Chapter 168 Dynamic Stabilization of the Lumbar Spine
Indications and Techniques
Over the past 20 years, developments have been under way to produce spinal systems that control a painful “dysfunctional” spinal unit without causing fusion. Examples of this include facet replacements, interspinous process devices, and pedicle screw–based motion-controlling systems. These latter systems include motion-limiting systems (e.g., the Graf device), mechanical micromotion systems (e.g., Scient’x Isobar TTL), and the Dynesys system. This latter system is the most commonly utilized system in the world today and is reviewed in detail in this chapter. While the theory of these systems is to restore stability yet provide motion, their efficacy has not been completely proved.1 the U.S. Food and Drug Administration (FDA) has not approved any pedicle screw–based system as a stand-alone device for nonfusion applications. The systems available for use, including the Dynesys system and others, are cleared through the FDA 510(k) process for fusion applications as being substantially equivalent to existing pedicle screw–based fusion augmentation devices.
Biomechanical Rationale
Both the spine surgeon and the implant design engineer benefit substantially from a clear understanding of the native function of the spine and the contribution of each of the spinal elements. The motion of the spine can be studied in the most basic form at a single level that begins with the functional spinal unit (FSU). The FSU comprises two vertebral bodies with their articulations, including the intervertebral disc, as well as the two posterior facet joints. The intervertebral disc forms an integral part of the FSU and has a high potential of becoming problematic, especially with age. Anatomically, the disc consists of fibrous layers arranged in alternating lamellar structures, in conjunction with a gelatinous inner core referred to as the nucleus pulposus. The other important articulations within the FSU are the facet joints, which are also susceptible to disease. Facets, in the normal condition, play a role in controlling the motion of the FSU. This three-joint complex within each FSU controls the kinematic response to load. The primary modes of loading taken into consideration are those associated with axial compression, flexion–extension bending, lateral bending, and axial torsion, as shown in Fig. 168-1.
Over time, as degeneration occurs, the disc becomes fibrotic and less able to dissipate and distribute loads. Consequently, nonphysiologic loads are then distributed to the vertebral endplates and the annulus of the disc, which may lead to morphologic endplate changes and annular fissuring. With the onset of the degenerative cascade, both the intervertebral disc and then facets becomes compromised. Currently, it is unclear whether the onset of anterior column degeneration leads to posterior degeneration, and vice versa. Regardless, the degeneration within the FSU may lead to the inability to withstand even physiologic loads, and eventually, depending on the severity, instability may develop. Both clinically and biomechanically, instability can be defined by the inability of the FSU to control physiologic displacement. With instability, the neurologic structures are prone to impingement and injury. Instability of the intervertebral discs shifts greater-than-normal motion and load to the facet joints and ligamentum flavum. With time, these structures all undergo hypertrophy with narrowing of the central neural canal, as well as of the lateral recesses and neural foramina.
Another design parameter that a potential manufacturer needs to address is that of the amount of stiffness required in an individual patient. Many in vitro biomechanical studies have demonstrated that the degree of inherent stiffness in the spine varies among individual specimens. Generally, older cadaveric spine specimens are more rigid than younger spines. Clinically, this has traditionally been assessed by the Kocher Clamp Test, described by Albert Key in 1944 (personal communication). Essentially, the surgeon grasps the posterior spinous processes and pulls. This provides a tactile indication of the spinal stiffness, which Key used to compare to prior experiences. A more objective measure of assessment was described by Brown et al., who utilized an FDA-approved intraoperative spinal stiffness gauge that measured force displacement (Spinal Stiffness Gauge, Mekanika, Boca Raton, FL)2 (Fig. 168-2). Their findings of 655 motion segments demonstrated wide variability in degrees of stiffness. Generally, the L5–S1 segment was stiffer than L4-5, which was stiffer than L3–4. Male patients had stiffer spinal segments than did female patients. Older patients had stiffer spinal segments than did younger patients. Finally, stiffness was found to decrease by 20% following decompression. These basic findings raise the issue of patient-specific requirements and the potential need to customize implants based on intraoperative findings.
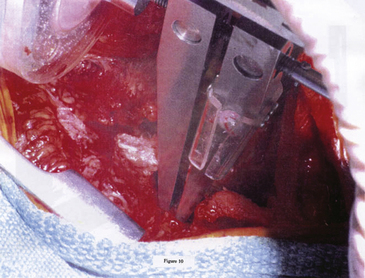
FIGURE 168-2 In vitro biomechanical testing probe placed between posterior spinous processes to determine spinal stiffness.
