2 Early developmental events
Development of the nervous system
The location of the cells destined to develop into the neuraxis (nervous system) is probably already defined in the late gastrula stage of the embryo, at about 18 postovulatory days. At this point the embryo is about 1.5 mm in length (Huttenlocher 2002).
The development proper of the nervous system can first be identified at approximately 3 weeks (21 days) after conception, with the appearance of the neural plate. Originally the neural plate is roughly the shape of a ping-pong paddle, but soon develops a distinct neural groove, flanked by neural folds (Fig. 2.1). This fold is eventually drawn together by contractile tissue deep to the neural groove which pulls the folds together. Initially, while the tube is closing, its walls consist of a single layer of neuroepithelial cells. Each of these cells contacts both the internal (luminal) and external (basal) limiting membranes. This fold fuses by the end of the third week to the early fourth week, generally in what will eventually become the cervical region of the spinal cord and then extends zipper-like, rostrally forming the brain proper and caudally forming the thoracic, lumbar, sacral, and coccygeal regions of the spinal cord (Fig. 2.2).
The neural tube is originally open to the amniotic cavity by virtue of the neuropores located both rostrally and caudally, but by the end of the fourth week the neural tube closes at both ends with the closure of the neuropores. At the rostral end of the neural tube the primitive forebrain divides into two cerebral hemispheres and forms the two lateral ventricles and the third ventricle. The embryo is about 7 mm long at this point (day 32) in development (Behrman & Vaughan 1987). Once the neural tube has closed, the ectoderm forming the lips of the neural fold separates and forms the neural crest tissue (Fig. 2.3).
The neural crest cells give rise to several components of the peripheral nervous system, as well as a number of non-neural tissues. Tissues eventually formed by the neural crest cells include pigment cells of the skin; medullary cells of the adrenal gland; calcitonin-secreting cells of the thyroid gland; neurons of the paravertebral ganglia; many of the neurons in the ganglia of the cranial nerves V, VII, VIII, IX, and X; and neurons of the dorsal root ganglia (Leikola 1976) (Fig. 2.4). The cells of the neural crest disperse by migrating along well-defined pathways to their destinations. The phenotype of each cell seems to be largely determined by the position that they eventually occupy (Le Douarin 1982). For example, cells destined to migrate to the adenyl medulla (norepinephrine-secreting cells) have been experimentally transplanted to sites that give rise to cholinergic (acetylcholine-secreting) cells. The transplanted cells converted to cholinergic-secreting neurons (Cowan 1992).
The continued growth and expansion of the neural tube leads to the stimulation of overlying ectodermal tissues at various sites called ectodermal placodes. These ectodermal placodes lead to the development and formation of the sensory epithelia and cranial nerve ganglia. The various placodal development sites include the olfactory placode, the trigeminal placode, the auditory/vestibular placode, the facial placode, the vagal placode, and the glossopharyngeal placode.
These early stages of development are largely dependent on the processes of primary embryonic induction, which is largely under genetic control (Williams & Warwick 1980).
Gross morphological development
Shortly after the closure of the neural tube, a series of three vesicle-like swellings appear at the rostral apex of the tube. These swellings will eventually form the three primary brain vesicles: the prosencephalic (forebrain), the mesencephalic (midbrain), and the rhombencephalic (hindbrain) areas. Through various folding patterns the three primary vesicles ultimately give rise to the mature brain structures. The development of the three primary vesicles into their representative mature structures results in the following progression: the prosencephalic region develops into the telencephalon and the diencephalon; the mesencephalon remains undifferentiated, giving rise to the mature mesencephalon; and the rhombencephalon develops into the myelencephalon and metencephalon. Each of these secondary brain vesicles further develops into the recognised mature divisions of the human brain (Figs 2.5 and 2.6).
Primary developmental processes
The development of the mature nervous system is brought about by a series of steps that include processes both progressive and regressive (Cowan 1978). These steps include cell proliferation, neuronal migration, selective cell aggregation, cytodifferentiation, axonal outgrowth, and synapse formation. The first regressive events occur about the time that neurons in each population begin to form connections within their prospective projection fields. This phase is marked by the selective death of a substantial proportion (50%) of the initial population of prospective neuron cells. Many connections that were initially formed are eliminated and certain axon terminals are withdrawn. A large number of axon collaterals are also removed at this stage (Cowan & Hunt 1985). Each phase is considered in detail below.
