Chapter 11 Electrodiagnostic Evaluation of Spinal Tumors
DIAGNOSTIC UTILITY OF ELECTROPHYSIOLOGY IN SPINAL TUMORS
Spinal cord tumors account for 15% of central nervous system neoplasms.1 The initial symptoms of spinal cord tumors may be vague 2 with patients presenting with myelopathies or radiculopathies. Thus, many of these patients may first be evaluated by neurologists. Even though physical examination provides useful information with regard to localization and etiology, non-invasive tests such as somatosensory evoked potentials (SSEPs), motor evoked potentials (MEPs), electromyography (EMG), or nerve conduction studies (NCS) may provide useful anatomical or physiological information. Practically, though, magnetic resonance imaging (MRI) is the diagnostic study of choice in the assessment of myelopathy and radiculopathy.3 This chapter will review the usefulness of electrodiagnostic testing in the assessment of spinal tumors and its application as a monitoring tool in the operating room.
TYPES OF ELECTRODIAGNOSTIC TECHNIQUES
Evoked Potentials
Evoked potentials (EPs) reflect a visual representation of multiple averages of sensory or electrical signals resulting from the stimulation of the nervous system. EPs that may be useful in the electrodiagnostic evaluation of patients with spinal tumors include SSEPs and MEPs. The advantages of EPs are that they are objective, can be more sensitive than a detailed neurological exam, and can be performed in an unconscious patient. However, disadvantages of EPs are that they are not disease specific and vary based on age, height, and the presence of comorbid disease (e.g., peripheral neuropathy).4 MRI has largely replaced EPs in the diagnostic evaluation of spinal tumors.
Somatosensory Evoked Potentials
SSEPs reflect the function of the somatosensory pathways. This is accomplished through the stimulation of a peripheral nerve—typically the median nerve at the wrist and the posterior tibial nerve at the ankle—to the point of muscle twitch. Electrical potentials generated by this stimulation can then be recorded at various points along the neural pathway, such as Erb’s point after a mixed peripheral nerve stimulation in the upper extremity and in the popliteal fossa after lower extremity stimulation at the ankle. These sensory-generated electrical volleys then orthodromically enter the spinal cord through dorsal roots at several levels. Typically, an EP recorded over the posterior neck can be recorded at a latency of approximately 13 ms after upper limb stimulation and 22 ms after lower limb stimulation. These volleys ascend in the axons of the white matter dorsal column pathways where they synapse in the medulla at the nucleus cuneatus and nucleus gracilis (for the upper and lower limbs, respectively).5,6 From here these second-order neurons cross the midline and ascend as the medial lemniscus where they terminate in the thalamus. Third-order neurons project to the primary somatosensory cortex of the parietal lobe where they can be recorded. The upper limb cortical SSEP occurs at a typical latency of 20 ms and is recorded as a negative potential. The lower limb SSEP occurs at a typical latency of 37 ms and is recorded as a positive potential.
Dermatomal Somatosensory Evoked Potentials
SSEPs record the responses elicited by stimulation of mixed motor and sensory nerves that are mediated by several cervical or lumbosacral nerve roots. Thus, they may be normal despite nerve root compromise, given that their abnormal function may be masked by the normal function of the other nerve roots comprising the nerve.7
To test the function of an individual sensory nerve root, dermatomal somatosensory evoked potentials (DSSEPs) can be recorded. The sensory dermatomes resulting from dorsal (sensory) nerve root innervation of the skin can be recorded by direct dermatomal electrical stimulation of the skin surface with surface electrodes and recorded as DSSEPs.7 Surface electrodes are placed over the dermatomal areas to be monitored and electrically stimulated, thus generating DSSEPs from that particular dermatome. The stimulation and recording parameters used are the same as those for SSEPs.
Practically, though, the reliability of DSSEPs in the diagnostic evaluation of patients with suspected radiculopathies is questionable.8,9 A report of the American Academy of Neurology’s Therapeutics and Technology Assessments Subcommittee recommended that the currently available evidence is insufficient to determine the appropriateness of DSSEPs. As a result, they should be regarded as investigational only.10
Transcranial Magnetic Stimulation
MEPs can be used to evaluate the functional integrity of the corticospinal tract system from the brain to the spinal cord or muscle. This can be accomplished by either magnetic or electric stimulation of the brain. However, in the awake patient only transcranial magnetic stimulation (TMS) is done because the transcranial electric motor evoked potentials (TcEMEPs) induce a current in the brain through a large stimulus that would activate pain fibers within the scalp and thus would not be tolerated by an awake patient.6
Magnetic activation of the corticospinal pathway can be accomplished by the placement of a magnetic coil that is held close to the patient’s head. This induces high-intensity current pulses within the patient’s brain, which stimulates the neurons of the cerebral cortex. This stimulation produces multiple D (direct) waves that are the result of multiple action potentials within the descending pyramidal tract neurons. After this initial D wave are I (indirect) waves, which are the result of synaptic activation of further pyramidal neurons via interneurons. Because the currents produced by magnets are predominantly tangential, I waves are the predominant WAVEFORM produced.6 Because TMS produces predominantly I waves, which are the result of synaptic activity within the cortex interneurons, it cannot be used in the anesthetized patient. Because anesthetics predominantly take effect at synapses, the I waves would be lost and, hence, there would be no recordable MEPs under general anesthesia.
These I waves must then synaptically transmit their impulses to the alpha motor neurons in the spinal cord, then to the peripheral motor nerve, and finally across the neuromuscular junction to muscle where compound muscle action potentials (CMAPs) can be recorded. Firing of the alpha motor neuron requires the temporal summation of multiple excitatory postsynaptic potentials. This can be accomplished by the multiple I waves elicited by a single transcranial magnetic stimulus.6
Because these I waves depend on cortical synaptic function, they can be lost with cortical lesions.6 However, for the evaluation of motor function resulting from lesions below the cortex (e.g., the descending axons in the spinal cord), this technique may still be useful when the spinal cord is the region to be assessed.
