Chapter 4 Diathermy and lasers
Diathermy
Diathermy has been used in surgical procedures for over 100 years (d’Arsonval 1893) for cutting and coagulation of tissues. Harvey Cushing pioneered the use of electrosurgery in neurosurgery, using a generator designed by Bovie in the 1920s, and this name is still synonymous with diathermy to some surgeons.
Monopolar diathermy
In monopolar diathermy, one electrode is applied to the patient who becomes part of the circuit. The surface area of the electrode plate is much greater than the contact area of the diathermy instrument to ensure that heating effects are confined to the end of the active electrode (Figure 4.1). The advantage of monopolar diathermy is that it can be used to cut as well as to coagulate tissues.
Tissue effects of diathermy
When household electrical current of 50 Hz frequency passes through the body, it causes an irreversible depolarization of cell membranes. If the current is sufficiently large, depolarization of cardiac muscles will occur and death may result. If household current is modified to a higher frequency, above 200 Hz, depolarization does not occur; instead, ions are excited to produce a thermal effect. This is the basis of diathermy. Figure 4.2 illustrates how the frequency of a current influences the effects on the body.
Factors which influence the effect of diathermy
Cutting and coagulation
When the electrode is brought into direct contact with tissue and the waveform is modulated, coagulation occurs rather than vaporization. An intermittent waveform is used and thus bursts of thermal energy are interspersed with periods of no energy (Figure 4.3). For the power delivered to the tissue to remain constant, the electrosurgical unit must deliver a higher voltage to compensate for the episodes when no energy is delivered (up to 90% of the time with pure coagulation current). Thus, whilst cutting current at 50 W power will produce a high-frequency current of approximately 200–1000 V, a coagulation current may produce over 3000 V to deliver the same wattage to the tissue. The coagulating effect is produced by slower desiccation and shrinkage of adjacent tissue, producing haemostasis. The higher voltage produced by coagulation current carries a higher risk of inadvertent discharge of energy.
Duration of application
The longer the duration of application of electrosurgical current, the greater the extent of thermal injury. Research on uterine tissue shows that the duration of exposure of the tissue to current, rather than the wattage used, was the most important factor in producing tissue damage (Duffy et al 1991).
Heat and tissue injury
Diathermy current produces thermal injury to tissues. The temperature generated will dictate the degree of injury (Table 4.1). If carbon is seen on the tip of the diathermy electrode, the surgeon can assume that, at some stage, a temperature of 200°C has been reached.
Table 4.1 The degree of injury caused at different temperatures
Temperature (°C) | Tissue effect |
---|---|
44 | Necrosis |
70 | Coagulation |
90 | Desiccation |
200 | Carbonization |
Short-wave diathermy
Electrode redesign has led to an interest in the use of short-wave diathermy for its tissue destructive effect (Phipps et al 1990). It has been used in endometrial ablation. In this, the two electrodes form a capacitor in the output circuit with the patient providing the dielectric medium between the two plates. Frequencies of approximately 27 MHz are employed with power levels of approximately 500 W. By altering the shape and size of the electrodes, heating effects may be localized or diffused as required. However, care must be exercised as the effects are not always predictable.
Lasers
A laser is a device capable of producing near-parallel beams of monochromatic light, either visible or invisible, at controlled intensities. This light can be focused, thus concentrating its energy, so that it can be utilized to treat various conditions. The term ‘laser’ is an acronym for ‘Light Amplification by the Stimulated Emission of Radiation’. The process of stimulated emission was foreshadowed by Einstein at the turn of the century, but it was not until 1960 that the first optical device was constructed (Maiman 1960). Since that time, many lasers have been made but comparatively few have found their way into gynaecological practice.
Basic laser physics
A laser consists of three main elements: a power supply, an excitable medium and an optical resonator (Figure 4.4). Atoms or molecules within the medium are raised to high-energy states (Figure 4.5A) by the power supply, and, under normal circumstances, these would decay to the ground state by the emission of energy as photons (Figure 4.5B). By confining the process to an optical cavity and restricting the decay paths, stimulated emission (Figure 4.5C) can take place. This process produces a build-up of photons (light) at a particular wavelength inside the cavity. The laser output is a small fraction of this which is allowed to escape from one end of the cavity.
Many substances have been found to be suitable laser media — solids, liquids, gases or metallic vapours — but the basic principles remain the same. A more detailed explanation of laser physics is provided elsewhere (Carruth and McKenzie 1986).
The radiation emitted is monochromatic (if only one decay path is involved), coherent and collimated. Collimation, or the near-parallel nature of laser light, can be exploited in many ways and is the main feature which makes such devices useful in the medical world. A single convex lens placed in the beam will bring it to a sharp focus, the size of which is dependent upon the width of the collimated beam. The use of different lenses or varying the lens-to-tissue distance alters the diameter of the beam at the point of contact with the tissue (Figure 4.6). This is referred to as changing the spot size.
