CHAPTER 69 Deep Brain Stimulation for Epilepsy
Electrical stimulation of the nervous system has a very long history. Legend holds that a freed slave of Emperor Tiberius in 15 AD accidentally stepped on a torpedo electric fish. After recovering from the shock, he noted improvement in his gout. A local physician consequently recommended torpedo fish treatment of pain.1,2 From early times, electrical stimulation therapies were prone to adoption by charlatans. The grand electrical machine of Francis Lowndes produced static electricity with impressive effects on the subject’s hairstyle and purported benefits for a wide list of maladies.3 In the 1700s, a Leyden jar, an early form of a battery, was used to generate sparks claimed to be beneficial for paralysis, blindness, warts, toothache, St. Vitus’ chorea, and death by drowning. A theatrical high point of DBS was accomplished by Dr. José Delgado, who stopped a charging bull with handheld radio control of brain electrodes implanted in the animal’s internal capsule and basal ganglia.
The first legitimate scientific use of electrical brain stimulation in a live patient was probably performed by Dr. Roberts Bartholow.2 The patient had an infected scalp ulcer and osteomyelitis. Débridement led to exposure of the brain. During the procedure, Dr. Bartholow stimulated the exposed cortex with a sporadic current source and noted muscle contractions. Intraoperative electrical brain stimulation came into mainstream medicine through the efforts of the neurosurgeon Wilder Penfield and the neurologist Herbert Jasper at the Montréal Neurological Institute in the 1940s and 1950s. Their use of stimulation was diagnostic rather than explicitly therapeutic, and the information gained from electrical stimulation mapping was used to aid tailored resections of the brain for lesions and seizures.
Many innovators used depth wires to record deep in the brain in the late 1940s and early 1950s, but therapeutic stimulation via implanted wires was probably first performed by Heath in the early 1950s, with stimulation of the posterior frontal/septal region being used to alleviate pain and psychosis. In a 1963 article, R. G. Heath refers to work begun in 1952 with electrodes implanted into the centromedian nucleus, septal region, and mesencephalic tegmentum in a patient with psychomotor epilepsy.4 Stimulation was set at 100 Hz, 3 to 5 mA for 15 minutes and could be triggered by the patient. A variety of subjective symptoms were produced by stimulation, some of which were sufficiently positive that patients would initiate stimulation up to 300 times per hour. At one point the patient’s acute psychotic symptoms were interrupted with septal stimulation.
Cerebellar Stimulation
Irving Cooper, working from Valhalla, New York, pioneered the use of DBS as chronic therapy for epilepsy.5 His first therapeutic target of DBS for epilepsy was the cerebellum based on previous animal work. Sherrington showed in 1897 that the cerebellar cortex provoked inhibitory activity in various structures. In 1941, Moruzzi demonstrated that motor twitches in cats from strychnine on the cortex could be attenuated by cerebellar stimulation.6 In 1955, Cooke and Snider demonstrated that 60-Hz stimulation of the cerebellar cortex could modify seizures provoked by electrical stimulation of the cat cerebral cortex.7 Cooper and colleagues used a silicon mesh with four to eight pairs of bipolar platinum electrodes applied to the midline cerebellum or neocerebellum to stimulate the cerebellar cortex chronically in patients.8 Electrodes were stimulated through a subcutaneous antenna on the chest by inductive coupling through the skin. Their 1973 article reported cerebellar stimulation in seven patients with intractable seizures, three with psychomotor seizures, three with grand mal seizures, two with petit mal seizures, and one with focal seizures involving the left side of the face, arm, and leg. Six of the seven were said to improve over a period of 8 months, and virtually complete control of seizures was achieved in four of them.
The original article by Cooper and coworkers in 1973 stimulated a small series of cerebellar implantation in people with epilepsy. As summarized by Krauss and Fisher, 11 uncontrolled studies showed benefit of cerebellar stimulation.9 Two blinded controlled studies, one by Wright and colleagures10 and the other by Van Buren and associates,11 were, however, negative. The Wright study was performed in 12 patients with treatment lasting 6 months. The Van Buren study included 5 patients. Neither showed efficacy over sham stimulation. Even though these two controlled studies were criticized methodologically and totaled just 17 patients, they served to place cerebellar stimulation for epilepsy on the back burner for many years. Certain practitioners, notably the neurosurgeon Ross Davis, continued arguments that cerebellar stimulation could be effective in selected people with epilepsy.12–16 At a mean follow-up of 17 years, 19 patients with cerebellar stimulators were contacted by Davis, and 53% indicated that that they were seizure free and 32% had reduced seizures.12 Generalized tonic-clonic seizures appeared to show the best response.
Velasco and colleagues performed a small double-blind, randomized trial of bilateral stimulation of the superomedial surface of the cerebellum in five patients with refractory motor seizures.17 The parameters of the stimulation consisted of 10 pulses per second, 4 minutes on and 4 minutes off adjusted to a current density of 2 microcoulombs (µC) per square centimeter per pulse. Statistical analysis indicated a beneficial effect of stimulation. The three patients who completed the protocol for 2 years showed a reduction in generalized tonic-clonic seizures to a mean of 24% of baseline levels. Definitive documentation of the efficacy of cerebellar stimulation will probably be provided only by a large, controlled clinical trial.
Stimulation Sites for Treating Epilepsy
Figure 69-1 illustrates various regions of the nervous system that have been stimulated in humans to treat seizures. Direct cortical stimulation is discussed separately in this text. Exemplary studies at the different sites are tabulated in Table 69-1. No attempt is made to list all DBS studies in the literature. Only stimulation of the peripheral vagus nerve is licensed in the United States for epilepsy therapy. Vagus nerve stimulation is not discussed in this chapter.
Brainstem
The brainstem is the source of the reticular activating system classically described by Moruzzi and Magoun.6 Activation of the reticular activating system would be expected to result in electroencephalographic (EEG) desynchronization and make the formation of seizures, which consist of highly synchronous rhythms, more difficult. Only two studies have evaluated brainstem stimulation for epilepsy in patients. In these studies,19,20 the locus caeruleus was stimulated in a total of three patients. Benefit was reported in the form of a reduction in seizures and prolongation of auras, but the benefit was mild and sustained for only a few days.
Posterior Hypothalamus
The mammillary bodies of the posterior hypothalamus are on the classic circuit of Papez, which links hippocampal outflow to the mammillary bodies and anterior thalamus, to the cingulate, and then back to the entorhinal cortex and hippocampus. Interruption of the mammillothalamic tract prevents pentylenetetrazol-induced seizures in guinea pigs.55 Stimulation of the mammillary nuclei at high frequency with depth electrodes similarly increases the pentylenetetrazol seizure threshold in rats.56 Activation of the histamine system mediated by the mammillary nucleus was hypothesized to be involved in the mechanism of action of mammillary body DBS.57 With these experimental data as background, van Rijckevorsel and colleagues in Brussels implanted DBS electrodes in three patients with refractory epilepsy.21 They observed paroxysmal epileptiform discharges in the mammillary bodies. Although potentially beneficial for seizures, stimulation and further implantations were not continued because of the potential risk for hemorrhage.