NERVE CONDUCTION STUDIES AND ELECTROMYOGRAPHY
Nerve Conduction Studies
NCS involve the electrical activation of nerves to provide functional assessment about the peripheral nervous system in the form of conduction and axonal integrity. They may provide diagnostic, descriptive, or prognostic information and are accomplished through the application of a depolarizing square wave electrical pulse over a peripheral nerve, which results in activation of both the sensory and motor axons of the mixed nerve. A nerve action potential is propagated, which can be recorded at a particular distance over the nerve, as well as a CMAP, which can be recorded over the target muscle. Components such as conduction velocity, amplitude, and shape are assessed. This is typically done with surface electrodes. Because peripheral nerves contain fibers of differing diameters, degrees of myelination, and amount of sensory or motor connections, NCS are designed to assess the fastest 20% of these fibers.11
H Waves
The H wave is the electrical representation of a monosynaptic spinal reflex. It assesses the S1 nerve root, as it is only consistently obtained from recording the gastrocnemius-soleus muscles after stimulation of the tibial nerve in the popliteal fossa. It is the EMG equivalent of the ankle deep tendon reflex. The amplitude can be compared with the contralateral side. It is beneficial in that it assesses the function of the sensory root fibers, including the segment proximal to the dorsal root ganglion (DRG). However, it can sometimes be normal in S1 radiculopathies, and its abnormality cannot always be attributed to the radiculopathy. It also can be abnormal in polyneuropathy cases and in patients older than 60 years.12
F Waves
F waves are a type of late motor response that may be able to be used to evaluate the motor nerve root. F waves are recorded from muscle after maximal stimulation of its nerve. When a motor nerve axon is stimulated, the action potential propagates in both directions so that an orthodromic potential can be directly recorded in muscle as a CMAP and an antidromic potential conducts proximally to the anterior horn cell. About 2% of axons then backfire, leading to a small additional orthodromic potential that can be recorded in muscle after the CMAP as an F wave. However, because a different population of anterior horn cells backfires with each stimulus, the F waves are variable in size, shape, and latency and are usually less than 5% of the CMAP amplitude.11,12
F waves initially were thought to be able to assess proximal nerve motor root segments inaccessible by conventional NCS. However, they have been found to be insensitive to this. The nerves stimulated contain innervation from usually more than one root, and they are mediated along a pathway that extends muscle, nerve, root, plexus, and spinal cord; thus, an abnormality anywhere along this pathway could lead to an abnormal F wave.12 When abnormal, they often offer little extra information, except in specific situations such as Guillain-Barré syndrome.
There is an exception in which both H waves and F waves may be clinically useful. In the case of an acute myelopathy, both F waves and H waves may disappear during the acute phase of spinal shock only to reappear thereafter.13
Needle Electromyography
EMG records the electrical activity in muscles. This is done by the insertion of a monopolar or concentric needle into a muscle and recording the observed and corresponding auditory response of spontaneous and recruited activity. It is used to differentiate myopathic from neurogenic disease and, by determining the distribution of neurogenic abnormalities, can localize the site of a lesion, such as differentiating nerve from plexus from radicular lesions.14,15
APPLICATION TO TUMORS
Myelopathy
Somatosensory Evoked Potentials
SSEPs can be used in the clinical diagnosis of neurological disease, in particular for multiple sclerosis.5 They may reveal dysfunction in the dorsal column/medial lemniscal thalamocortical sensory pathway or reveal subclinical involvement, help to define the anatomical involvement of a lesion, or be used to monitor a patient’s status over time.4 Even though SSEPs have been shown to be sensitive in detecting abnormalities in patients with intraspinal neoplasms, this technique is not as sensitive in detecting these tumors as MRI is.1
Motor Evoked Potentials
TMS can be used to measure central motor conduction time. Pyramidal tract dysfunction will result in slowing of central motor conduction time. MEPs are most often used in the assessment of multiple sclerosis or amyotrophic lateral sclerosis, conditions in which evidence of corticospinal tract involvement that may not be clinically evident is sought.14
For spinal cord lesions for which the predominant injury is demyelination leading to slowing of central motor conduction time, TMS can be useful in assessing the level of functional impairment. Through appropriate muscle sampling, the level of the lesion can be localized to a particular root.14
Radiculopathy
Dermatomal Somatosensory Evoked Potentials and Electromyography
Radiculopathies are usually caused by root compression. They are the most common cause of referral to the EMG laboratory.12 EMG has been shown to be of great utility and has been used in the evaluation of radiculopathies for more than 50 years.12
There are 31 pairs of spinal nerves attached to the spinal cord by dorsal (sensory) and ventral (motor) roots. The ventral roots originate from cells in the anterior and lateral gray columns of the cord; the dorsal roots originate from the DRG, which lie distal to the cord. Thus, sensory NCS, which do not assess the sensory roots proximal to the DRG, remain unchanged in radiculopathies. The dorsal and ventral roots join to form spinal nerves.12
The region of skin with sensory innervation from a single dorsal root constitutes a dermatome. The muscles that share innervation from a single ventral root constitute a myotome. Most muscles are made up of more than one myotome. Sensory fiber compromise alone is the most common clinical presentation of radiculopathies. Isolated motor dysfunction is the least common.12
However, in most patients with signs and symptoms of radiculopathy, the most efficient diagnostic test is MRI. Even though electrodiagnostic studies cannot provide an etiology, localization of the involved myotome can be detected by EMG.16
Although there are reports of DSSEPs being sensitive to changes in nerve root function,17 their value in diagnosing radiculopathy is questionable.8 For the detection of radiculopathies, EMG is more sensitive than DSSEPs.12 In the case of radiculopathies caused by spinal neoplasms, EMG may show early signs of denervation in the paraspinal musculature.18,19
Conus Medullaris
The conus medullaris comprises the S2–5 nerve roots. Intrinsic spinal cord tumors that invade the conus typically do so caudally, beginning with the S5 root. This leads to the usual presenting symptoms of dull pain with paresthesias in the perianal and genital regions followed by sphincter disturbance or impotence. As the lesion spreads rostrally, the ankle jerks may be lost. If the symptoms are initially unilateral, they will quickly spread to involve the contralateral side.20
EMG evaluation of conus medullaris lesions will often indicate bilateral and multiple radiculopathies. Anal sphincter EMG may show evidence of denervation. NCS may reveal abnormal motor potentials, but sensory nerve action potentials should be normal because the lesion is preganglionic.20
SSEPs may reveal abnormalities after lower extremity stimulation because the ascending dorsal column pathways are affected by the lesion. Similarly, TMS may be abnormal if the descending corticospinal tract is affected. However, these findings, too, would be limited to the lower extremities.20
Cauda Equina
Extramedullary spinal cord tumors below the level of the spinal cord can lead to a cauda equina syndrome. Depending on the affected nerve roots, the patient will typically present with pain, paresthesia, weakness, or diminished reflexes in an asymmetric radicular distribution. If the lesion compresses the cord, then upper motor neuron signs may appear. These lesions resemble conus medullaris lesions except that the distribution is more asymmetric, the pain is often more severe, and the sacral and lumbar roots will be affected.20
EMG is helpful in showing evidence of denervation involving the lumbar and sacral levels in an asymmetric radicular distribution. The paraspinal muscles and sphincter muscles also may be affected. NCS may reveal an asymmetric, side-to-side difference in amplitude of CMAPs. Sensory conduction studies will again be normal.20
INTRAOPERATIVE NEUROPHYSIOLOGICAL MONITORING OF SPINAL TUMORS
Why Use Monitoring?
