between the cell surface and internal membranes. As sphingolipids are important for anterograde transport through the secretory membrane system (see Fig. 21-4), the nonclathrin/noncaveolar pathway might help to resupply the secretory pathway with sphingolipids. Another function might be to help differentiate the plasma membrane of epithelial cells into polarized apical (enriched with rafts) and basolateral (deficient in rafts) domains. By contrast, most proteins that are taken up by clathrin-mediated endocytosis, such as transferrin receptors and LDL receptors, are excluded from lipid rafts and do not enter the nonclathrin/noncaveolar endocytic pathway. Conditions that lead to depletion of cholesterol inhibit nonclathrin/noncaveolar endocytosis but not clathrin-mediated endocytosis.
The Endosomal Compartment and the Endocytic Pathway
In clathrin-mediated endocytosis, four classes of endosomes are distinguished based on the kinetics with which they accumulate endocytic tracers, their morphology, localization within the cell, and the presence of specific marker proteins (Fig. 22-12). Newly internalized proteins are first delivered to so-called early endosomes, which lie near the plasma membrane and appear as an anastomosing network of tubules and vacuoles. Receptors returning to the cell surface accumulate in so-called recycling endosomes, which are tubular portions of early endosomes located in the perinuclear Golgi region of the cell. Vacuolar structures or endosome carrier vesicles detach from the early endosomes and gradually acquire internal membrane vesicles. These so-called multivesicular bodies mature into late endosomes (Fig. 22-13). Late endosomes ultimately fuse with lysosomes (discussed in Chapter 23), whose acid hydrolases degrade internalized cargo.
The Early Endosomal Compartment
Second, the geometry of the early endosome can affect protein and lipid sorting. Soluble ligands accumulate in the volume-rich vacuolar portions of the early endosome, whereas receptors accumulate in the membrane-rich tubular portions. Tubules may recycle their content directly back to the plasma membrane or later through the recycling endosome, or they may carry their content back to the TGN, depending on when they detach from the endosomal membrane. Vacuolar portions of the early endosome undergo directed maturation first into a multivesicular body and then into a late endosome before eventually fusing with lysosomes where their contents are degraded.
A third feature of early endosomes that facilitates protein sorting is the presence of V-type proton pumps (see Fig. 8-5) in the membrane. This vacuolar ATPase lowers the luminal pH progressively along the endocytic pathway from approximately pH 6.5 in early endosomes to approximately pH 5.0 in late endosomes (Fig. 22-14). The interaction of many ligands with their sorting receptors is sensitive to pH. When receptor-ligand complexes reach their threshold pH for dissociation, the ligands are released into the lumen of the endosome and are carried in the vacuolar portion of the endosome toward lysosomes, while the receptors remain membrane-bound and are returned in tubules to the plasma membrane (Fig. 22-14). The pH gradient thus facilitates membrane sorting within the endosomal system by providing spatial and temporal control over dissociation of ligand-receptor complexes and cargo degradation.
A fourth feature that influences early endosome sorting is the oligomerization or aggregation of transmembrane proteins (e.g., receptors). For example, monomeric Fc receptors recycle from endosomes to the plasma membrane, but Fc receptors cross-linked by binding antigen-antibody complexes on the cell surface are targeted from endosomes to lysosomes for degradation. Processing of internalized EGF receptors (see Fig. 27-6) is another example. The binding of EGF causes receptors to dimerize, followed by addition of a single ubiquitin (see Fig. 23-7). After internalization, EGF does not dissociate until the pH is less than 5.0, so the EGF–EGF receptor complex is targeted for degradation in lysosomes. This process, termed downregulation (see Fig. 23-2), is one of the negative feedback loops that allow cells to adapt to continuous stimulation (see Fig. 27-6).
In addition to these features, early endosomes exhibit a “mosaic” of specialized membrane domains that serves to orchestrate membrane fusion, tubulation, and invagination within this compartment (Fig. 22-15). The differentiated domains of lipids, protein-lipid, and protein-protein complexes on the early endosome are dynamically maintained. Localized production of PI(3)P by PI(3)-kinase on early endosomal membranes contributes to the formation of these domains. PI(3)P recruits the early endosome antigen 1 (EEA1) and then Rab5 and its effectors, which together serve to organize the early endosome membrane.
EEA1 is a tethering factor in the early endosomal membrane. Homodimers of EEA1 have Rab5-binding sites at both ends of a long coiled-coil and a FYVE-domain to bind PI(3)P at the one end. This allows EEA1 to tether two Rab5-positive membranes, such as two early endosomes or an endocytic vesicle and early endosome. Rab5 recruits SNARE machinery (e.g., syntaxin 13 and NSF; see Fig. 21-12) to membranes forming dynamic oligomeric complexes that promote homotypic and heterotypic fusions of early endosomes. Inhibiting PI(3)-kinase with the drug wortmannin prevents the formation of such fusion assemblies, since PI(3)P is required to recruit EEA1. The absence of PI(3)P precludes fusion among early endosomes.