Corpus Callosum
Marino Junior and Gronich in São Paulo implanted DBS electrodes in 10 patients with disabling convulsive disorders.22 Preliminary evaluation suggested that such implantation was better tolerated than corpus callosotomy and possibly beneficial against the seizures.
Caudate
A long history of animal experimentation suggests the possible utility of DBS in the caudate for controlling seizures. The caudate has been shown to exert inhibitory control over propagation of seizures. In 1982, Oakley and Ojemann showed that caudate stimulation attenuated seizures produced by placing alumina cream on the cortex of monkeys.58 After continuous caudate stimulation, five of the six monkeys exhibited a rebound in increased seizure activity. Psatta showed that caudate stimulation at 5 Hz, 0.3 msec, 1- to 5-V pulses for 1 to 3 seconds reduced interictal spiking in freely moving cats made epileptic by cobalt on the neocortex.59 La Grutta and Sabatino placed bipolar stimulating electrodes bilaterally in the caudate of cats, followed by hippocampal injection of penicillin. Hippocampal spiking decreased by 55% with stimulation.60
Clinical application of caudate stimulation was first performed by Sramka and associates. In a study of various DBS sites for epilepsy, six patients underwent unilateral or bilateral caudate stimulation. Over a period of 4 to 6 days of treatment, two were said to be without seizures and four improved.23 Further studies by Sramka and the Chkhenkelis of low-frequency (4 to 8 Hz) stimulation of the head of the caudate reduced the frequency of mesial temporal neocortical seizures, often to 10% to 30% of baseline levels.24–26
Centromedian Thalamus
The centromedian thalamus (CM) is part of the so-called nonspecific thalamic activating system that interacts with diffuse regions of the cortex and, in the case of the CM, also with the basal ganglia and brainstem. In 1987, the Velasco brothers and colleagues implanted electrodes in five patients with multifocal or primary generalized refractory seizures.27 Stimulation parameters were bipolar square pulses 0.1 msec in duration, 60 to 100 Hz, and 0.8 to 2.0 mA in trains of 1 minute every 5 minutes, alternating on the right and left side for 2 hours daily. The stimulator wires were external. Generalized tonic-clonic seizures were reduced 80% to 100% and complex partial seizures 60% to 100%. One patient with myoclonic seizures had the seizures abolished completely with stimulation.
Fisher and associates tested this protocol in a double-blind, randomized crossover trial in seven patients.28 Stimulation was on or off for 3 months, then off in all patients for a 3-month “wash-out,” and then off or on as the opposite treatment of the first 3 months for an additional 3 months. Patients could not tell whether the stimulator was on or off. Overall seizure frequency improved 30% but the difference was not statistically significant. The patient with the greatest improvement could not be analyzed because the family did not wish the stimulator settings to be changed from the apparently beneficial effects in the first 3 months (stimulator on). The degree of carryover effect from stimulation in the first cycle could not be estimated but may have influenced the frequency of seizures during the control months. Subsequently, Velasco and colleagues implanted deep brain stimulators into the centromedian nucleus in 50 to 100 patients.30–33,61,62 Further experience suggested that generalized tonic-clonic seizures improved the most and atypical absence seizures in part, but complex partial seizures and focal EEG spiking improved little. Patients with the otherwise highly intractable Lennox-Gastaut syndrome had up to an 80% reduction in seizures. The only controlled study of CM stimulation has been that of Fisher and associates,28 and no definitive large randomized trial has been performed.
Hippocampus
Most brain regions subjected to DBS for seizures are synaptically connected to but remote from the seizure focus or foci. Hippocampal stimulation provides an exception to this rule in that the hippocampus is often the primary site of the seizure focus. Direct hippocampal stimulation in humans to treat epilepsy was again pioneered by the Velasco brothers. In 2000, Velasco and colleagues published a study of 16 patients with complex partial and secondarily generalized tonic-clonic seizures.34 Hippocampal stimulation was delivered at high frequency, low amplitude continuously with 130 pulses per second, 450-µsec pulse duration, and an amplitude of 200 to 400 µA. In 7 patients, seizures were abolished after 6 days of stimulation. Long-term follow-up by Velasco and associates of 9 patients for 18 months to 7 years showed that the reduction in seizures was greater than 95% with hippocampal stimulation, provided that the baseline MRI findings were normal, versus a 50% to 70% reduction in the presence of mesial temporal sclerosis.41
Vonck, Boon, and colleagues extended the findings of Velasco for hippocampal stimulation.37,38 In an initial study of 3 patients with complex partial seizures, all had greater than 50% improvement in their seizures. In a later study of 7 patients implanted with bilateral hippocampal electrodes, seizures were reduced by half or better. Boon and coworkers evaluated 10 patients with long-term hippocampal stimulation: 1 became seizure free, 1 had a 90% reduction, 5 had at least a 50% reduction, 2 had a 30% to 49% reduction, and 1 was not helped.42 A paradigm of seizure detection and hippocampal stimulation in response was used by Osorio and colleagues in 4 patients.39 With this paradigm, seizures were reduced by 56%. A small, randomized crossover trial involving 4 patients was performed by Tellez-Zenteno and associates.40 Left hippocampal stimulation at 190 Hz was on for a month and off for a month or in the reverse order. Seizure frequency decreased a median of 15%, which was not statistically significant. No large, randomized trial of hippocampal stimulation has been completed as of the time of this writing, but such a trial is under development.