Another design concern is the amount of stability that an individual patient may require to achieve the best clinical outcome. That is, all other variables being equal, it remains unknown as to the required stiffness in an individual situation that will lead to pain relief and enough stabilization so as to prevent recurrence of symptoms and clinical (and/or potential radiographic) instability.
System Designs with Biomechanical and Clinical Outcomes
Complex biomechanical and clinical needs have been addressed by designers with varying degrees of success. As previously stated, all pedicel screw–based nonfusion stabilization devices are similar in that they anchor an implant that allows for some degree of mobility to pedicle screws. Perhaps the most basic design type was the Graf ligament. This system limited movement in flexion, and had good early clinical results, but ultimately it has not received larger clinical use.3
N-Hance Spine System
The N-Hance spine system is a unique system in that it comprises a solid titanium rod with a sliding component. The sliding component, which attaches to a pedicle screw, has polyurethane bumpers immediately above and below it. This system damps flexion and extension (biomechanically represented by an increase and decrease of the interpedicular distance) against the polyurethane stops (Fig. 168-3). The length of the fixed component of the rod can accommodate span multiple segments so that the system can be utilized for both rigid and nonrigid fixation. This combination of rigid stabilization (acting as a traditional pedicle screw fusion augmentation construct) and nonrigid stabilization (potentially utilized as a nonfusion spinal augmentation construct) is commonly described as a “hybrid” construct.4
Isobar TTL
The Isobar TTL system was initially released in Europe and was introduced in the United States about a decade ago. The system has been cleared by the FDA as an adjunct to fusion but has not been tested in a premarket approval study. This device, like other devices that permit movement, is suggested to be beneficial to fusion augmentation procedures by allowing movement and load sharing, to some degree, with the anterior column. The FDA approval for this device is as a fusion adjunct with anterior column support. The device allows small amounts of motion through a coupling joint incorporated into the interpedicular rod (Fig. 168-4).
The TTL system has been utilized as a nonfusion device. No larger outcome studies have been published describing its efficacy.
Dynesys
The system is elegant in its simplicity. It consists of three components: pedicle screws, a spacer, and a tensioning cord (Fig. 168-5). The pedicle screws are made of titanium alloy and have a textured surface that promotes bone on-growth. Recently, hydroxyapatite-coated screws were made available to further bone adhesion and promote ingrowth, potentially reducing screw-loosening concerns. The Dynesys screws have fixed heads and are self-tapping.
The specific biomechanical characteristics of the Dynesys implant are important to recognize, as well as the metrics used to describe the device. Traditional methods of comparing fixation implants for fusion procedures have relied on biomechanical characterization through the flexibility test protocol.5–7 The methodology of flexibility testing has been well described in the literature, originating with Panjabi’s early description of load input utilizing pure moments.8 The standardization of the pure-moment test protocol by Goel et al. has contributed to repeatability, despite biologic variability inherent to cadaveric testing.9 Advances in finite element modeling and the ability to determine the exact design effect on a generalized model provide additional important information.
Dynesys has been shown to provide immediate stabilization through cadaveric biomechanical testing.10 In that study, the ROM in pure-moment testing was used to assess and compare the performance of Dynesys and rigid rod fixation in providing immediate stabilization to an unstable FSU, referred to as the index level. With Dynesys instrumentation at the index level, L3-4, a mean ROM measurement of 1.28 ± 0.42 degrees in flexion–extension bending was reported. With rigid rod fixation, the mean ROM was 2.07 ± 1.21 degrees for the same instrumented L3-4 FSU. Dynesys, when characterized by a single-level FSU, the FSU appears biomechanically no different from the FSU in rigid rod fixation at the index level under pure-moment biomechanical testing parameters. In effect, when the treatment was limited to a single-level FSU, differences in the effects to the index level using the ROM metric alone were difficult to detect between rigid rod fixation treatments and Dynesys. This does not mean that differences do not exist but highlights the need for additional metrics to assess posterior, dynamic stabilization devices.
Other studies have discussed the difficulty of attributing additional disease to the adjacent level from prior rigid fixation treatments.11,12 Clearly, it can be shown that both Dynesys and a rigid rod construct provide immediate stability in flexion–extension at the instrumented levels. Dynesys provided increased stiffness in lateral bending but no increased stiffness on axial rotation. Biomechanically, immediate stabilization is important; however, long-term, posterior, dynamic stabilization devices are designed to continually withstand the repeated loading often characterized by bench-top fatigue cycling techniques.