The use of continuous intraoperative SSEP monitoring in one study was found to reduce the incidence of neurological injury in cervical surgery from 3.7% to 0%. This was likely a result, in part, of the early detection of vascular or mechanical compromise of the spinal cord or nerve roots and the subsequent rapid response in anesthesia or surgical management.21
The risk of postoperative paraplegia or paraparesis after scoliosis surgery is 0.72%.22 Nuwer et al23 performed a large multicenter survey in 1995 to compare SSEP changes to neurological function after scoliosis surgery. Out of 51,263 surgeries, they found a false negative rate of 0.063% and a false positive rate of 1.51%. However, their survey also included cases in which intervention by the surgeon may have occurred based on the changes in the SSEPs. There was a true negative rate of 97.4% and a true positive rate of 0.423%. Based on these findings, the sensitivity of SSEPs in scoliosis surgery was calculated to be 92%, the specificity 98.9%, the negative predictive value 99.33%, and the positive predictive value only 42%. Based on these findings, Nuwer et al concluded that SSEPs detected more than 90% of potential deficits. The factors that appeared to most affect the rate of new neurological deficits included the experience of the surgeon and the surgeon’s and technologist’s experience with SSEP monitoring.23
Intraoperative neurophysiological monitoring (IOM) can be used to monitor intramedullary1,2 and extramedullary,24 primary, or metastatic tumors.25 Intramedullary tumors are rare, and the majority are histologically benign.2 Thus, their radical removal will improve long-term survival with an acceptable morbidity.26 IOM techniques can aid in the degree of aggressiveness of tumor resection.
Occasional false positive cases have been reported in which there is a significant change in the EPs but no new postoperative neurological deficit. These cases could be the result of prompt surgical intervention or the sensitive detection of a subtle clinical change by the monitoring procedure. False negative cases also have been reported in the absence of EP changes. These possibly could be a result of delayed onset in symptomatology from effects such as latent vascular change or compression exacerbated by patient movement.27
Monitoring Techniques
Somatosensory Evoked Potentials
Electrophysiological monitoring of the spinal cord during surgery, which may carry a risk of spinal cord compromise, has been described for more than 30 years.28 SSEPs initially were used to provide warning of impending spinal cord compromise and to supplement the wake-up test to assess spinal cord function. SSEPs continue to be the most widely used monitoring modality.7 In particular, SSEP monitoring alone has been shown to improve neurological outcome after spinal surgery.23
Technical Aspects
Stimulation
SSEPs monitor the functional status of the somatosensory pathways.29 Stimulating electrodes are placed over the median or ulnar nerve at the wrist for upper extremity SSEPs and posterior tibial nerve at the ankle or peroneal nerve at the knee for lower extremity SSEPs. For median nerve stimulation, which encompasses the nerve roots originating from levels C6–T1, the anode is placed at the palmar crease and the cathode is placed between the palmaris longus and flexor carpi radialis tendons, 2–3 cm proximal to the anode to avoid “anodal block.”7 To assess the lower cervical spinal roots (C8–T1), the ulnar nerve is preferentially stimulated with the anode at the wrist crease on either side of the flexor carpi ulnaris tendon, 2–3 cm distal to the cathode. The superficial radial nerve at the wrist and the ulnar nerve at the elbow are other potential sites of stimulation.7 For posterior tibial nerve stimulation, the cathode is placed between the medial malleolus and the Achilles tendon, 2–3 cm proximal to the anode. For peroneal SSEPs, the common peroneal nerve is stimulated in the popliteal fossa, medial to the biceps femoris tendon with the cathode below the crease, 2–3 cm proximal to the anode. Posterior tibial nerves are the preferred SSEPs of the lower extremity because they are larger, less variable, produce smaller muscle contractions, are more easily accessible, and provide a peripheral response at the knee.