Subthalamic Nucleus
The subthalamic nucleus (STN) is a target for the treatment of certain movement disorders.63 In 1998, Vercueil, Benabid, and colleagues demonstrated that high-frequency stimulation of the STN suppressed absence seizures in a genetic absence seizure strain of rats.64 In the clinic of Benabid, this was carried forward to patients.43 DBS electrodes were inserted bilaterally into the STN of five patients. Stimulation was mostly continuous at 130 pulses per second, a duration of 90 µsec, and an amplitude 1.5 to 5.2 V. Mean reductions in seizure frequency for each patient were 81%, 42%, 68%, 67%, and 0%. Neme and colleagues implanted electrodes in the STN in four patients with partial epilepsy.45 Two of the patients had a reduction in seizures in the range of 42% to 75%. No benefit occurred in the other two. Handforth and coworkers showed a 33% to 50% reduction during the time of STN stimulation in two patients with partial seizures.46 Vesper reported 50% improvement with STN stimulation in a patient with progressive myoclonic epilepsy.47
Anterior Nucleus of the Thalamus
The anterior nucleus of the thalamus (AN) was an original target of Cooper in his early studies of DBS for epilepsy. His rationale for implanting in this particular nucleus was not clearly expressed in his publications, except that it was considered to be part of the nonspecific thalamus. The AN can influence EEG activity in regions of the frontal cortex. Cooper, Upton, and associates published several case series reporting the beneficial effects of AN stimulation on seizures,65–68 but the results were not quantifiable from the publications. In 1988, a group from the Medical College of Pennsylvania published two abstracts on 5 patients who underwent AN stimulation.49 Seizures were reduced to 23% of baseline. A pilot study was then initiated by Fisher and colleagues to develop parameters and methodology for a controlled trial of AN stimulation.28 The results in the 10 patients in the pilot study were published in two separate reports. The group from Toronto reported on 5 of the patients.50 Seizures were reduced to a mean of 47% of baseline. Interestingly, benefit appeared to occur mainly after implantation, before turning on the stimulator. The second cohort of 5 different patients in the pilot trial was reported from the Barrow Neurological Institute and the University of Pennsylvania.51 Seizures were reduced to 33% of baseline. There appeared to be particular benefit against seizures of a type that could produce falls and injuries. In South Korea, Lee and associates showed seizures to be reduced to 44% of baseline with AN stimulation in 6 patients.52 Lim and colleagues in Taiwan showed a reduction to 49% of baseline in 4 patients.53 Osorio and colleagues in Kansas reported a reduction to 25% of baseline in 4 patients with AN stimulation.39 A per-patient analysis of the six studies demonstrated a mean reduction in seizures to 47% of baseline levels at approximately 12 months after implantation.
Recently, a multicenter, randomized, double-blind parallel-group study of AN stimulation for epilepsy was published.54a,69 The study, sponsored by Medtronic, is abbreviated SANTE for “stimulation of the anterior nucleus of the thalamus for epilepsy.” The protocol admitted patients with partial and secondarily generalized seizures at a frequency of six or more per month and refractory to at least three antiepileptic drugs. Patients could not be candidates for conventional resective surgery. Stimulators were implanted bilaterally in the AN and connected to a Medtronic (Santa Rosa, CA) model 7428 Kinetra neurostimulator using DBS model 3387 leads. A 3-month baseline seizure frequency was recorded before implantation of the stimulator, and randomization was performed 1 month later. Patients randomized to the treatment group received 5-V stimulation at 145 pulses per second, 90-µsec pulse duration, on for 11 minutes and off for 5 minutes, synchronously to the left and right. Those randomized to the control group underwent stimulation with the same settings, but at 0 V. After a 3-month double-blind phase, the stimulation parameters were set open-label to 5 V in all patients and then allowed to vary systematically. A total of 158 patients were entered into the study and 110 underwent implantation. During the 3-month blinded phase, the stimulated group experienced a 40.5% median reduction in seizures compared with baseline versus a 14.5% reduction in the control group (p = 0.038). Improvement was maintained during a 2-year open-label phase. No symptomatic hemorrhages or deaths attributable to stimulation occurred. Stimulated subjects reported more adverse events linked to depression or memory problems.
Complications
Complications of DBS can be categorized as tissue reactions, hemorrhage, abnormal sensations, memory impairment, infection, or an adverse impact on seizures. Tissue reactions to chronic cerebellar stimulation in monkeys were examined by Brown and coworkers.70 After 2 months of stimulation of the cerebellum, light and electron microscopic analysis was performed on the monkey brains. The electrode array produced meningeal thickening and loss of Purkinje cells. An estimated charge density of 7.4 µC/cm2 per pulse, which is approximately 5 times the usual clinical intensity, did not result in any morphologic changes in tissue. In contrast, Henderson and coauthors reported a case in which an electrode migrated from the thalamic ventral intermediate nucleus to the centromedian nucleus and produced cell loss in that region.71 Cell loss in the cerebellum may not always be a consequence of neurostimulation. Salcman and colleagues showed significant Purkinje cell loss in five patients in whom the cerebellar cortex was biopsied at the time of electrode implantation.72 Very little information is known about tissue loss as a result of thalamic stimulation to treat epilepsy. One patient treated by AN stimulation for seizures died of “sudden unexplained death in epilepsy” 8 months after implantation. Examination by microscopy and immunohistochemistry showed no significant changes other than mild inflammation along the electrode track.73
Hemorrhage is perhaps the most feared complication of DBS. It usually occurs at the time of electrode implantation or immediately thereafter. Improvements in imaging technology, surgical technique, and the flexibility of electrodes have reduced the likelihood of a hemorrhagic complication. In addition, it is important to note that not all implantation-related hemorrhage is symptomatic; some hemorrhages are detectable only with neuroimaging. Little information is available about the risk for hemorrhage with implantation in the hippocampus, CM, or AN for epilepsy. One patient in an early trial of AN stimulation suffered a symptomatic hemorrhage resulting in prolonged hemiparesis, although that result was not documented in a scientific publication. Much more information is known about the complications of DBS for treating movement disorders because the number of patients is much larger and the experience over time much greater. In the pivotal trial of STN stimulation for Parkinson’s disease reported in the New England Journal of Medicine by the Deep-Brain Stimulation for Parkinson’s Disease Study Group, 3 of 102 (3%) patients experienced intracranial hemorrhage related to the implantation procedure.74 Hemiparesis as a result of the hemorrhage developed in all 3. Three patients had seizures and 4 had infections. Beric and colleagues reported the complications of DBS for Parkinson’s disease, tremor, and dystonia after 149 implants in 86 patients.75 Two patients (2.3%) experienced clinically significant hemorrhage. A third patient had a delayed chronic subdural hematoma discovered 2 months after implantation. Other complications included seizures in 2 patients (1 with hemorrhage), confusion in 4, and behavioral changes in 2, along with other occasional complications. Overall, 26 of 86 patients experienced some adverse event, and in 6 the effects were lasting. Sansur and coauthors specifically reported on the incidence of symptomatic hemorrhage after stereotactic electrode placement.76 Between 1991 and 2005, two neurosurgeons placed 567 electrodes in 259 patients for DBS, radiofrequency lesioning, or recording of seizure foci. The overall risk for symptomatic hemorrhage was 1.2%, and 0.7% of the patients had lasting symptoms. Poor prognostic factors included old age, male sex, hypertension, and the diagnosis of Parkinson’s disease. This suggests that the incidence of symptomatic hemorrhage might be expected to be lower in a cohort of younger patients with epilepsy.