The Dynesys system has been implanted worldwide in more than 40,000 patients. Most constructs performed outside of the United States have been applied for nonfusion, spinal stabilization of the lumbar spine. The indications for use have been highly varied, as have the clinical results. Reported uses have included treatment of spinal stenosis, degenerative lumbar instability, lumbar spondylosis, internal disc derangement, recurrent disc rupture, massive disc rupture, and adjacent-level degeneration prophylaxis. An early article by Dubois et al. reported its use in 57 patients with degenerative disc disease.13 These patients underwent decompression and nonfusion spinal stabilization. Mean follow-up was 13 months. Of the patients, 63% were pain free and 30% had mild pain. Also, 85% had a good or excellent results based on their MacNab scores.
Another study with long-term follow-up was published by Stoll et al. in 2002.11 The authors performed an examination of 83 patients who underwent decompression and Dynesys nonfusion stabilization for lumbar spinal stenosis, degenerative spondylolisthesis, and degenerative disc disease. The mean follow-up on these patients was 38 months. The authors demonstrated a statistically significant improvement in the Oswestry Disability Index (ODI) score and improvements in the visual analogue score (VAS) for lower back and leg pain. Putzier et al. compared 35 patients who underwent nucleotomy and Dynesys placement for disc rupture to 84 patients who underwent nucleotomy alone.14 Again, improvements were noted in VAS lower back and leg scores and in ODI outcomes at 34 months. Bordes-Monmeneu et al. showed similar results in 94 patients treated for lumbar spinal stenosis, degenerative disc disease, and disc herniation.15
A smaller study was performed on 26 patients treated for lumbar spinal stenosis and degenerative spondylolisthesis.16 These patients underwent stabilization with placement of the Dynesys system in all cases and decompression in some cases. The patients were followed for 2 years. This study showed significant improvements in VASs and walking distances but revealed that patients who underwent decompression and Dynesys fared better than patients who underwent Dynesys placement alone. Grob et al. had less positive results with application of the Dynesys system.17 In this study of 31 patients, 13 patients had Dynesys application alone and 18 underwent decompression with Dynesys supplementation. Results at 2-year follow-up showed that two thirds of the patients improved in VAS back and leg pain scores but only half of the patients said that the operation had helped and had improved their quality of life. Again, it was felt that Dynesys supplementation alone was inadequate and that decompression was a necessary to improve surgical outcomes.
As part of the FDA application process, the Dynesys system underwent a single-blinded, multicenter, prospective randomized, investigative device trial. The study group included patients 20 to 80 years of age with degenerative spondylolisthesis (anterolisthesis or retrolisthesis) up to grade I and a stenosing spinal lesion (neuroforaminal, lateral recess, or central stenosis). The patients had to experience greater leg symptoms than lower back symptoms. All patients entered into the study were felt, by the enrolling surgeon, to be candidates for single- or contiguous two-level lumbar fusion between L1 and S1. The minimum preoperative leg pain score was 40 mm on a 100-mm VAS,18 and an ODI version 219 score of 30 was required. Degenerative back pain more severe than the leg pain was the primary exclusion criterion. Patients who had pain not attributed to instability or stenosis, who had radiculopathic signs at more than two levels, or who had previously undergone lumbar fusion procedures, facetectomy, or trauma at the index level were also excluded.
We reviewed the preliminary (1 year) results on 101 patients in 2007.20 That review concentrated on the clinical results from six investigational device exemption (IDE) sites of the larger FDA IDE trial. The study included 53 women and 48 men with a mean age of 56 years. There were 43 prior surgeries in the group. Of the patients, 13% had secondary legal issues and 21% were smokers. Preoperative symptoms were present for an average of 5.3 years with mean ODI score of 56%. The mean VASs were 80 for leg pain and 54 for lower back pain.
With 80% follow-up at the 1-year postoperative period, there was a statistically significant reduction in VAS leg and lower back pain scores (26 and 29, respectively). There was also a statistically significant improvement in the mean ODI score to 26% (Fig. 168-6). Ten patients required further surgery for radiculopathy or increased lower back symptoms.
The complete FDA IDE study comprised 367 patients who were enrolled at one of 28 U.S. sites between March 2003 and June 2005. Ultimately, 253 patients received Dynesys, and 114 patients underwent pedicle screw–augmented PLF.