Contact impedance is limited to 5 kOhms or less. To reduce stimulus artifact, a ground electrode is placed on the stimulated limb. Either a constant voltage or a constant current stimulator is used. Constant current stimulators can compensate for changes in contact resistance; however, this is limited by the maximum output voltage of the stimulator. Alternatively, constant voltage stimulators will provide a constant stimulus intensity only if the contact resistance remains constant. Thus, constant current simulators are recommended. Monophasic rectangular pulse waves with a typical pulse duration of 100–300 μsec at a rate between 2–5 Hz should be given. Stimulus rates that are exact subharmonics of line frequency (60 Hz in North America) should be avoided (e.g., 6 Hz). Lower extremity stimulation rates between 1.5–3 Hz may improve the responses. Increasing the stimulus rate to greater than 5 Hz for the lower extremities and greater than 9 Hz for the upper extremities often degrades the cortical responses. Supramaximal stimulus intensity to more than the motor threshold should be used, though usually it should not be necessary to exceed 50 mA.5,7
Recording
Either needle electrodes or standard electroencephalographic disc electrodes are applied with either paste or collodion. If disc electrodes are used, collodion is preferred in the operating room setting because of its durability. Impedances are kept at less than 5 kOhms. A system bandpass with low frequency filters of 30 Hz and high frequency filters of 3 kHz are used.5 These filters optimize noise rejection while retaining the SSEP characteristics.27 The number of average sweeps required is usually between 300–500. However, this number depends on the signal-to-noise ratio and the time required to obtain a response. For example, at a stimulus rate of 4.7 Hz, it would take about 1 minute to acquire 300 sweeps. During temporary vessel occlusion, this amount of time may need to be reduced, thus acquiring fewer samples. However, to ensure that the responses are real and not artifact, verifying by repetition or odd and even averaging can be done.7
The time bases to acquire and display the SSEPs are usually set at approximately 50 ms for the upper extremities and 100 ms for the lower extremities. These values are based on the typical latencies for the peaks of interest (i.e., approximately 20 ms for the upper extremity and approximately 40 ms for the lower extremity). Factors such as age and size of the patient also need to be taken into account.7
Electrodes are placed based on the International 10–20 System. Ci and Cc correspond to C3 or C4, ipsilateral and contralateral to the stimulus. CPi and CPc (or C3′ and C4′) are midway between C3 or C4 and P3 or P4 (or approximately 2 cm posterior to C3 and C4). Recording spinal electrodes are placed in the midline and are labeled by the level they are placed followed by the letter ‘S’ (e.g., a recording cervical spinal electrode over the C7 vertebra is labeled as C7S). Ep refers to recordings over Erb’s point (i.e., the angle between the clavicle and the clavicular head of the sternocleidomastoid muscle). Pf refers to electrodes in the popliteal fossa (Fig. 11-1).5
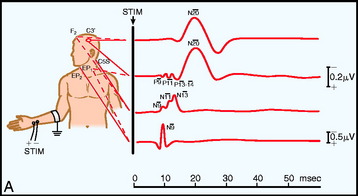
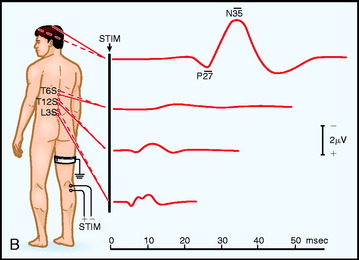
Fig. 11-1 Electrode placement for upper limb (A) and lower limb (B) SSEPs.30
From Spehlman R. Evoked Potential Primer: Visual, Auditory, and Somatosensory Evoked Potentials in Clinical Practice. Boston, Butterworth, 1985, with permission.
The primary purpose of the Ep and Pf potentials is to verify that the peripheral stimulus is adequate to elicit SSEPs. Thus, technical problems, such as a malfunctioning stimulator or a displaced electrode, can be diagnosed.7 The cervical spine recording is important for spinal cord surgery below the level of the cervical spinal cord because this potential will be relatively unaffected by anesthetic drugs. However, if the cervical spine is within the surgical site, this response can be recorded from one or linked earlobes. These responses have several generators, and as a result several peaks. The N13 peak has generators below the medulla and at the cervicomedullary junction. The P14 peak has generators only above the spine; it is best recorded using the CZ linked ears reference. The cortical signals can serve as a backup during spinal surgery; however, it must be emphasized that they are susceptible to anesthetic drugs typically used during surgery (Table 11-1).
Terminology
N (negative) and P (positive) is the nomenclature used to designate polarity of the potentials. The potentials may be labeled based on their latency (e.g., N20 to refer to the near-field cortical somatosensory potential after median nerve stimulation).5 They may alternatively be given numerical labels based on their order of occurrence (e.g., N1 to refer to the first negative deflection, P2 to refer to the second positive deflection, and so on).
Interpretation
Criteria for intervention include a reproduced change after other technical, anesthetic, and physiological factors have been eliminated. The parameters that are considered critical include an increase in latency of 10% or a decrease of 50% in the peak-to-peak amplitude of the primary SSEP.7,27
Dermatomal Somatosensory Evoked Potentials
DSSEPs record the responses elicited by stimulation of mixed motor and sensory nerves, which are mediated by several cervical or lumbosacral nerve roots. Thus, they may be normal despite nerve root compromise given that their abnormal function may be masked by the normal function of the other nerve roots comprising the nerve. To test the function of an individual sensory nerve root, DSSEPs can be recorded.7
The sensory dermatomes resulting from dorsal (sensory) nerve root innervation of the skin can be recorded by direct dermatomal stimulation of the skin surface with surface electrodes and recorded as DSSEPs. Surface electrodes are placed over the dermatomal areas to be monitored. The stimulation and recording parameters used are the same as those for SSEPs. They are unaffected by muscle relaxants but are sensitive to anesthetic drugs. Changes in the cortical responses are sensitive to mechanical trauma or compression of nerve roots.7
The practical use of DSSEPs, however, is questionable. Even though this technique may be useful for cauda equina tumor removal, it is still uncertain as to whether it is sensitive to nerve root decompression.17 Additionally, DSSEPs are slow and technically demanding to produce and may not be reproduced reliably. They also have been shown to be poorly predictive of clinical outcome.9 As a result, their usefulness in the operating room setting may be limited.