Hamani and Lozano reviewed hardware-related complications of DBS.77 Adverse events were identified in 922 patients. The most common was infection in 6.1%, with the next most common being misplacement or migration of the leads in 5.1%, lead fractures in 5%, and skin erosion in 1.3%. Hariz and associates evaluated complications at 4 years in 69 patients participating in DBS implantation in the STN and globus pallidus pars interna (GPi).78 Seven of the patients required additional surgery because of device-related complications such as infection, lead fracture, or skin erosion. Non–device-related complications included cognitive or memory decline or psychiatric disturbances in 18.8% of the STN patients and 12.5% of the GPi stimulation patients. A total of 4.7% of the STN patients exhibited depression. Speech difficulties were present in about 14% of the patients, disequilibrium or falls in 12.5%, and gait disorders in 14%. Numerous other side effects occurred at lower percentages.
DBS can produce untoward changes in cognition. Hippocampal stimulation with subdural strips or electrodes inserted into the hippocampus at 2 Hz, 3 V, and a pulse duration of 2.5 msec produced measurable deterioration in memory performance.79 It is notable that this low-frequency stimulation of the hippocampus is distinct from the typical 130-Hz stimulation used in more recent clinical trials. However, memory deficits were also observed in an 11-year-old child undergoing 60-Hz, 1-msec, 1.0- to 7.5-mA stimulation, which is closer to the currently used parameters.80
Stimulation of the thalamus can produce paresthesias and other abnormal sensations. Dostrovsky and Lozano studied more than 150 patients undergoing stereotactic thalamotomy for relief of tremor.81 Stimulation of the central thalamus produced paresthesias, illusions of movements, and occasionally other sensations. Stimulation of the centromedian nucleus is usually asymptomatic, but if the current spreads beyond the nucleus, paresthesias and ocular convergence could sometimes occur.28
Mechanisms of Deep Brain Stimulation
At the level of an individual fiber, the effects of stimulation can be inferred from information about orientation of the current (longitudinal versus transverse), resistance of the membrane, extracellular and intracellular tissue, the presence of myelin and nodes of Ranvier, and action potentials and active membrane properties. At the level of a neuronal network, the effects are not predictable. Stimulation can excite inhibitory neurons and change the sign of the effect. Few brain nuclei are round and isotropic, so real-life stimulation probably spreads beyond the target nuclei, with unknown effects on other nuclei and nearby fibers of passage. In the immediate region of the stimulation, neurons may be depolarized to the point of inactivity, but excitatory effects may prevail at a distance.82 The effects of stimulation are well known to depend on the stimulus parameters, such that stimulation at one frequency or intensity may be inhibitory and at others, excitatory. Complex neuronal functions can be disrupted, but not replicated, by stimulation.
Mechanisms have been elucidated by studies involving hippocampal slices by Durand and colleagues83–91 and by Schiff and associates.92–94 Direct or alternating electric fields applied to hippocampal slices that have been made hyper-synchronous by various manipulations appear to be able to disrupt epileptiform activity in the slice. Stimulation produces direct current negative shifts accompanied by marked release of potassium from the intracellular space into the extracellular space. The elevation in extracellular potassium levels produces a depolarization block that causes the neurons to fail to fire because of inactivation of sodium channels. It is not known whether axons may still be able to fire in such circumstances.
At the gross level, stimulation of the STN or ventral intermediate nucleus for tremor or Parkinson’s disease imitates the effects of lesions, thus implying an inhibitory action of stimulation,95 but the mechanisms of DBS are probably not just inhibitory. In live animals and humans, the effects of DBS appear to be more complex than simple depolarization block or neuronal inactivation. Stimulation of the rat STN with adjacent intracellular recording showed that neuronal firing is inhibited after stimulation but that action potentials can still be made to fire during the time of inhibition, which rules out depolarization block.96 Furthermore, stimulation produces sequences of excitation followed by inhibition in some neurons. Unit recordings in patients undergoing implantation for Parkinson’s disease have shown similar inhibition of neurons after stimulation.81 In monkeys made Parkinson-like with 1-methyl-4-phenyl-1,2,3,6-tetrahydropyridine (MPTP), stimulation of the STN excited neurons in the GPi, a target of outgoing STN neurons.97 This suggests a functional stimulatory effect of DBS, even though local STN neurons show inhibition. Metabolic imaging with thalamic ventralis intermedius stimulation for tremor showed increased blood flow in the thalamus and ipsilateral frontal cortex.98 There is an apparent paradox, then, with stimulation at clinically relevant parameters producing neuronal inhibition in the STN but a functional network effect consisting of elements of apparent excitation.
Explanations for the effect of DBS have been sought by examining changes in neurotransmitters after stimulation. STN stimulation increases glutamate in the GPi, consistent with a stimulatory effect on the glutamatergic output of the STN.99 Effects on dopamine are less clear.97 In rats in which seizures were induced with the convulsant drug pentylenetetrazol, although stimulating the AN to attenuate the seizures, microdialysis showed widespread elevation of norepinephrine and relatively localized elevation of serotonin in the AN.100 No major changes in glutamate and γ-aminobutyric acid (GABA) were demonstrated with AN stimulation. Evidence regarding changes in neurotransmitters with stimulation has thus far failed to explain the mechanisms of stimulation.
Scheduled Versus Responsive Stimulation
In the majority of DBS studies for epilepsy and other disorders, stimulation is activated on a predetermined schedule. This can be continuous stimulation, which is the usual practice with stimulation for movement disorders, or intermittent stimulation, which is more common in epilepsy studies. Intermittent stimulation may theoretically have less of an irritating effect on tissue near the electrode and certainly preserves battery life. Vagus nerve stimulation was tested with stimulation cycles on for 30 seconds and off for 5 minutes. Pioneering studies by Cooper and the Velasco brothers used intermittent stimulation, typically with 5-minute cycles. The use of intermittent stimulation presumes that effective stimulation persists longer than the interval of stimulation. The duration of benefit from individual cycles of DBS has never been specifically characterized in patients. The SANTE trial of AN stimulation sponsored by Medtronic is an example of scheduled stimulation.54a Scheduled stimulation is sometimes referred to as open-loop stimulation because there is no feedback governing the timing of stimulation.
Closed-Loop Devices
Closed-loop devices are a basic tenet of control theory, a technique that combines mathematics and engineering. As shown in Figure 69-2, an open-loop device performs an operation on the system to produce a certain output. For an open-loop system to be effective, the control signal has to be selected carefully, which often requires a good understanding of the system being modeled. One drawback with open-loop systems is that they tend to be sensitive to noise in the system, and the controller cannot sense when the output is no longer appropriate.101 The principle of closed-loop devices is a feedback loop that uses a sensor to monitor the output of the system and adjust the control signal accordingly. The comparison between the input and output is known as the “error signal.” The designation “closed loop” refers merely to the appearance of the schematic in Figure 69-2 when the sensor signal is fed back to the input, thereby “closing the loop.”