Light–tissue interaction
Light impinging on tissue is subject to the normal laws of physics. Some of the light is reflected and some is transmitted through the air–tissue barrier and passes into the tissue where it is scattered or absorbed. Obviously the extent to which each process dominates is dependent upon the physical properties of the light and the tissue. The theory of light–tissue interaction is not as well developed as that of ionizing radiation (Wall et al 1988), but enough is known to explain the macroeffects upon which most laser treatments depend.
At very-low-energy density levels (power density × time), say below 4 J/cm2, a stimulating effect on cells has been observed, but above this level, the effect is reversed and suppression occurs (Mester et al 1968). As the energy density rises to 40 J/cm2, indirect cell damage can take place if any sensitizing agents present become activated (e.g. haematoporphyrin derivative). Direct tissue damage does not take place until approximately 400 J/cm2, when the first thermal effects appear and photocoagulation occurs. Another 10-fold increase in energy density results in complete tissue destruction as it is sufficient to raise the cell temperature rapidly to 100°C, causing tissue vaporization. Obviously these are general observations and other properties of the incident beam will have an influence, but the general 10-fold relationship alluded to above holds although other parameters may be varied. Amongst the most important of these are the wavelength and the pulsatile nature of the radiation involved.
The wavelength absorption characteristics of various body tissues are reasonably well understood, qualitatively if not quantitatively. Figure 4.7 shows the absorption curves for water, melanin and haemoglobin which, to a large extent, will determine the curves for tissue as a whole. In the ultraviolet and the middle-to-far infrared spectrum, absorption by water predominates whereas melanin and haemoglobin effects take over in the visible range. From this graph, it is easy to see that a particular laser, operating at a fixed wavelength, will be preferentially absorbed by one tissue constituent and its effects will be different from those of another laser with a different wavelength.
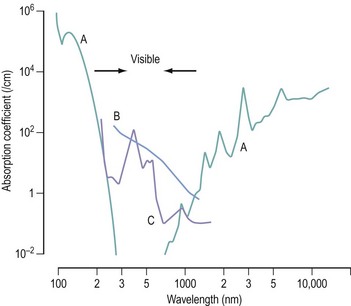
Figure 4.7 Absorption characteristics of (A) water, (B) melanin and (C) haemoglobin at different wavelengths.
Source: With kind permission from Springer Science+Business Media: Lasers in Medical Science, Photophysical proceses in recent medical laser developments: a review, 1:47–66, 1986, Boulnois J.
So far, it is implied that the laser is operated continuously for long enough for thermal effects to appear [continuous wave (CW) mode]. However, it is relatively simple, by means of a shutter or a controllable power supply, to switch the energy on and off rapidly (pulse mode). In general, the medical definition of a pulse is a burst of energy lasting 0.25 s or less. This does not correspond to the physics definition of a pulse, and care must be taken to avoid confusion. Methods of generating these pulses differ between lasers and the tissue effects can also vary. The reason for this phenomenon is shown in Figure 4.8.
Figure 4.8A shows the output from a CW laser which is modulated by a perfect shutter. The average power delivered to the tissue can be calculated from a knowledge of the CW power, the pulse repetition rate and width. In the case of an electronically controlled high-pulse power system where the time–power curve is not so closely defined (Figure 4.8B), it is much more difficult to determine the average power delivered. It is necessary to measure the energy of the pulse and the pulse repetition rate. The peak power, although impressive, is almost irrelevant.
Very short pulses, such as those produced by Q-switched lasers, can cause electromechanical breakdown in tissues because of the extremely high energy densities obtainable. In the early days, these lasers were tested in gynaecology but untoward side-effects were noted (Minton et al 1965).
Common laser systems
Two laser systems established themselves in gynaecological practice during the 1980s:
Carbon dioxide laser
This is the system which has been most used in gynaecology since it was used for the first time in 1973 for the treatment of cervical erosions. This has been because of its clinical stability, ease of use, reliability and easy serviceability. It is a precise laser, especially in ultrapulse mode, making it useful for division of adhesions and accurate and safe vaporization of deposits of endometriosis (Sutton 1993). Although a major drawback is that its output cannot be transmitted by fibres, it has so far not been totally displaced by other lasers but is rivalled by modern diathermy equipment.