Transcranial Electric Motor Evoked Potentials
SSEP monitoring during intramedullary spinal cord tumor surgery may predict poor postoperative motor function.31 However, SSEPs monitor only the dorsal column somatosensory pathways; thus neurological deficits may still occur in the absence of SSEP changes.32
The vascular supply to the motor and sensory tracts in the spinal cord is separate. The dorsal columns are predominantly supplied by the posterior spinal arteries, whereas the corticospinal tracts in the anterolateral funiculi are supplied by the anterior spinal artery.6 Impairment of the blood supply to the anterior spinal artery could then render the patient paraplegic without affecting the SSEPs.33 Thus, separate monitoring of the motor tracts is necessary.
It is not surprising that MEPs are superior in identifying motor tract injury as compared with SSEPs.34 Motor tracts of the spinal cord can be directly monitored using TcEMEP. This can be accomplished by a high-intensity stimulus applied to the scalp to overcome the high electrical resistance of the skull, resulting in only a small fraction of the current reaching the intracranial compartment. As a result of this stimulus, pain fibers in the scalp are activated.6 Thus, this procedure is limited to the anesthetized patient.
Muscle motor EPs recorded as CMAPs have been shown to be highly sensitive and specific (100% and 91%, respectively) in predicting postoperative motor deficits (Fig. 11-2).35
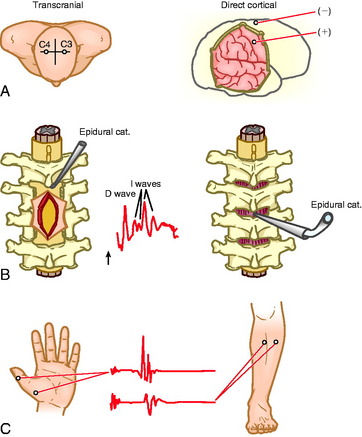
Fig. 11-2 A, Electrode positions for transcranial and direct electrical stimulation of the motor cortex. B, Schematic diagram of placement of an epidural catheter electrode inserted after a laminectomy (left) or inserted percutaneously (right). Middle, a typical epidural recording of D and I waves. C, Recording of the muscle MEPs from the thenar and tibial anterior muscles.36
From Deletis V, Rodi Z, Amassian VE: Neurophysiological mechanisms underlying motor evoked potentials in anesthetized humans: Part 2. Relationship between epidurally and muscle recorded MEPs in man. Clin Neurophysiol 2001;112:445–452, with permission.
D Waves
Electrical anodal stimulation of the cerebral cortex produces action potentials in the pyramidal cell neurons within the corticospinal tract, generating D waves (“direct”), as well as cortical interneurons that lead to subsequent activation of further pyramidal tract neurons, generating I waves (“indirect”).37 Unlike I waves, the D waves are elicited by direct stimulation of the axon within the white matter and thus will remain intact with ablation of the cerebral cortex and its cortical synaptic function. In addition, even though anesthetic agents affect cortical synaptic activity, resulting in a depression of I waves, action potential propagation along axons remains relatively unaffected. Thus, consistently reproducible D waves can be recorded from electrodes close to the spinal cord,6 either in the epidural space or transcutaneously (Fig. 11-3).38 Changes in D waves that are regarded as criteria for alarm include a greater than 50% drop in amplitude from baseline.39
Compound Muscle Action Potentials
TcEMEP also can be monitored by direct recording of EMG activity in contralateral limb musculature. This requires further synaptic transmissions in the spinal cord at the alpha motor neurons where anesthetic agents may depress synaptic activity. To overcome this, there needs to be a temporal summation of multiple excitatory postsynaptic potentials (EPSPs) to result in the firing of an alpha motor neuron. This may be accomplished by multiple I waves. However, anesthesia suppresses I waves. With a single electrical stimulus, only a D wave is elicited.36 Thus, high-intensity trains of transcranial electrical stimuli are necessary to elicit CMAPs.40 This results in multiple D waves sufficient to fire the alpha motor neurons and result in recordable CMAPs (Fig. 11-4). Multi-pulse stimulators are used to accomplish this with an interstimulus interval (ISI) long enough to overcome the refractory period of the pyramidal tract axons and short enough to prevent decay of the EPSP within the alpha motor neuron (usually 2–4 msec). If the ISI is too short, the D waves following the first one are attenuated because of the refractory period of the pyramidal tract axons; if the ISI is too long, the EPSP within the alpha motor neuron will decay, resulting in a reduced temporal summation sufficient to depolarize it.6,41,42 However, because the population of alpha motor neurons that are depolarized changes with each stimulus train, the CMAPs produced are variable with each stimulation. Also, there are reports that up to 80% decreases from baseline amplitudes are not correlated with postoperative motor deficits.43 Thus, most centers use an “all-or-none” response with regard to CMAPs.40
WHAT TO MONITOR
Spinal Epidural D Waves or Compound Muscle Action Potentials
With simultaneous monitoring of spinal epidural D waves and intramusculature electrodes eliciting CMAPs, D waves may persist while CMAPs are lost. Clinically, this can correlate with a transient postoperative neurological deficit. On the other hand, loss of D waves correlates with a permanent postoperative neurological deficit. Additionally, in some patients with intramedullary spinal cord tumors and preserved motor function, D waves recorded rostral to the tumor cannot be recorded caudal to the tumor. However, CMAPs may still be recordable in some of these patients.35 Thus, loss of CMAPs may represent a period during which compromise to the motor pathway is still potentially reversible.