Can a closed-loop control system be used in a human body? The answer is a resounding “yes,” as long as there is a sensor to produce an error signal. Physiologic homeostasis is nothing more than an intricate web of closed-loop controllers: baroreceptors for blood pressure that modulate the autonomic system, chemoreceptors that modulate the respiratory drive acutely and renal function chronically, and tight control of temperature and glucose concentration. In the case of glucose control, medical research is presently attempting to duplicate it—glucose levels are easily accessible, and insulin can control the levels, thus leaving a clear opportunity to develop an implantable, closed-loop glucose control device.102 There is also strong evidence that brain homeostasis has similar control through mechanisms such as chandelier cells,103 GABA receptors,104 and calcium channel modulation of thalamocortical circuit loops.105
In the realm of electrical stimulation therapy, perhaps the most intriguing example is the unequivocal success of implantable cardiac pacemakers/defibrillators. First developed in the 1970s, closed-loop control systems for cardiac devices began with pressure sensors for feedback control.106,107 They have since become increasingly refined and continue to afford patients better control of various cardiac conditions.108 In the heart, the electrical system is not only straightforward and well understood but also easily accessible to implantable sensors. Determining the presence of ventricular fibrillation can now be readily accomplished. Perhaps most importantly, the treatment (defibrillation) is simple and known to be effective. Thus, the error signal and control signal are both clearly defined, which has led to an industry of implantable cardiac devices that have been successful both clinically and financially.
Initial Closed-Loop Research
Early work on a closed-loop approach was performed in the Soviet Union by S. A. Chkhenkeli’s group and was published primarily in Russian journals. The results were only recently summarized in English.109 This early work began in the 1970s and primarily consisted of experimentation with various open-loop locations for DBS. However, despite the biofeedback studies popular at that time, they also acquired some preliminary data during the initial hospitalization in which the patients could watch spectral integration of their real-time brain recordings and push a button to trigger a stimulus when the power exceeded a certain threshold. These experiments were not designed to test the efficacy of the closed-loop paradigm but included the key components of both feedback control and device tuning. Later, this group presented important data to the World Society for Stereotactic and Functional Neurosurgery in 1997, when they demonstrated that stimuli appeared to decrease interictal discharges and stop spread of the seizure.25 This study provided compelling evidence that brain stimulation could help control seizure activity when triggered by a detected event.
The first step in producing a closed-loop epilepsy control device is to identify an appropriate feedback signal. One form of potential feedback is an “afterdischarge” (AD), abnormal activity that is often seen during cortical brain mapping procedures near the epileptic focus. If left alone, these ADs can either stop or develop into electrical and sometimes clinical seizures. This potential biomarker of epilepsy was used by Lesser and colleagues in 1999 as a manually triggered error signal.110 Responsive electrical stimuli were applied through subdural electrodes in 50-Hz trains of 0.3-msec duration and alternating-polarity square wave pulses. When an AD was noted, an additional stimulation was applied in an attempt to abort it (Fig. 69-3). Although controlled manually, this method was a true closed-loop system. The investigators found that ADs could indeed be interrupted, thus demonstrating proof of principle that closed-loop electrical stimulation can abort ADs provoked during brain mapping by electrical stimulation. Later studies by the same group provided a more rigorous study of these findings. In 2002, Motamedi and colleagues demonstrated that certain control features could be selected to improve treatment efficacy: stimulation was more effective when it was administered at the primary AD focus, within 4.5 seconds of initiation of the AD, or when the AD was negative in polarity.111 Sensor processing was also improved, with the group being able to demonstrate that wavelet analysis could predict which ADs were most likely to be abortable.112
Responsive Neurostimulation for Epilepsy
Clinicians have known for years that some patients use physiologic closed-loop control; that is, they can control their seizures with various stimuli.113–115 Yet it was not until just after Lesser and Chkhenkeli presented their work that technology had reached a point where an implantable closed-loop device became feasible. Several groups became interested in developing a closed-loop epilepsy device at the turn of the 21st century. Gluckman and associates worked in vitro and showed that seizure-like activity can be adaptively controlled with electric fields.93 Osorio and colleagues implemented a working closed-loop system116,117 and tested it in eight patients.39 These eight patients were split into two groups: half received 100- to 500-Hz stimulation for about 1 second directly in the seizure focus, and half received 100- to 200-Hz stimulation for a mean time of 22 seconds into both ANs. Mean amplitude was similar (5 mA) in both groups, and patients were placed in the second group if there was more than a single epileptic focus. This pilot study demonstrated some improvement in seizure frequency in both groups, better in the group with a single focus, and the procedure was found to be safe and well tolerated. In this system, feedback was determined by a seizure detection algorithm that calculated the power ratio between an event and 30 minutes of baseline.
The largest endeavor into closed-loop seizure control to date has been the Responsive Neurostimulator (RNS) study from NeuroPace, Inc (Mountain View, CA). This study used two leads, each containing four electrode contacts (either lead can be a subdural strip electrode or a depth electrode), placed in or near the seizure focus as determined by clinical evaluation. These leads are attached to a self-contained processor that both records from the electrodes and administers electrical stimulation (Fig. 69-4). Placement of the strip and depth electrodes is unique to each individual and is determined by the clinicians. The neurostimulator unit is secured into a recessed titanium plate that is placed within a skull window and fastened with screws to the surrounding skull. This configuration leaves a small profile after the scalp wound is closed, indistinguishable to the eye from the normal head contour. The two electrode leads enter the cranial cavity through separate burr holes over their targeted regions. Their proximal contacts are secured to the implanted device after being tunneled under the scalp. The neurostimulator contains the battery and microprocessor, which is programmable through a telemetric wand.118 It has the capability of sensing four channels of electrocorticography (ECoG), performing analog-to-digital conversion of the signals, extracting three quantitative features from these data, detecting seizures, recording them in memory, and triggering programmable electrical stimulation in response to detection of events. The detection and stimulation parameters are individually adjustable via multiple settings so that it can be tuned to individual patients. The processor uses custom algorithms to determine when to stimulate and is capable of producing a wide variety of stimuli that can be used in attempts to stop seizures.