Cell proliferative phase
The wall of the neural tube is formed initially by pseudostratified columnar epithelium that rests on the external basement membrane. Each cell sends a peripheral process to both the external (basal) lamina and the neural tube internal (luminal) surface. At the luminal surface a complex of junctional connections allows communication with other cells. The nuclei are found at different levels within the neuroepithelium, except those in the later stages of mitosis which are constantly found at the luminal aspect (Cowan 1981). The young neurons constantly migrate from the internal or luminal lamina to the external or basal lamina in a process called interkinetic nuclear migration. Throughout this process the neurons must continually retract and reform their peripheral processes. At this stage of development the neural tube consists of two histological areas of tissues, the ependymal or germinal cell layer found on the luminal aspect of the tube and the ventricular zone or matrix layer which spans the remainder of the tube and is in contact with the basal membrane. Continued cell division leads to growth and expansion of the neural tube in three ways: general expansion of the neural tube surface epithelium, rapid growth of the brain and spinal cord, and differentiation of the different lineages of cell types including glial cells and neurons. This differentiation phase is marked by a migration of some of the cells from the ventricular zone to the newly emerging intermediate or mantle zone, away from the luminal surface. The neurons in the intermediate zone send processes outward towards the basal membrane which eventually develops into the marginal zone. To summarise, at this point (4 weeks or 28 days) the development of the neural tube consists of four histologically identifiable layers: the ependymal or germinal layer, from which all the cells of the central nervous system will develop; the ventricular or matrix layer, which consists of newly formed cells undergoing interkinetic nuclear migration and mitosis; the intermediate or mantle zone, which consists of cells migrating from the intermediate zone; and the marginal zone, which consists of the elongated cytoplasmic processes of the cells in the intermediate zone. Interposed throughout these layers are developing glial cells including the radial glial cells which seem to act as guide cells for the migrating neurons (Fig. 2.7).
Each population of neurons is generated during a distinct period of embryonic or fetal life. This period is usually quite short, ranging from a few days to a week. Cells born of the same cohort can be classified as embryological homologues. The relationships that each cohort of neurons forms with other neurons of the same cohort become very important as the system matures. These relationships can be used clinically to gain insight into the function of other related homologous systems by stimulating one system of neurons and measuring the response of a homologous system through a variety of clinical testing procedures (see Embryological Homologous Relationships later in this chapter). The sequence at which the cells withdraw from the proliferative pool is well defined. For example, in the retina the ganglion cells farthest from the original luminal zone are generated first, followed by the inner nuclear layer, which are in turned followed by the photoreceptors. The actual sequence may vary somewhat from region to region; for example, the situation in the motor cortex is completely reversed with the deepest cells forming first (Fig. 2.8).
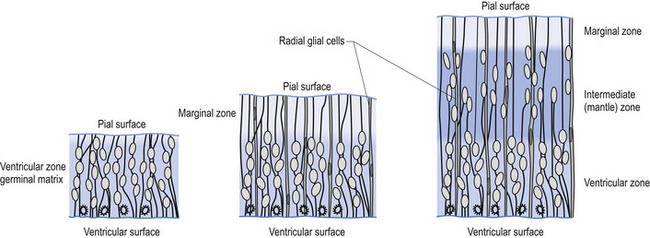
Figure 2.8 A magnified diagrammatic view of Fig. 2.7. The various cell proliferation zones, the intermediate zone, and the migration of the neurons via the radial glial cells are illustrated.
The process of neurogenesis seems to be highly programmed in both space and time. The process seems to progress largely in a ventral to dorsal, and cervical to sacral gradient (above, down, inside, out). In keeping with this strategy, the motor systems arising from the basal plate located ventrally are generally generated before the sensory systems arising from the alar plate, which is located dorsally (Figs 2.9A and 2.9B).
Neuronal migration
Peripheral nervous system
Neural crest and placodal precursor cells migrate along predetermined, but as yet molecularly undefined pathways to their various locations in the cranial, spinal, and autonomic ganglia. These cells undergo the majority of their proliferation once they reach their definitive destinations. There is good evidence to suggest that the neural phenotype of most neural crest cells is largely determined by the regions through which they migrate and the location in which they ultimately settle (Sidman & Rakic 1974). Neuropathologies can develop when cells migrate too far or in the wrong direction. This situation results in the condition referred to as neuronal ectopias.
Central nervous system
In the central nervous system the situation is quite different from the peripheral nervous system in that all cells must undergo at least one migratory phase as a rudimentary neuron or glial cell before locating to their final destination. The cells of the peripheral nervous system are stem cells or immature neurons when they start their migration. The rudimentary cells have a primitive form of differentiation that identifies the cell as either a neuron or a glial cell when they start their migration out of the nuclear zone. The final differentiation of the cell occurs throughout its migratory process through interactions with the various other cells along its determined pathway and is completed when it reaches its final destination through associations with other cells also located there.