The advantages of D waves are that they are not affected by anesthesia or neuromuscular blockers, require only a single stimulus, and are less variable in morphology. However, disadvantages are their increased invasiveness, lack of ability to lateralize the corticospinal tract, potential lack of recordability in patients younger than 21 months, and lack of change at a potentially reversible time of compromise to the motor pathways.6,44 Thus, while both D waves and motor unit potentials (MUPs) may be used simultaneously, most centers rely solely on CMAPs.6
Direct Spinal Cord Stimulation
Direct intraoperative, translaminal, electrical stimulation of the spinal cord or epidural space45 intended to monitor motor function while recording responses in the peripheral nerve or muscle, called neurogenic motor evoked potentials (NMEP), was proposed as a method of monitoring the motor system.46,47 However, this stimulation has been found to activate both sensory and motor pathways. By reflex stimulation of motor neurons at lower spinal levels, it leads to a myogenic response while bypassing the descending motor tracts.48 After stimulation, an action potential is antidromically produced in the same sensory fibers as those pathways mediating SSEPs.49 As such, direct spinal cord stimulation should be used exclusively to evaluate the functional integrity of the somatosensory pathways mediated by the dorsal columns.50
Direct Corticospinal Tract Mapping
Mapping of the pyramidal tract within the spinal cord has not been rigorously validated with regard to value and correlation with postoperative neurological outcome.51
Electromyography
To prevent postoperative radiculopathy, both free-running and triggered EMG can be monitored. Mixed-nerve SSEPs may not detect injury to specific nerve roots.52 Dermatomal SSEPs are more sensitive than mixed nerve SSEPs in detecting radiculopathy but are slow to attain and provide information regarding each individual sensory nerve root function only.8,53 EMG, however, is more sensitive than either SSEPs or DSSEPs in the detection of radiculopathy.12
Spontaneous motor activity can be recorded from multiple channels simultaneously as continuous free-running EMG. These are recorded from needle electrodes in muscles corresponding to various nerve roots thought to be at risk for intraoperative injury. The technique involves the insertion of paired intramuscular needle or wire electrodes into muscles after anesthesia. Trauma to these nerve roots will cause them to depolarize, resulting in an action potential in the motor axon that leads to EMG activity in the corresponding muscle,54 which can be visualized as well as heard on speaker to provide immediate feedback to the surgeon. These neurotonic discharges can alert the surgeon to prevent irreversible injury to the nerve roots. However, trauma is less likely to illicit neurotonic discharges from abnormal nerves.55 Also, EMG may remain silent during nerve transection.56 Anal and urethral sphincters also can be monitored to preserve bowel and bladder function.57
Triggered EMG also can be recorded via electrical stimulation of motor nerves. A monopolar or bipolar hand-held sterile stimulator is used by the surgeon. For monopolar stimulation an anode is remotely placed. This provides more widespread activation, useful for identifying deep structures during surgical dissection.53 Bipolar stimulation provides a localized current. This prevents current spread to adjacent structures and is useful for evaluating a specific nerve root. Hook electrodes and a dry surgical field will prevent false negative responses caused by current shunting in pools of fluid.53 Triggered EMG will evoke CMAPs in the innervated muscles.53 Intraoperative CMAPs differ from those recorded in the electrodiagnostic laboratory, in that the former are obtained by submaximal stimulation and result in “all-or-none” polyphasic responses with variable onset latencies and amplitudes.53 However, stimulus thresholds required to evoke a CMAP can provide information regarding nerve function.58
Muscle selection is important so that appropriate muscles for nerve roots potentially at risk are monitored. The intramuscular needle electrodes are placed after the patient is under general anesthesia. As a result, knowledge of surface anatomy is vital so that placement in the appropriate muscles is obtained, since the patient cannot voluntarily activate the muscles to confirm correct placement. Multiple muscles often must be monitored to accurately localize a particular nerve root, given the co-innervation in muscles.53
Criteria for alarm for spontaneous EMG activity would include irregular EMG bursts consistently elicited by a surgical maneuver, prolonged (>3 sec) semirhythmic neurotonic discharges, or an active EMG signal becoming acutely silent (Fig. 11-5).59
APPLICATION TO TUMORS
SPINAL CORD
Somatosensory Evoked Potentials and Motor Evoked Potentials
Given that the function of the sensory and motor pathways can be affected separately during intramedullary60 and extramedullary spinal tumor surgery,61 both systems should be monitored for potential compromise.
SSEPs and MEPs have been shown to be beneficial for the resection of intramedullary spinal cord tumors.39,62 It has been shown that the monitoring of SSEPs during intramedullary spinal tumor surgery can reduce postoperative spinal cord impairment. This is accomplished by warning the surgeon about potential spinal cord damage, resulting in prompt intervention before permanent injury occurs.63
However, insults to the spinal cord appear to be detected by TcEMEP before those detected by SSEPs. This may be a result of a selective vulnerability to trauma of large diameter, thickly myelinated, fast conducting fibers, which are the predominant composition of the corticospinal tract axons mediating MEPs.25
In fact, MEP monitoring has been shown to offer a better predictor for postoperative neurological outcome than the preoperative motor status of the patient itself.39 Monitoring with both SSEPs and MEPs can reassure the surgical team that the surgery has not caused additional injury when the EPs remain unchanged.43,64
Recently it has been shown that the combined use of TcEMEP and free-running EMG may in fact be advantageous in monitoring the motor pathways during resection of intramedullary spinal cord lesions compared with either method alone.59
Invasive Techniques
SSEPs also can be used for the initial planning of the myelotomy incision. After the anatomical visualization of the midline by branches of the dorsal medullary vein penetrating the median sulcus, SSEPs can be obtained by serially stimulating at 1-mm intervals on either side of the midline. The region where there is no response can be selected for posterior myelotomy.61 This may be particularly important for intramedullary metastatic tumors, given the distorted anatomy common with these neoplastic lesions.25 However, myelotomy for resection of intramedullary tumors can on its own result in a reduction of dorsal column integrity25 and result in a deterioration in SSEPs after myelotomy retraction.62
Epidurally recorded SSEPs after peripheral nerve stimulation can be made from both above and below the surgical site. Latency values between the two electrodes can be calculated to provide a conduction velocity through the surgical region.27
Direct electrical stimulation of the dorsal spinal cord caudal to the intramedullary tumor with recording via an epidural electrode placed rostral to the tumor has been attempted to produce SSEPs. These were thought to be superior to standard SSEPs by staying present after peripheral nerve stimulated SSEPs were abolished, providing faster responses with larger amplitudes, having a higher sensitivity to spinal cord insult, and better predicting the postoperative outcome.65 However, this method has in fact been found to activate multiple tracts, both ascending and descending, simultaneously and as a result may produce false negative results.45
Interpretation
An amplitude decrease of 50% and latency increases of 10% in SSEPs typically are used as cause for alarm.7,27 With these changes, the patient is at risk for new postoperative neurological deficits, especially if the SSEPs do not recover by the end of the procedure. Complete loss of SSEPs or MEPs, though, may result in new neurological postoperative changes in only approximately 50% of cases.27 False positive cases could be explained by prompt anesthetic or surgical intervention based on the EP changes.