The key to developing the RNS device was crafting and testing algorithms that could be performed on board the device, thereby allowing the patient to be “unplugged” from higher powered acquisition and processing machines while being monitored for seizure activity. This fully contained monitoring and therapeutic solution therefore works during “real life” rather than only in the hospital and has real potential to improve the patient’s quality of life. Early work demonstrated that computationally feasible algorithms could be reliably implemented in such a device.119 Shortly thereafter, a pilot study demonstrated that seizures could be controlled by responsive stimulation in one patient.120 These results were presented at the annual meeting of the American Epilepsy Society (AES) in 2002, along with work demonstrating further refinement in parameter optimization and tuning121 and the establishment of a clinical trial for the RNS.122 Since that time, the AES meeting has served as the venue for periodic updates in the status of this trial.123–126 This device successfully completed its pivotal clinical trial in 2009 and showed significant improvement in seizure control during the blinded phase. The device is currently seeking FDA approval. One clear benefit of the closed-loop approach is the versatility of having recorded feedback: it effectively provides ambulatory ECoG data and allows tuning of the system in patient-specific training sessions.123 Although the trial is still under way, the preliminary results are promising and the device has a favorable safety record.126 Examples of early success in terminating seizures with both the RNS and the system described by Osorio and coworkers are shown in Figure 69-5.39
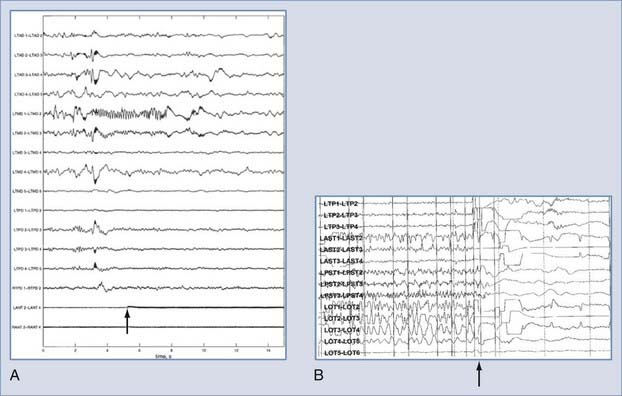
FIGURE 69-5 Examples of responsive antiseizure stimuli. A, An applied stimulus (arrow) after detection of an event prevents the event from lasting its typical length.39 B, An event detected in the Responsive Neurostimulator device is halted by an abortive stimulus (arrow).
(© NeuroPace Inc., Mountain View, CA. Used with permission.)
Closed-Loop Device Considerations for the Future
With current technology, an implantable closed-loop device can perform a significant amount of onboard processing and recording and can upload data via telemetry. The RNS has these capabilities, but there is much room for improvement in that processing speed and storage capacity have greatly increased since its creation. For instance, the RNS device can store only a limited number of discrete short segments of ECoG and needs to download them externally to avoid overwriting them.127 This is only one of several features of the device that can be enhanced as a result of recent advances in hardware and consequently improve clinical results with closed-loop control. Onboard data storage is rapidly dropping in cost and becoming increasingly compact. Available flash memory will allow longer recording times and more feedback data. Battery technology continues to improve and will increase the longevity of the devices. Early devices have significant memory, processing, and battery limitations, thus forming strong motivation for using simple algorithms in a device,119 but these limitations become less stringent with every passing year. Thus, there may be a greater selection of algorithms available in future devices. Perhaps the most important improvements, however, have yet to be discovered. As described in the following section, there is a growing community of researchers trying to determine which electrographic biomarkers of epilepsy can be used as feedback and how best to intervene to prevent seizures.
This chapter has focused on electrical stimulation to abort seizures, but there are many other methods that can also be incorporated into the next-generation implantable antiseizure devices.128 One promising method is being developed by two independent groups and uses a Peltier device to induce focal cooling of the brain cortex to stop seizures.129–132 No clinical trials in humans have yet been attempted, but Peltier devices are becoming small enough to be considered for human implantation. Transcranial magnetic stimulation has had mixed success133–136 but is not feasible as an implantable device. Another method with tremendous therapeutic potential is local drug delivery.137–141 This method uses known antiepileptic medications, stored in a reservoir, and injects them automatically at specific times into the seizure focus. The technology for these systems already exists on both the macroscopic and microscopic scale. It can be used both for open-loop infusion and as a responsive rescue medication and has the added benefit of proven efficacy in stopping seizures. Future nanotechnologies, such as light-triggered ion channel modulation, may provide even more opportunities for closed-loop seizure control devices.142 Other technologies, such as adapting the vagus nerve stimulator (Cyberonics, Inc., Houston) with closed-loop control, are also being explored.143
Seizure Detection and Prediction
Automated methods to detect seizures allow the RNS device to administer stimuli after initiation of the seizure.144–146 Further refinement of seizure detection technology is needed to improve both sensitivity and specificity. Impending signs of seizures may be evident on intracranial EEG traces before the emergence of traditional EEG seizure patterns.147,148 Study of these preictal changes, known as seizure prediction, attempts to find early biomarkers of seizure generation. In the past decade several methods have been tried, including the use of nonlinear mathematics, hidden Markov models, and statistics.149–153 A related problem is refining the data processing within a device by using machine learning algorithms, neural networks, or other methods that use classifiers and feature extraction to help maximize the system’s accuracy in an automated fashion.154–156 To date, these methods have had mixed results, but researchers are optimistic that automated analysis will be able to predict seizures before they occur in some patients.128
Biomarkers of Epilepsy: High-Frequency Oscillations
High-frequency oscillations of EEG potentials may signal impending seizures.157–160 High-frequency discharges, called ripples or fast ripples, tend to localize to epileptic tissue, and changes in their temporal and spatial characteristics may predict seizures before they occur, both temporally and spatially. Additionally, studies of intracranial EEG signals sampled at higher frequencies than typically used in clinical machines have found that there is abnormal activity well above 70 Hz long before seizures occur.147 While clinical research further evaluates the characteristics of this activity, basic science research is striving to understand its physiologic mechanisms.161–166 These findings may indicate that fast activity can be used as a biomarker for seizures, and future technologies may discover ways to use such activity as an early feedback for advanced closed-loop devices.
Conclusion
The first site to be stimulated for epilepsy therapeutics was the cerebellum. Uncontrolled case series were positive, but two small controlled trials were negative, and interest in DBS for treating epilepsy declined. Attention to DBS redeveloped with the success of vagus nerve stimulation for epilepsy and STN and GPi stimulation for movement disorders. Since then, small uncontrolled case series of DBS for epilepsy have been attempted in several sites, including the locus caeruleus, mammillary bodies, corpus callosum, head of the caudate nucleus, CM, AN, STN, hippocampus, and direct stimulation of the cortex. Stimulation can occur on a scheduled basis or can be programmed to be responsive to epileptiform EEG patterns. Interpretation of these trials, however, is difficult in the absence of control groups. Typical reductions in seizure frequency in the range of 30% to 90% are reported, but it is impossible to be certain that these reductions are due to the stimulation. A multicenter, randomized clinical trial of bilateral stimulation of the anterior thalamic nucleus54a showed a 40.5% median reduction in seizures compared with 14.5% in the control group (p = 0.038). Adverse events were typical of those see for implanted brain electrodes. At time of this writing, deep brain stimulation for partial and secondarily generalized seizures in adults now is approved in Europe and several other countries, but not yet in the United States. A multicenter, randomized trial of responsive neurostimulation similarly showed efficacy for stimulation; however, results are not yet published.