Surgical Techniques and Complication Management
Safe, accurate, and nonrevised placement of the pedicle screws is paramount for success of the nonfusion systems, because the surgeon is not relying on arthrodesis for stability. All efforts are made to maximize the bone–screw interface. Most surgeons use a pedicle probe to create the bony channel in the pedicle. This may be done under fluoroscopy for increased accuracy. The pedicle holes are created to maximize medial trajectory (Fig. 168-7). Tapping of the holes is to be avoided whenever possible. The largest and longest screw possible is placed into the pedicle to maximize the bone–screw interface. Furthermore, the depth of screw insertion is carefully observed so that the surgeon does not need to back the screw out (and subsequently reduce the screw–bone strength).
Once all screws are secured in the pedicles, the various systems are assembled per the manufacturer’s suggestions and the patient’s needs. Many systems require determination of the distance between pedicle screw heads, and this is easily determined with supplied measuring devices. Should distraction across the neuroforamina be desired, a slightly longer spacer or rod can be fashioned or selected. The system is secured to the pedicle screws, and the fusion bone, if desired, is placed. The wound is closed in the usual fashion. Lumbar bracing may be prescribed for 6 weeks to remind the patient to limit lumbar flexion and extension.
Bordes-Monmeneu M., Bordes-Garcia V., Rodrigo-Baeza F., et al. System of dynamic neutralization in the lumbar spine: experience on 94 cases. Neurocirugia (Astur). 2005;16:499-506.
Brown M.D., Holmes D.C., Heiner A.D., et al. Intraoperative measurement of lumbar spine motion segment stiffness. Spine. 2002;27:954-958.
Chapman C.R., Casey K.L., Dubner R., et al. Pain measurement: an overview. Pain. 1985;22:1-31.
Cheng B.C., Gordon J., Cheng J., et al. Immediate biomechanical effects of lumbar posterior dynamic stabilization above a circumferential fusion. Spine. 2007;32:2551-2557.
Dubois B., de Germay B., Schaerer N.S., et al. Dynamic neutralization: a new concept for restabilization of the spine. In: Szpalski M., Gunzburg R., Pope M.H., et al. Lumbar Segmental Instability. Philadelphia: Lippincott, Williams & Wilkins; 1999:233-240.
Fairbank J.C., Pynsent P.B. The Oswestry Disability Index. Spine (Phila Pa 1976). 2000;25:2940-2952.
Gardner A., Pande K.C. Graf ligamentoplasty: a 7-year follow-up. Eur Spine J. 2002;11:157-163.
Goel V.K., Panjabi M.M., Patwardhan A.G., et al. Test protocols for evaluation of spinal implants. J Bone Joint Surg Am. 2006;88(Suppl 2):103-109.
Grob D., Benini A., Junge A., et al. Clinical experience with the Dynesys semirigid fixation system for the lumbar spine: surgical and patient-oriented outcome in 50 cases after an average of 2 years. Spine (Phila Pa 1976). 2005;30:324-331.
Maserati M.B., Tormenti M.J., Panczykowski D.M., et al. The use of a hybrid dynamic stabilization and fusion system in the lumbar spine: preliminary experience. Neurosurg Focus. 2010;28:E2.
Mihara H., Onari K., Cheng B.C., et al. The biomechanical effects of spondylolysis and its treatment. Spine. 2003;28:235-238.
Nockels R.P. Dynamic stabilization in the surgical management of painful spinal disorders. Spine. 2005;30:S68-S72.
Oda I., Cunningham B.W., Abumi K., et al. The stability of reconstruction methods after thoracolumbar total spondylectomy. An in vitro investigation. Spine. 1999;24:1634-1638.
Panjabi M.M. Biomechanical evaluation of spinal fixation devices: I. A conceptual framework. Spine. 1988;13:1129-1134.
Patwardhan A.G., Carandang G., Ghanayem A.J., et al. Compressive preload improves the stability of anterior lumbar interbody fusion cage constructs. J Bone Joint Surg Am. 2003;85-A:1749-1756.
Putzier M., Schneider S.V., Funk J.F., et al. The surgical treatment of the lumbar disc prolapse: nucleotomy with additional transpedicular dynamic stabilization versus nucleotomy alone. Spine (Phila Pa 1976). 2005;30:E109-E114.
Schmoelz W., Huber J., Nydegger T., et al. Influence of a dynamic stabilisation system on load bearing of a bridged disc: an in vitro study of intradiscal pressure. Eur Spine J. 2006;15:1276-1285.
Schnake K.J., Schaeren S., Jeanneret B. Dynamic stabilization in addition to decompression for lumbar spinal stenosis with degenerative spondylolisthesis. Spine. 2006;31:442-449.