Direct Spinal Cord Mapping
A monopolar or bipolar stimulator is used by the surgeon to stimulate the surgical field with observation of EMG recording in the extremities. EMG recording with direct spinal cord stimulation may be beneficial in determining the interface between the tumor and normal spinal cord tissue, particularly for ventral tumors and intramedullary metastasis, as well as for intramedullary spinal cord mapping. Traction on the tumor capsule itself can endanger the pyramidal tract axons, resulting in acute changes in TcEMEPs.25
NERVE ROOTS
DSSEPs may be useful for cauda equina tumor removal by possibly being sensitive to changes in nerve root function.17 However, there are significant technical limitations in their production, and they may not be sensitive in predicting changes during nerve root decompression.7,9
EMG is more sensitive than DSSEPs for the detection of radiculopathies.12 EMG is sensitive to blunt mechanical trauma of nerve roots resulting in neurotonic discharges. These are high-frequency bursts of MUPs.52 However, with sharp nerve transaction, EMG may in fact be quiescent.56 In addition, the distal stump of the nerve can still activate myogenic responses by mechanical or electrical irritation,52 thus leading to a false negative response.
Chronically compressed nerve roots and nerve roots that have undergone axonotmesis (Wallerian degeneration) require higher stimulation thresholds (i.e., >10–20 mA [normal stimulation threshold is 0.2–5.7 mA]).55,58 Even though this has been applied predominantly to pedicle screw testing, it also may have implications in spinal tumor surgery.
CONUS MEDULLARIS
Along with SSEPs and MEPs to monitor the sensory and motor tracts, anal sphincter EMG can be used to monitor anal sphincter mechanical activity and can be extrapolated to external urethral sphincter activity because both are striated muscles innervated by the pudendal nerve that arises from the S2, S3, and S4 sacral nerve roots. This is particularly useful during conus medullaris, cauda equina, or sacral nerve root surgery. After the patient is under general anesthesia, an electrode-containing anal plug or two needle electrodes can be inserted into the anal sphincter muscle. They can then record free-running EMG or can be stimulated to determine triggered EMG responses of structures thought to be at risk.66
CAUDA EQUINA
The electrical stimulation of structures with recording of triggered EMG in the corresponding lower extremity and anal sphincter muscles to identify neural elements has been shown to be beneficial in cauda equina surgery.67 Visual examination may not be adequate in identifying functional neural structures or in determining the functionality of nerve roots. Even though DSSEPs may aid in identifying compromise in a sensory nerve root,54 the technical limitations associated with their acquisition raise questions about their usefulness.
ANESTHESIA
Anesthetic agents produce their effects through the alteration of synaptic function or axonal conduction, leading to alterations in neuronal excitability.68 Thus, cortically generated signals, which are subject to more synapses, are more sensitive to alteration from anesthetics than the subcortical signals. However, certain agents have more profound effects on EPs, namely the volatile inhaled anesthetics (e.g., nitrous oxide, halogenated inhalants) as compared with intravenous agents (e.g., opioid analgesics). Nitrous oxide in particular, when combined with other inhaled anesthetics, produces a synergist depression in SSEPs. With changes in concentrations, the effects also can change rapidly.48
SOMATOSENSORY EVOKED POTENTIALS
As mentioned, SSEPs are responses occurring from the stimulation of a peripheral nerve that orthodromically enter the spinal cord through dorsal roots, where they then ascend in the white matter dorsal column pathways to synapse in the medullary nuclei.6 They finally synapse in the thalamus and project to the primary somatosensory cortex.7 Thus, the SSEP responses remain relatively unaffected by anesthetic agents up to the level of the medulla, but a dose-related increase in latency and decrease in amplitude of the cortically generated SSEPs result from halogenated inhalational anesthetics. However, during spinal surgery the cortical responses become less important when a subcortical EP can be recorded from the spinal cord/brainstem above the level of the region at risk.
TRANSCRANIAL ELECTRIC MOTOR EVOKED POTENTIALS
As mentioned, D waves are generated by activation of the pyramidal tract neurons in the motor cortex of the brain or the subcortical white matter. They can be recorded without synapsing in the epidural space of the spinal cord. I waves, however, rely on synapses within the motor cortex. Thus, D waves will be relatively unaffected by anesthetic agents, whereas I waves will be affected when recorded over the spinal cord.48
TcEMEPs also can be recorded as CMAPs within the muscles themselves. This, however, requires further synapses in the spinal cord at the level of the anterior horn cells (AHCs). The firing of multiple AHCs is required to produce a CMAP, thus if high anesthetic concentrations are used, there is the possibility that not enough AHCs may fire to generate a CMAP. Also, CMAPs are dependent on synapses at the neuromuscular junction (NMJ), where anesthetic drugs have little effect aside from NMJ blocking agents.48
PREFERRED ANESTHETIC REGIMEN DURING INTRAOPERATIVE NEUROPHYSIOLOGICAL MONITORING
For EPs with multiple synapses (namely cortical SSEPs and possibly CMAPs), volatile inhaled anesthetics should be avoided or limited. If they are used, they should be restricted to less than 0.5 mean anesthetic concentration (MAC).48 Total intravenous anesthesia (TIVA) using non-inhaled anesthetics (e.g., opioids, propofol) would be preferred in these situations. However, the subcortical SSEP components that are able to monitor a distal spinal cord region at risk would be relatively unaffected by inhaled anesthetics.