Neuronal cytodifferentiation
Morphological differentiation
This process involves the development of a number of different outgrowth processes from the neuron cell body that will eventually become the dendrites and axons of the neuron. The process usually begins with the development of a single axon and one or more dendritic processes. Initially, the outgrowths all look remarkably similar and contain the same organelles, including ribosomes that will disappear in the axon processes but remain in the dendritic processes as both structures mature. At this point in their development they are referred to as neurites. The establishment of the dominate axon seems to be dependent on a predetermined polarity thought to be produced by chemical morphogenic gradients produced by guide cells and locator cells in the region of the final destination of the neuron. These morphogenic gradients may act to induce a certain family of genes responsible for the production of growth-associated proteins (GAPs). These GAPs are involved with the rapid elongation of the axon at a highly specialised structure referred to as the growth cone of the axon. Growth occurs through continuous addition of cytoplasmic and membrane components supplied by the neuron cell body via anterograde axonal transport to the growth cone. This axonal transport can reach velocities of up to 200 mm/day. The axon itself can reach growth velocities of up to 5 mm/day (Cowan 1992). Some axons, such as those of the giant pyramidal cells of Betz, can grow to over a metre in length! In such cases it could potentially take proteins and transmitter substance 5–6 days to reach the axon terminal.
The formation of dendrites also occurs at a specialised growth cone structure.
Physiological and molecular differentiation
The cell membrane components necessary for the development of membrane and action potential production, including enzymes, transmembrane proteins, gap junctions, and specific receptors, do not appear simultaneously in the evolving neuron. The development of these specialised membrane structures seems to follow a specific sequential order of appearance and function in most neurons. In the early developmental period when the cells are still in the interkinetic nuclear migration cycle in the ventricular zone they develop electrically coupled (gap) junctions. Just prior to leaving the ventricular zone the cells undergo an uncoupling of their gap junctions. This uncoupling phase is replaced by long-lasting (10–100 ms) action potentials produced by calcium ion fluxes across the membrane. The next phase of development is heralded by the appearance of much shorter (1–2 ms) sodium-produced action potentials superimposed over the long-lasting calcium action potentials. In the final stage of development, in most neurons, the calcium slow potentials disappear, leaving only the sodium action potentials active in the neuron (Spitzer 1981). A complex relationship between calcium and sodium interaction remains in most mature neurons with the permeability of sodium across the neuronal membrane inversely proportional to the concentration of extracellular calcium. It is not clear why this sequence of events occurs in most neurons but it outlines the importance of temporally pre-programmed expression of genes in the development of ion-specific protein channels so important to the establishment of neuron function.
The functional attributes of a neuron begin by the production of at least one group and sometimes several groups of neurotransmitter synthesising enzymes. Thus a single neuron may produce more than one neurotransmitter. In conjunction with the appearance of these specialised transmitter enzymes, enzymes for the production of neuropeptides, one or several transmitter receptors proteins, pro-oncogenes, growth factor receptor proteins, insertion proteins, and structural maintenance proteins are also produced (Black et al. 1984).
Establishment of neuronal connections and axonal pathfinding
Is the formation of the multitude of connections in the nervous system random?
The short answer is probably not. There is insufficient genetic material in any individual neuron to code for all of the neuronal connections that need to develop, break down, and reform throughout the life of a neuron in a functional nervous system (Kandel et al. 1995). However, there is a good deal of evidence to suggest that neurons have innate predetermined programmes that lay out the basic patterns of connections to be formed initially in their development. Little is known about the mechanism of implementation or of how the information is actually stored in the neurons. Predetermined connection fields develop quite early in some neurons, perhaps as early as their positional determination in the neural plate is achieved. This is particularly true in neurons developing as retinal cells. These cells seem to have developed a positional orientation or map of their location in the retina before they start developing their axons which will form the optic nerve. This positional orientation is temporally dependent, although initially maintaining a degree of flexibility, after a certain time period becomes permanently fixed (Cowan & Hunt 1985). This same pattern seems to apply to other areas of the nervous system also. The initial neurons in any given location seem to be under the influence of a gross general polarity-based guidance system that operates throughout the entire body of the developing embryo. This general positional system is responsible for guiding the initial neurons of a particular local to their destination. Initially, this system can be altered or reversed if the conditions in the location are not optimal. Once the original neurons become established they act as guideposts for further infiltration by additional neurons. At a critical point this process becomes irreversible and the destiny of each neuron becomes fixed. How these neurons determine where they need to be from a cellular level is again theoretical, but may be best explained by the chemoaffinity hypothesis first proposed by Sperry in 1963 and further developed by Hunt and Cowan in the 1990s. This theory proposes that the positional address of these cells becomes coded on the cell membrane in the form of a distinct labelling molecule or grouping of molecules that allow neurons to differentiate between areas of attraction and repulsion. The neurons would naturally gravitate to areas of attraction and move away from areas of repulsion, eventually arriving in the most attractive environment (Sperry 1965).