The binding of Rab5 effectors to early endosomes and the presence of Rab5 exchange factors in the effector complexes establishes a positive-feedback loop that amplifies the recruitment and the activation of Rab5 GTPases in specific regions of early endosomes. This feedback allows the generation and maintenance of domains that contain Rab5-EEA1-PI(3)P in early endosomes. Other Rabs are thought to operate in a similar fashion in the endosomal system, with Rab4 and Rab11 in early/recycling endosomes and Rab7 and Rab9 in late endosomes (Fig. 22-15). Like Rab5, these other Rab proteins are thought to organize mem-brane domains with distinct functions within a single compartment.
Multivesicular Bodies and Late Endosomes
MVB formation begins on the vacuolar portions of early endosomal membranes by invagination of receptors destined for late endosomes or lysosomes (Fig. 22-16; see Fig. 23-2). Many downregulated receptors are ubiquitinated, and this modification is responsible for sorting of these receptors into newly forming MVBs through the action of HRS (hepatocyte-growth-factor-regulated tyrosine kinase substrate) and ESCRT-I, -II, and -III (endosomal sorting complexes required for transport-I, -II, and -III). When ubiquitinated receptors arrive in early endosomes, they bind HRS, which is retained at the membrane through its association with PI(3)P. Through interactions with clathrin, HRS sorts ubiquitinated receptors into clathrin-coated domains that form on new MVBs. These domains do not form clathrin-coated vesicles but instead sort ubiquitinated membrane proteins for transfer to ESCRT-1. Sequential transfer to ESCRT-II and -III on the cytoplasmic surface of the MVB drives both receptor incorporation into the membrane invaginations and invagination of the membrane itself. Wortmannin (the phosphatidylinositol 3-kinase inhibitor) inhibits the formation of intralumenal vesicles in MVBs.
Interestingly, ESCRT-1 is also involved in retrovirus budding at the plasma membrane (e.g., Ebola and human immunodeficiency virus (HIV)), a process that is topologically equivalent to membrane invagination occurring in MVBs. This occurs because HIV Gag protein highjacks the ESCRT-1 complex by mimicking the binding activity of HRS.
Late endosomes are structurally distinct from MVBs in being pleiomorphic, with cisternal, tubular, and multivesicular regions. Their protein/lipid composition is also distinct from MVBs in containing large amounts of lysosomal glycoproteins (in particular, lysosome-associated membrane proteins Lamp1 and Lamp2), which are very abundant in both late endosomes and lysosomes. In addition, late endosomes contain large amounts of lysobisphosphatidic acid (Fig. 22-15) that prefers to be in a hexagonal phase. This lipid structure can promote positive or negative bilayer curvatures that are important for invagination and intralumenal vesicle formation. Interestingly, whereas Lamps are restricted to the limiting membranes of multivesicular, late endosomal elements, lysobisphosphatidic acid is enriched in their internal membranes.
In contrast to MVBs, which function primarily as an intermediate in late endosomes/lysosome delivery, late endosomes function as an important sorting station to the TGN and back to the plasma membrane (Fig. 22-15). The elaborate structure of late endosomes serves as a final station for determining which membrane constituents in the endocytic pathway will be degraded and which will be recycled. Among the proteins that are recycled from late endosomes to the TGN and back to the plasma membrane are acid hydrolase receptors (e.g., mannose 6-phosphate receptors) and transmembrane enzymes involved in the proteolytic processing of precursor proteins (e.g., the dibasic endopeptidase furin and carboxypeptidase D). Transport from late endosomes to TGN is thought to involve budding of membrane-enclosed carriers from late endosomes followed by fusion with the TGN. Genetic and biochemical analyses of this process has revealed an important role of retromer, a complex composed of five proteins that include members of the sorting nexin family of proteins. Mutations in any of the retromer proteins prevent acid hydrolase receptors from being retrieved to the TGN and result in secretion of acid hydrolases at the plasma membrane.
Other Endocytic Compartments and Pathways
Endocytic cargo and membrane taken up by phagocytosis, macropinocytosis, caveolae, and nonclathrin/noncaveolar pathways can follow distinct itineraries from that taken up by clathrin-mediated endocytosis (Fig. 22-17). For example, cargo molecules that are taken up into phagosomes during phagocytosis or into macropinosomes during macropinocytosis do not pass through multivesicular bodies or late endosomes. Instead, they remain within the phagosome or macropinosome as these structures mature into and/or fuse with lysosomes. Caveosomes that are formed during caveolae-mediated uptake do not mature into or fuse with lysosomes but serve as a conduit for movement of molecules to other regions of the plasma membrane. Nonclathrin, noncaveolar endocytotic structures also do not mature into multivesicular bodies or lysosomes. They traffic back to the TGN to resupply the Golgi with glycosphingolipids.
Viruses and Protein Toxins as “Opportunistic Endocytic Ligands”
The threat of infectious diseases throughout the world has made research on the survival tactics of intracellular pathogens and cellular defenses against them particularly crucial. Many enveloped viruses (i.e., those with a membrane bilayer) enter cells by catching a ride on membrane proteins capable of endocytosis. Once inside an endosome, specific viral membrane proteins undergo pH-dependent conformational changes that promote their insertion into and fusion with the organelle membrane. This places the viral nucleocapsid in the cytoplasm, where it has access to the cell’s synthetic machinery, which it uses to replicate itself (Fig. 22-18).