For responses that are dependent on NMJ transmission (namely MEPs and EMG), paralytic agents should be avoided.48 However, a constant infusion of neuromuscular blocking agents to provide partial paralysis may be desired to reduce patient movement during TcEMEP. This infusion should, however, remain constant to avoid anesthetic-related changes in CMAP response.40
SYSTEMIC FACTORS
Blood Flow
There is a relationship between regional cerebral blood flow (CBF) and cortical EPs.48 Normal CBF is globally 45–55 cc/100g/min (75–80 cc/100g/min for the cortex and approximately 200 cc/100g/min for subcortical structures). Cortical SSEP responses are unaffected by CBF down to approximately 20 cc/100g/min.48 However, when CBF reduces to 14–16 cc/100g/min, the cortical SSEP amplitude decreases by 50%. This is important because ischemic infarction of tissue does not occur until CBF reaches 12 cc/100g/min. Thus, cortical SSEPs can be the first indicator of potentially reversible ischemia caused by hypotension.22
Regional ischemia on the spinal cord or nerve roots caused by mechanical or regional vascular insufficiency also may be first noted by the monitoring of EPs. In these cases, the mean arterial pressure can be increased to improve cord perfusion.25
Hematocrit
Hematocrit reflects oxygen carrying capacity, and hence oxygen delivery, as well as blood viscosity. Maximum oxygen delivery occurs when the hematocrit is between 30–32%. EPs also are consistent within this range.48
Oxygen and Carbon Dioxide
Alterations in SSEPs can occur from hypoxemia and hypocapnia.69 These may lead to vasoconstriction, resulting in changes in SSEPs and potentially MEPs during spinal surgery.
Temperature
Hypothermia can affect SSEPs and MEPs. Extremity cooling during surgery from ambient temperature or spinal cord cooling from cold irrigation can prolong the latencies of all peaks of SSEPs.22,48 It also has been shown that MEP onset can become gradually delayed and an increase in stimulation threshold can occur as core temperature, recording from the esophagus, decreases from 38 to 32°C.48
SPECIAL CONSIDERATIONS IN THE PEDIATRIC PATIENT
Intramedullary tumors are rare in children, accounting for only 35–40% of all intraspinal tumors.2 Even though MRI remains the diagnostic study of choice,3 just as in adults, the pediatric patient may come to the attention of the neurologist for electrodiagnostic evaluation. As in adults, the same studies, namely EMG, NCS, and EPs may be performed in infants and children. The pediatric population also has shown to benefit from intraoperative monitoring during spinal deformity surgery.70 However, there are important physiological differences because of neural development. As well, a varied approach based on physical size and behavior must always be considered when working with children.71
Neurodevelopmental Physiology
Myelination begins between the 10th and 15th weeks of gestation.71 There is, however, a clear difference in postnatal maturation of motor nerve fibers in the peripheral nervous system compared with that of the central nervous system.72 Because nerve conduction velocity depends on axon length and diameter, myelin thickness, node of Ranvier width, and internode distance, nerve conduction velocities will vary with the age of the patient. Peripheral myelination is complete by 5 years of age, and this correlates with NCS reaching adult values by 4 to 5 years of age.71
However, as compared with the peripheral nervous system, there is a delay in the post-myelination growth rate of the central nervous system pathways. Conduction velocities in the corticospinal pathways range from 47–75 m/sec in adults. In comparison, full-term newborns have values four to five times lower than that for adults. This also appears to remain unchanged during the first 3 months of life, reflecting the slow maturation of the central motor pathways during this time. There is a rapid increase in the rate of conduction beginning at 2 to 3 months of age and continuing until 15 months of age. There is then a slower increase up until adult values are reached by the age of 8 years. Central motor pathway myelination then appears to occur in three stages, with a slow increase in conduction velocities between birth and 3 months of age, followed by a more rapid increase until the first year of life, and then a slower increase until 8 years of age.72
There also is a difference in motor fiber myelination as compared with sensory fiber myelination. Motor fibers are myelinated in a cephalocaudal route, whereas sensory fibers do so in the opposite caudocephalic route. Also, myelination of the pyramidal tracts occurs later than that of the dorsal column tracts.72 This clearly would have implications on the attainment of SSEPs and MEPs.
Application to Tumors
Spinal cord tumors in children may affect EPs differently from those in adults. In fact, in some circumstances, EPs may be more difficult to attain in children with spinal cord tumors. This may be a result of the immature neural structures in children being more vulnerable to tumor compression. Also, neoplasms tend to extend multiple levels in children as compared with adults.73
However, the timing during development of tumors in early gestation may actually result in the preservation of EPs. It has been found that children younger than 9 to 10 years often retain SSEPs after dorsal midline myelotomy.62 In these cases, the tumors may have developed early enough during gestation to lead to dorsal column displacement away from the midline.73
For MEPs, recordable CMAPs after TcEMEP and TMS in both term and preterm neonates have been described.44,72 With regard to spinal cord tumors, in one study assessing children ages 8 to 36 months with intramedullary spinal cord tumors, the D waves were recorded from the lower thoracic spinal cord in the youngest child aged 21 months of age when elicited by transcranial electrical stimulation and recorded epidurally.44
Finally, just as in adults, free-running EMG and mapping techniques with EMG and perhaps DSSEPs during conus medullaris and cauda equina surgery should be done to identify the filum terminale and nerve roots.73
22 López JR. Intraoperative neurophysiological monitoring. Int Anesthesiol Clin. 1996;34:33-54.
27 Nuwer MR. Spinal cord monitoring. Muscle Nerve. 1999;22:1620-1630.
46 Burke D, Hicks RG. Surgical monitoring of motor pathways. J Clin Neurophysiol. 1998;15:194-205.
53 Holland NR. Intraoperative electromyography. J Clin Neurophysiol. 2002;19:444-453.
68 Sloan TB. Anesthetics and the brain. Anesthesiol Clin North Am. 2002;20:265-292.