How do axons know where to go?
Developing ganglion cells in the inferior nasal portion of the retina send their axons to the lateral geniculate body of the thalamus, whereas developing ganglion cells of the superior temporal retina send their axons to the superior colliculus of the midbrain (Fig. 2.10).
Axons tend to grow in the direction that follows a selective substrate pathway specific for certain axonal membrane receptors contained on the surface of their developing axons. These molecules or receptors include various integrins such as fibronectin and laminin. Tropic influences include substances that promote axon growth along a concentration gradient. One such factor is nerve growth factor (NGF), which has been shown to exert strong growth influences on sympathetic nerve axons to the extent in some cases of causing them to change direction (Gundersen & Barrett 1979).
Synaptogenesis
When the growth cone of an axon comes into close proximity of a postsynaptic cell surface at a potential target destination the terminal portion of the growth cone starts to accumulate vesicles. At the same time, morphologic changes occur on the pre- and postsynaptic membranes that allow the presynaptic transmitter to be recognised by the postsynaptic receptors. Functional synaptic integrity has been observed within minutes of the initial contact between an axon growth cone and a target muscle at acetylcholine (ACh) neuromuscular junctions (Kidokoro & Yeh 1982). Initially, the effect of the transmitter on the postsynaptic receptors is quite variable but as the functionality of the synaptic connection becomes stabilised, the action of ACh on the postsynaptic receptors results in a progressively shorter opening time of the sodium depolarising channels until a fairly consistent opening and closing time becomes established.
Most neuronal systems undergo a phase of substantial neuron death at some phase of their development. In most neuron systems about 50% of the initial neurons formed undergo cell death. This process usually occurs temporally at the same time that the axons of the system have formulated contacts with their destination areas. This suggests that a certain amount of the stimulus for neuron death may actually arise or be initiated from the axon destination field through some form of feedback system (Hamburger & Oppenheim 1982). The feedback mechanism may be in the form of tropic growth factors produced at the destination site tissues. Active competition by axons for these growth factors may determine which axons and thus which neurons remain alive. In the case of dorsal root ganglion (DRG) cells one such growth factor that has been isolated is neuron growth factor, without which DRG cells cannot survive (Levi-Montalcini 1982).
Not only is there an overproduction of neurons initially but most neurons establish many more synaptic connections than are necessary or than they can physically maintain. This results in a phase of synaptic elimination in most systems. This was first recognised at the neuromuscular junctions where in the mature system each muscle fibre is innervated by a single axon. Early in development, however, many (6–7) axons may innervate a single muscle fibre (Perves & Lichtmann 1980). This same pattern has been shown to occur in many other systems including the autonomic system and is now thought to be a common strategy in most neuronal systems (Perves 1988). It is important to point out that axon collaterals may be eliminated but the parent axon and other synapses of the parent axon can remain functional and actually multiply in some cases. The most notable example of this occurs in the cortex. Initially, all pyramidal cells of lamina V in all cortical areas send axons through the cortical spinal tracts. In the case of the visual and other inappropriate areas of cortex, the inappropriate axons but not the parent cell bodies are eliminated so that only axons from the pyramidal cells of the motor and some areas of sensory cortex remain in the cortical spinal tracts at maturity (O’Leary & Stanfield 1989).
Neural plasticity
Neural plasticity results when changes in the physiological function of the neuraxis occur in response to changes in the internal or external milieu (Jacobson 1991). Neuroplastic changes are stimulated under two basic conditions.
Injury-related plasticity is the response of the normal, remaining tissues to the demands placed on them following injury or disease. Commonly, the remaining tissues will ‘take over’ some or all of the functions of the damaged tissues over time. This concept is important in a variety of treatment approaches utilised in functional neurology. A striking example of this type of plasticity is the relative normal development of language in young infants who receive damage to their dominant hemisphere. In an adult, damage to the dominant hemisphere usually results in permanent, severe language comprehension and/or articulation problems. This does not occur in infants, even with a complete removal of the dominant hemisphere (hemispherectomy) if the damage occurs before the age of 3, who will, in most cases, develop language skills in a normal fashion. This is the result of other brain areas changing their own response patterns and taking over the responsibilities of the injured areas (Huttenlocher 2002).