Benabid AL, Vercucil L, Benazzouz A, et al. Deep brain stimulation: what does it offer? Adv Neurol. 2003;91:293.
Beric A, Kelly PJ, Rezai A, et al. Complications of deep brain stimulation surgery. Stereotact Funct Neurosurg. 2001;77:73.
Boon P, Vonck K, De Herdt V, et al. Deep brain stimulation in patients with refractory temporal lobe epilepsy. Epilepsia. 2007;48:1551.
Chkhenkeli SA, Sramka M, Lortkipanidze GS, et al. Electrophysiological effects and clinical results of direct brain stimulation for intractable epilepsy. Clin Neurol Neurosurg. 2004;106:318.
Cooper IS, Amin I, Gilman S. The effect of chronic cerebellar stimulation upon epilepsy in man. Trans Am Neurol Assoc. 1973;98:192.
Dostrovsky JO, Lozano AM. Mechanisms of deep brain stimulation. Mov Disord. 2002;17(suppl 3):S63.
Gildenberg PL. Evolution of neuromodulation. Stereotact Funct Neurosurg. 2005;83:71.
Graves NM, Fisher RS. Neurostimulation for epilepsy, including a pilot study of anterior nucleus stimulation. Clin Neurosurg. 2005;52:127.
Handforth A, DeSalles AA, Krahl SE. Deep brain stimulation of the subthalamic nucleus as adjunct treatment for refractory epilepsy. Epilepsia. 2006;47:1239.
Krauss GL, Fisher RS. Cerebellar and thalamic stimulation for epilepsy. Adv Neurol. 1993;63:231.
Lehnertz K, Mormann F, Osterhage H, et al. State-of-the-art of seizure prediction. J Clin Neurophysiol. 2007;24:147.
Litt B, Echauz J. Prediction of epileptic seizures. Lancet Neurol. 2002;1:22.
McIntyre CC, Savasta M, Walter BL, et al. How does deep brain stimulation work? Present understanding and future questions. J Clin Neurophysiol. 2004;21:40.
Osorio I, Frei MG, Sunderam S, et al. Automated seizure abatement in humans using electrical stimulation. Ann Neurol. 2005;57:258.
Osorio I, Overman J, Giftakis J, et al. High frequency thalamic stimulation for inoperable mesial temporal epilepsy. Epilepsia. 2007;48:1561.
Peters TE, Bhavaraju NC, Frei MG, et al. Network system for automated seizure detection and contingent delivery of therapy. J Clin Neurophysiol. 2001;18:545.
Sramka M, Fritz G, Galanda M, et al. Some observations in treatment stimulation of epilepsy. Acta Neurochir (Wien). 1976;23(suppl):257.
Velasco AL, Velasco F, Velasco M, et al. Electrical stimulation of the hippocampal epileptic foci for seizure control: a double-blind, long-term follow-up study. Epilepsia. 2007;48:1895.
Velasco F, Velasco AL, Velasco M, et al. Deep brain stimulation for treatment of the epilepsies: the centromedian thalamic target. Acta Neurochir Suppl. 2007;97:337.
Velasco M, Velasco F, Velasco AL. Centromedian-thalamic and hippocampal electrical stimulation for the control of intractable epileptic seizures. J Clin Neurophysiol. 2001;18:495.
Velasco M, Velasco F, Velasco AL, et al. Subacute electrical stimulation of the hippocampus blocks intractable temporal lobe seizures and paroxysmal EEG activities. Epilepsia. 2000;41:158.
Vonck K, Boon P, Claeys P, et al. Long-term deep brain stimulation for refractory temporal lobe epilepsy. Epilepsia. 2005;46(suppl 5):98.
Worrell G, Wharen R, Goodman R, et al. Safety and evidence for efficacy of an implantable responsive neurostimulator (RNS) for the treatment of medically intractable partial onset epilepsy in adults. Epilepsia. 2005;46:226.
Wright GD, McLellan DL, Brice JG. A double-blind trial of chronic cerebellar stimulation in twelve patients with severe epilepsy. J Neurol Neurosurg Psychiatry. 1984;47:769.
1 Stillings D. Mediterranean origins of electrotherapy. Electomagn Biol Med. 1971;2:181.
2 Gildenberg PL. Evolution of neuromodulation. Stereotact Funct Neurosurg. 2005;83:71.
3 Rowbottom M, Susskind C. Electricity and Medicine, History of their Interaction. San Francisco: San Francisco Press; 1984.
4 Heath RG. Electrical self-stimulation of the brain in man. Am J Psychiatry. 1963;120:571.
5 Rosenow J, Das K, Rovit RL, et al. Irving S. Cooper and his role in intracranial stimulation for movement disorders and epilepsy. Stereotact Funct Neurosurg. 2002;78:95.
6 Moruzzi G. Sui rapporti fra cervelletto e corteccia cerebrale: azione d’impulsi cerebellari sulle attivitá motrici provocate dalla stimolazione faradica o chimica del giro sigmoideo nel gatto. Arch Fisiol. 1941;41:87.
7 Cooke PM, Snider RS. Some cerebellar influences on electrically-induced cerebral seizures. Epilepsia. 1955;4:19.
8 Cooper IS, Amin I, Gilman S. The effect of chronic cerebellar stimulation upon epilepsy in man. Trans Am Neurol Assoc. 1973;98:192.
9 Krauss GL, Fisher RS. Cerebellar and thalamic stimulation for epilepsy. Adv Neurol. 1993;63:231.
10 Wright GD, McLellan DL, Brice JG. A double-blind trial of chronic cerebellar stimulation in twelve patients with severe epilepsy. J Neurol Neurosurg Psychiatry. 1984;47:769.
11 Van Buren JM, Wood JH, Oakley J, et al. Preliminary evaluation of cerebellar stimulation by double-blind stimulation and biological criteria in the treatment of epilepsy. J Neurosurg. 1978;48:407.
12 Davis R. Cerebellar stimulation for cerebral palsy spasticity, function, and seizures. Arch Med Res. 2000;31:290.
13 Davis R, Emmonds SE. Cerebellar stimulation for seizure control: 17-year study. Stereotact Funct Neurosurg. 1992;58:200.