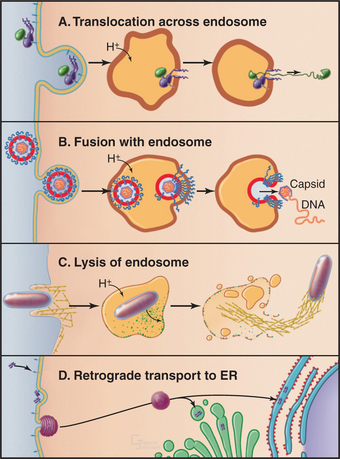
Figure 22-18 viruses and toxins have several means to gain entry into the cell. Many viruses and toxins bind to cell surface receptors that are efficiently internalized. A, Once in endosomes, pH-dependent conformational changes can trigger the translocα-tion of toxin subunits across the endosomal membrane into the cytoplasm. B, pH-dependent conformational changes can activate fusogenic viral coat proteins to mediate fusion of the viral envelope with the endosomal membrane releasing the nucleocapsid into the cytoplasm. C, Some bacteria secrete toxins after entering the endosome/phagosome; these intercalate into the membrane, creating large pores that disrupt endosomal compartments. Once in the cytoplasm, the bacterium can usurp the cell’s actin (yellow filaments) assembly machinery for propulsion. (See Fig. 37-12.) D, Some toxins enter through alternative endocytic pathways (e.g., through caveolae) and are transported in a retrograde manner back to the endoplasmic reticulum (ER), where they can utilize the cell’s translocation machinery—in reverse—to enter the cytoplasm.
Both bacteria and plants secrete protein toxins that kill animal cells efficiently by inhibiting cytoplasmic functions, such as protein translation. Some of these toxins bind to cell surface “receptors” (either integral proteins or glycolipids) via their B-chains; the toxins are endocytosed, and then the enzymatically active “A subunit” escapes into the cytoplasm (Fig. 22-18). Despite their structural similarities, various toxins enter the cytoplasm from different intracellular compartments, because their requirements for translocation differ. When pH is the trigger, toxins can be translocated directly across the endosomal membrane. Other toxins travel back to the endoplasmic reticulum and use the cell’s translocation machinery in reverse to enter the cytoplasm.
Connor SD, Schmid SL. Regulated portals of entry into the cell. Nature. 2003;422:37-44.
Finlay BB, Cossart P. Exploitation of mammalian host functions by bacterial pathogens. Science. 1997;276:718-725.
Gruenberg J, Stenmark H. The biogenesis of multivesicular endosomes. Nat Rev Mol Cell Biol. 2004;5:317-323.
Ikonen E. Roles of lipid rafts in membrane transport. Curr Opin Cell Biol. 2001;13:470-477.
Katzmann DJ, Odorizzi G, Emr SD. Receptor downregulation and multivesicular-body sorting. Nat Rev Mol Cell Biol. 2002;3:893-904.
Kirchhausen T. Clathrin. Annu Rev Biochem. 2000;69:699-727.
Lemmo SK, Traub LM. Sorting in the endosomal system in yeast and animal cells. Curr Opin Cell Biol. 2000;12:457-466.
Lindmo K, Stenmark H. Regulation of membrane traffic by phosphoinositide 3-kinases. J Cell Sci. 2005;119:605-614.
May RC, Machesky LM. Phagocytosis and the actin cytoskeleton. J Cell Sci. 2001;114:1061-1077.
McMahon HT, Gallop JL. Membrane curvature and mechanisms of dynamic cell membrane remodeling. Nature. 2005;438:590-596.
Meresse S, Steele-Mortimer O, Moreno E, et al. Controlling the maturation of pathogen-containing vacuoles: A matter of life and death. Nat Cell Biol. 1999;1:E183-E188.
Miaczynska M, Zerial M. Mosaic organization of the endocytic pathway. Exp Cell Res. 2002;272:8-14.
Mukherjee S, Maxfield FR. Role of membrane organization and membrane domains in endocytic lipid trafficking. Traffic. 2000;1:203-211.
Nichols BJ, Lippincott-Schwartz J. Endocytosis without clathrin coats. Trends Cell Biol. 2001;11:406-412.
Owen DJ, Collins BM, Evans PR. Adaptors for clathrin coats: Structure and function. Ann Rev Cell Dev Biol. 2004;20:153-191.
Pelkmans L, Helenius A. Endocytosis via caveolae. Traffic. 2002;3:311-320.
Piper RC, Luzio JP. Late endosomes: Sorting and partitioning in multivesicular bodies. Traffic. 2001;2:612-621.
Raiborg C, Rusten TR, Stenmark H. Protein sorting into multivesicular endosomes. Curr Opin Cell Biol. 2003;15:446-455.
Simonsen A, Wurmser AE, Emr SD, Stenmark H. The role of phosphoinositides in membrane transport. Curr Opin Cell Biol. 2001;13:485-492.