The process of neural plasticity appears to occur through the reorganisation of synaptic contacts in a neural system in response to changing stimulus in such a way that synapses that receive more stimulation become strengthened and those that receive less stimulation become weakened (Hebb 1949; Lashley 1951). Not all areas of cortex have the same ability to undergo plastic changes. The hard-wired areas of the motor and sensory cortex do not respond to the same extent as certain areas of frontal cortex such as those areas responsible for higher cortical functions like language, mathematics, musical ability, and executive functions. For example, the same left-sided hemispheric injury described above in an infant that did not result in language difficulties will still result in right-sided paralysis or weakness.
Embryological homological relationships
A third example involves the neurons in the hippocampal formation and parahippocampal gyrus in the medial temporal lobe. During embryological development the neurons that originally were born side by side undergo an elaborate series of folding, resulting in neurons that are physically in different areas (Fig. 2.11). These neurons maintain their original synaptic connections and influence the central integrated state of the others in the functional group (Fig. 2.12). This neural circuit is involved in the development of memory.
Development of the vertebral column
A portion of mesenchymal tissue does not proliferate, but remains in the space between the sclerotomal development and results in the formation of the intravertebral disc. Embedded still more centrally is the remnant notochordal tissue, which eventually develops into the nucleus pulposus, which is later surrounded by circular fibrous tissue, the annular fibrosis (Sadler 1995) (Fig. 2.13).
A variety of spinal anomalies arise from the abnormal development or closure of the neural tube and/or fusion of the posterior aspects of the vertebral bodies. These anomalies include spina bifida occulta, spina bifida vera, diastematomyelia, and tethered cord (Guebert et al. 2005). The term spinal dysraphism refers to a variety of conditions in which the posterior aspects of the first or second sacral segments are involved.
Spina bifida occulta
Spina bifida occulta is a defect of the posterior arch of a vertabra in which one or the other of the developing pedicle segments fails to fuse to form the spinous process. In spina bifida occulta failure of the arch formation does not affect the development of the thecal sac or its contents. The most common areas of the spine involved are the lumbosacral areas. Clinical manifestations of spina bifida occulta usually only become apparent sometime after birth and include back pain, increased incidence of disc herniation, and spondylolisthesis (Fidas et al. 1987; Avrahami et al. 1994). Although neurological manifestations are rare, a number of conditions have been associated with spina bifida occulta. These include low termination of the conus medullaris (tethered cord syndrome), syrinx formation, lipoma, nerve root adhesions, and conjoined nerves (Giles 1991; Gregerson 1997).
Spina bifida vera
In situations involving spina bifida vera there is a wide bony defect in the posterior arch development of usually more than one vertebra. The thecal sac and its contents are usually also involved and protrude beyond the confines of the spinal canal. There is some evidence to suggest that an adequate supply of folic acid during this critical period of development can prevent this type of condition. Failure of fusion of the posterior arch to the degree necessary to result in spina bifida vera must take place in the period of the 21st to 29th fetal day. Unfortunately, this is a period in which most women do not realise they are pregnant; thus to be effective supplementation with folic acid must begin prior to conception. Herniation of the fluid-filled sac that contains cerebral spinal fluid is called meningocele. When protrusion includes the meninges, cerebral spinal fluid, and neural elements, it is called a myelomeningocele. When neural elements protrude without thecal covering, it is called a myelocele (Figs 2.14A and 2.14B). Myeloschisis refers to the presence of complete uncovering of the neural elements along a sagittal midline defect that involves bone, thecal sac, and all posterior tissues (Guebert et al. 2005) (Fig. 2.15). Failure of closure of the caudal neuropore results in absence of the cranial vault with the cerebral hemispheres either completely missing or reduced to non-functional masses. This condition is referred to as anencephaly (Figs 2.16A and 2.16B).
References
Behrman R.E., Vaughan I.I. Nelson’s Textbook of Paediatrics. Philadelphia: WB Saunders, 1987.
Hebb D.O. The Organization of Behavior. New York: Wiley, 1949.
Jacobson M. Developmental Neurobiology, third ed. New York/London: Plenum Press, 1991.
Lashley K.S. Central Mechanisms in Behavior. New York: Academic Press, 1951.
Le Douarin N.M. The Neural Crest. New York: Cambridge University Press, 1982.
Spitzer N. Development of membrane properties in vertebrates. Trends Neurosci.. 1981;4:169-172.
Williams P.L., Warwick R. Embryology. In Gray’s Anatomy. Edinburgh: Churchill Livingstone; 1980.