The final delivery to the operation site can be carried out in two ways, both incorporating a focusing lens. For hand-held surgery, the lens at the end of a straight delivery tube focuses the beam on the operative field approximately 1 cm from the end of the tube. The lens-to-tissue distance can thus be varied at will so that the spot size will be changed within a narrow range (Figure 4.9A). Colposcopic delivery involves a mirror after the focusing lens which brings the beam into line with the viewing axis of the colposcope. This mirror is at the end of a joystick so that the beam may be moved around within the field of view (Figure 4.9B). Originally the spot size was changed using lenses of different focal lengths and so was limited to three or four predetermined sizes. Nowadays, the use of zoom optics allows infinite adjustment over a limited range (0.5–4 mm).
Neodymium:yttrium aluminium garnet laser
The Nd:YAG laser has a greater depth of penetration and is more suited to hysteroscopic surgery (Sutton 1993). Hysteroscopic procedures include removal of fibroids and polyps, transsection of uterine septae, lysis of adhesions and endometrial ablation.
Other laser systems
Photodynamic therapy
Photodynamic therapy is a treatment for certain tumours (Spikes and Jori 1987). It relies upon selective retention in tumour tissues of a drug (photosensitizer), such as haematoporphyrin derivative, which only becomes active on exposure to light of a particular wavelength. This provides highly selective tissue necrosis. Each photosensitizer has a particular spectrum of action requiring light of the appropriate wavelength for maximum absorption and effect. Clinically used sensitizers work between 420 nm (blue) and 780 nm (deep red). Longer wavelengths penetrate deeper (blue 1–2 mm and red >5 mm). As there are many decay pathways available, the output is multi-wavelength, but a narrow wavelength range can be selected with suitable optical devices. The copper vapour and dye laser combination can produce over 5 W of red light at 630 mm, which is an absorption peak of haematoporphyrin derivative.
Argon laser
The argon laser, producing light at 488 nm (blue) and 514.5 nm (blue/green), is particularly favoured by ophthalmologists. The green output of the KTP laser (potassium titanyl-phosphate, 532 nm) has been more useful in gynaecology. The output is derived by taking the Nd:YAG beam and frequency and doubling it in a KTP crystal, thus providing the surgeon with a choice of wavelengths (or even a mixture of the two). The advantages of the light lasers, argon and KTP lasers, over the CO2 laser is their selective absorption by haemoglobin, less smoke plume production and an easy delivery system that uses lower power settings. They have been considered to be more suitable for treatment of ovarian endometriomas and ectopic pregnancies, as the CO2 laser energy is strongly absorbed by the water molecule and is rendered ineffective in the presence of blood (Sutton 1993). The main disadvantage of the light lasers is the need to wear special protective glasses which can distort the view of the pelvis.
Ultraviolet lasers
The early use of lasers in gynaecology was in the treatment of cervical intraepithelial neoplasia, but subsequently spread to most other areas of the lower reproductive tract. The use of the laser in treatment of intraepithelial neoplasia of the genital tract is described in Chapter 38. Similar diathermy techniques are described in the same chapter.
We are under pressure to decrease health costs, so alternative, less-expensive technology has reduced the use of laser technology in recent years. However, lasers are still used in various clinical settings in obstetric and gynaecological surgery. Some examples include intrauterine laser ablation of placental vessels for the treatment of twin–twin transfusion syndrome (Robyr et al 2005), and ovarian drilling for ovulation induction in patients with anovulatory polycystic ovary syndrome (Farquhar et al 2007). Other uses are being investigated, but the evidence on safety and efficacy outcomes thus far is insufficient to support use in a non-research environment. Examples of these include laparoscopic laser myomectomy and percutaneous laser therapy for fetal tumours. There are also controversial areas regarding the use of lasers which have entered commercial gynaecology without much regulation or scientific evaluation, such as laser vaginal rejuvenation.
Laser safety
All lasers sold in the UK must conform to three basic standards. Electrical safety requirements are detailed in BS EN 60601-1-1 (British Standards Institution 2001) and non-ionizing radiation hazards are covered by BS EN 60825-1 and BS EN 60601-2-22 (British Standards Institution 1996, 2007). A further publication by the Medicines and Healthcare Products Regulatory Agency (2008) outlines good laser practice together with some additional equipment safety features. All these publications should be considered by a laser protection adviser (a health authority or trust appointee) who will assist each user in specifying and installing lasers in clinical surroundings. Although the requirements will vary from laser to laser and from site to site, the following sections outline the major considerations involved.
Control of hazards
Administrative controls are framed to ensure that the equipment is then used safely. A set of local rules must be drawn up which specify who is allowed to use a laser and where it can be used. A laser controlled area must be defined and the activities within that area must be controlled. There must be no possibility of radiation in excess of the maximum permitted exposure level passing out of the area, even if this means blocking windows and doors. Warning signs must be exhibited at the entrance to the laser controlled area, and door interlocks can be used but are not obligatory. Examples of such local rules can be found in other texts (Stamp 1983, Medicines and Healthcare Products Regulatory Agency 2008).