Stoll T.M., Dubois G., Schwarzenbach O. The dynamic neutralization system for the spine: a multi-center study of a novel non-fusion system. Eur Spine J. 2002;11(Suppl 2):S170-S178.
Welch W.C., Cheng B.C., Awad T.E., et al. Clinical outcomes of the Dynesys dynamic neutralization system: 1-year preliminary results. Neurosurg Focus. 2007;22:E8.
1. Nockels R.P. Dynamic stabilization in the surgical management of painful spinal disorders. Spine. 2005;30:S68-S72.
2. Brown M.D., Holmes D.C., Heiner A.D., et al. Intraoperative measurement of lumbar spine motion segment stiffness. Spine. 2002;27:954-958.
3. Gardner A., Pande K.C. Graf ligamentoplasty: a 7-year follow-up. Eur Spine J. 2002;11:157-163.
4. Maserati M.B., Tormenti M.J., Panczykowski D.M., et al. The use of a hybrid dynamic stabilization and fusion system in the lumbar spine: preliminary experience. Neurosurg Focus. 2010;28:E2.
5. Mihara H., Onari K., Cheng B.C., et al. The biomechanical effects of spondylolysis and its treatment. Spine. 2003;28:235-238.
6. Oda I., Cunningham B.W., Abumi K., et al. The stability of reconstruction methods after thoracolumbar total spondylectomy: an in vitro investigation. Spine. 1999;24:1634-1638.
7. Patwardhan A.G., Carandang G., Ghanayem A.J., et al. Compressive preload improves the stability of anterior lumbar interbody fusion cage constructs. J Bone Joint Surg Am. 2003;85:1749-1756.
8. Panjabi M.M. Biomechanical evaluation of spinal fixation devices. I. A conceptual framework. Spine. 1988;13:1129-1134.
9. Goel V.K., Panjabi M.M., Patwardhan A.G., et al. Test protocols for evaluation of spinal implants. J Bone Joint Surg Am. 2006;88(suppl 2):103-109.
10. Cheng B.C., Gordon J., Cheng J., et al. Immediate biomechanical effects of lumbar posterior dynamic stabilization above a circumferential fusion. Spine. 2007;32:2551-2557.
11. Stoll T.M., Dubois G., Schwarzenbach O. The dynamic neutralization system for the spine: a multi-center study of a novel non-fusion system. Eur Spine J. 2002;11(suppl 2):S170-S178.
12. Schmoelz W., Huber J., Nydegger T., et al. Influence of a dynamic stabilisation system on load bearing of a bridged disc: an in vitro study of intradiscal pressure. Eur Spine J. 2006;15:1276-1285.
13. Dubois B., de Germay B., Schaerer N.S., et al. Dynamic neutralization: a new concept for restabilization of the spine. In: Szpalski M., Gunzburg R., Pope M.H., et al. Lumbar Segmental Instability. Philadelphia: Lippincott, Williams & Wilkins, 1999. pp. ∗∗∗
14. Putzier M., Schneider S.V., Funk J.F., et al. The surgical treatment of the lumbar disc prolapse: nucleotomy with additional transpedicular dynamic stabilization versus nucleotomy alone. Spine (Phila Pa 1976). 2005;30:E109-E114.
15. Bordes-Monmeneu M., Bordes-Garcia V., Rodrigo-Baeza F., et al. System of dynamic neutralization in the lumbar spine: experience on 94 cases. Neurocirugia (Astur). 2005;16:499-506.
16. Schnake K.J., Schaeren S., Jeanneret B. Dynamic stabilization in addition to decompression for lumbar spinal stenosis with degenerative spondylolisthesis. Spine. 2006;31:442-449.
17. Grob D., Benini A., Junge A., et al. Clinical experience with the Dynesys semirigid fixation system for the lumbar spine: surgical and patient-oriented outcome in 50 cases after an average of 2 years. Spine (Phila Pa 1976). 2005;30:324-331.
18. Chapman C.R., Casey K.L., Dubner R., et al. Pain measurement: an overview. Pain. 1985;22:1-31.
19. Fairbank J.C., Pynsent P.B. The Oswestry Disability Index. Spine (Phila Pa 1976). 2000;25:2940-2952.
20. Welch W.C., Cheng B.C., Awad T.E., et al. Clinical outcomes of the Dynesys dynamic neutralization system: 1-year preliminary results. Neurosurg Focus. 2007;22:E8.