Fire is an ever-present hazard with the use of Class 4 lasers and simple precautions must be observed. Aqueous instead of spirit-based solutions should be used and paper drapes should be avoided. CO2 radiation is readily absorbed by water, and by keeping swabs soaked in water or saline, tissues adjacent to the operation site can be protected. A suitable fire extinguisher should always be available. Greater care must be exercised in the presence of inflammable anaesthetic gases. Endotracheal tubes have been ignited by a laser with serious results (Bandle and Holyoak 1987). While this situation is not likely to be encountered in gynaecology, inflammable gases may be passed rectally by the patient during laser treatment of the lower genital tract, posing a theoretical risk of explosion.
A by-product of laser treatment (and diathermy to a certain extent) is a plume of smoke and debris, with a characteristic odour. This must be evacuated and collected from as near as possible to the impact zone for two important reasons. First, the emission of smoke is likely to obscure the operating site. This is particularly true in cervical or vaginal surgery. Second, doubts have been raised over the viability of particles contained within the plume (Garden et al 1988). Although the evidence is not overwhelming, it is essential that the smoke should be adequately extracted and filtered.
Conclusions
KEY POINTS
Bandle AM, Holyoak B. Laser incidents. In: Mosely H, Haywood JK, editors. Medical Laser Safety. London: Institute of Physical Sciences in Medicine; 1987:47-57.
Boulnois J. Photophysical processes in recent medical laser developments: a review. Lasers in Medical Science. 1986;1:47-66.
British Standards Institution. Medical Electrical Equipment. Particular Requirements for Safety. Specification for Diagnostic and Therapeutic Laser Equipment. BS EN 60601-2-22. London: BSI; 1996.
British Standards Institution. Medical Electrical Equipment. Collateral Standard. Safety Requirements for Medical Electrical Systems. BS EN 60601-1-1. London: BSI; 2001.
British Standards Institution. Safety of Laser Products. Equipment Classification and Requirements. BS EN 60825-60821. London: BSI; 2007.
Carruth JAS, McKenzie AL. Medical Lasers, Science and Clinical Practice. Bristol: Adam Hilger; 1986.
d’Arsonval MA. Production de courants de haute frequence et de grande intensite: leurs effects physiologiques. Comptes Rendus Societe de Biologie. 1893;9:122-124.
Duffy S, Reid PC, Smith JHF, Sharp F. In vitro studies of uterine electrosurgery. Obstetrics and Gynaecology. 1991;78:213-220.
Farquhar C, Lilford RJ, Marjoribanks J, Vandekerckhove P 2007 Laparoscopic ‘drilling’ by diathermy or laser for ovulation induction in anovulatory polycystic ovary syndrome. Cochrane Database of Systematic Reviews 18: CD001122.
Garden JM, O’Banion MK, Shelnitz LS, et al. Papillomavirus in the vapour of carbon dioxide laser treated verrucae. JAMA: the Journal of the American Medical Association. 1988;259:1199-1202.
Maiman TH. Stimulated optical radiation in the ruby. Nature. 1960;187:493-494.
Medicines and Healthcare Products Regulatory Agency 2008 Guidance on the Safe Use of Lasers, IPL Systems and LEDs. MRHA, London.
Mester E, Ludany G, Vajda J, et al. Uber die Wirkung von Laser-Strahlen auf die Bakteriemphagozytose der Leukozyten. Acta Biologica et Medica Germanica. 1968;21:317-321.
Minton JP, Carlton DM, Dearman JR, et al. An evaluation of the physical response of malignant tumour implants to pulsed laser radiation. Surgery, Gynaecology and Obstetrics. 1965;121:538-544.
Phipps JH, Lewis BV, Roberts T, et al. Treatment of functional menorrhagia by radiofrequency-induced thermal endometrial ablation. The Lancet. 1990;335:374-376.
Robyr R, Quarello E, Ville Y. Management of fetofetal transfusion syndrome. Prenatal Diagnosis. 2005;25:795.
Spikes JD, Jori G. Photodynamic therapy of tumours and other diseases using porphyrins. Lasers in Medical Science. 1987;2:3-15.
Stamp JM. An introduction to medical lasers. Clinical Physics and Physiological Measurement. 1983;4:267-290.
Sutton C. Lasers in infertility. Human Reproduction. 1993;8:133-146.
Wall BF, Harrison RM, Spiers FN. Patient Dosimetry Techniques in Diagnostic Radiology. York: Institute of Physical Sciences in Medicine; 1988.