14 Davis R, Engle H, Kudzma J, et al. Update of chronic cerebellar stimulation for spasticity and epilepsy. Appl Neurophysiol. 1982;45:44.
15 Davis R, Gray E, Engle H, et al. Reduction of intractable seizures using cerebellar stimulation. Appl Neurophysiol. 1983;46:57.
16 Davis R, Gray EF. Technical problems and advances in the cerebellar-stimulating systems used for reduction of spasticity and seizures. Appl Neurophysiol. 1980;43:230.
17 Velasco F, Carrillo-Ruiz JD, Brito F, et al. Double-blind, randomized controlled pilot study of bilateral cerebellar stimulation for treatment of intractable motor seizures. Epilepsia. 2005;46:1071.
18 Cooper IS, Upton AR. Effects of cerebellar stimulation on epilepsy, the EEG and cerebral palsy in man. Electroencephalogr Clin Neurophysiol Suppl. 1978;34:349.
19 Faber J, Vladyka V. Antiepileptic effect of electric stimulation of the locus coeruleus in man. Act Nerv Super (Praha). 1983;25:304.
20 Feinstein B, Gleason CA, Libet B. Stimulation of locus coeruleus in man. Preliminary trials for spasticity and epilepsy. Stereotact Funct Neurosurg. 1989;52:26.
21 van Rijckevorsel K, Abu Serieh B, de Tourtchaninoff M, et al. Deep EEG recordings of the mammillary body in epilepsy patients. Epilepsia. 2005;46:781.
22 Marino Junior R, Gronich G. Corpus callosum stimulation and stereotactic callosotomy in the management of refractory generalized epilepsy. Preliminary communication. Arq Neuropsiquiatr. 1989;47:320.
23 Sramka M, Fritz G, Galanda M, et al. Some observations in treatment stimulation of epilepsy. Acta Neurochir (Wien). 1976;23(suppl):257.
24 Sramka M, Chkhenkeli SA. Clinical experience in intraoperational determination of brain inhibitory structures and application of implanted neurostimulators in epilepsy. Stereotact Funct Neurosurg. 1990;54-55:56.
25 Chkhenkeli SA, Chkhenkeli IS. Effects of therapeutic stimulation of nucleus caudatus on epileptic electrical activity of brain in patients with intractable epilepsy. Stereotact Funct Neurosurg. 1997;69:221.
26 Chkhenkeli SA, Sramka M, Lortkipanidze GS, et al. Electrophysiological effects and clinical results of direct brain stimulation for intractable epilepsy. Clin Neurol Neurosurg. 2004;106:318.
27 Velasco F, Velasco M, Ogarrio C, et al. Electrical stimulation of the centromedian thalamic nucleus in the treatment of convulsive seizures: a preliminary report. Epilepsia. 1987;28:421.
28 Fisher RS, Uematsu S, Krauss GL, et al. Placebo-controlled pilot study of centromedian thalamic stimulation in treatment of intractable seizures. Epilepsia. 1992;33:841.
29 Velasco F, Velasco M, Marquez I, et al. Role of the centromedian thalamic nucleus in the genesis, propagation and arrest of epileptic activity. An electrophysiological study in man. Acta Neurochir Suppl. 1993;58:201.
30 Velasco F, Velasco M, Velasco AL, et al. Electrical stimulation of the centromedian thalamic nucleus in control of seizures: long-term studies. Epilepsia. 1995;36:63.
31 Velasco F, Velasco M, Jimenez F, et al. Predictors in the treatment of difficult-to-control seizures by electrical stimulation of the centromedian thalamic nucleus. Neurosurgery. 2000;47:295.
32 Velasco F, Velasco M, Jimenez F, et al. Stimulation of the central median thalamic nucleus for epilepsy. Stereotact Funct Neurosurg. 2001;77:228.
33 Velasco AL, Velasco F, Jimenez F, et al. Neuromodulation of the centromedian thalamic nuclei in the treatment of generalized seizures and the improvement of the quality of life in patients with Lennox-Gastaut syndrome. Epilepsia. 2006;47:1203.
34 Velasco AL, Velasco M, Velasco F, et al. Subacute and chronic electrical stimulation of the hippocampus on intractable temporal lobe seizures: preliminary report. Arch Med Res. 2000;31:316.
35 Velasco M, Velasco F, Velasco AL, et al. Subacute electrical stimulation of the hippocampus blocks intractable temporal lobe seizures and paroxysmal EEG activities. Epilepsia. 2000;41:158.
36 Velasco M, Velasco F, Velasco AL. Centromedian-thalamic and hippocampal electrical stimulation for the control of intractable epileptic seizures. J Clin Neurophysiol. 2001;18:495.
37 Vonck K, Boon P, Achten E, et al. Long-term amygdalohippocampal stimulation for refractory temporal lobe epilepsy. Ann Neurol. 2002;52:556.
38 Vonck K, Boon P, Claeys P, et al. Long-term deep brain stimulation for refractory temporal lobe epilepsy. Epilepsia. 2005;46(suppl 5):98.
39 Osorio I, Frei MG, Sunderam S, et al. Automated seizure abatement in humans using electrical stimulation. Ann Neurol. 2005;57:258.
40 Tellez-Zenteno JF, McLachlan RS, Parrent A, et al. Hippocampal electrical stimulation in mesial temporal lobe epilepsy. Neurology. 2006;66:1490.
41 Velasco AL, Velasco F, Velasco M, et al. Electrical stimulation of the hippocampal epileptic foci for seizure control: a double-blind, long-term follow-up study. Epilepsia. 2007;48:1895.
42 Boon P, Vonck K, De Herdt V, et al. Deep brain stimulation in patients with refractory temporal lobe epilepsy. Epilepsia. 2007;48:1551.
43 Chabardes S, Kahane P, Minotti L, et al. Deep brain stimulation in epilepsy with particular reference to the subthalamic nucleus. Epileptic Disord. 2002;4(suppl 3):S83.
44 Benabid AL, Vercucil L, Benazzouz A, et al. Deep brain stimulation: what does it offer? Adv Neurol. 2003;91:293.
45 Neme S, Montgomery EBJr, Rezai A, et al. Subthalamic nucleus stimulation in patients with intractable epilepsy: the Cleveland experience. In: Luders HO, editor. Deep Brain Stimulation and Epilepsy. New York: Martin Dunitz; 2004:349.
46 Handforth A, DeSalles AA, Krahl SE. Deep brain stimulation of the subthalamic nucleus as adjunct treatment for refractory epilepsy. Epilepsia. 2006;47:1239.
47 Vesper J, Steinhoff B, Rona S, et al. Chronic high-frequency deep brain stimulation of the STN/SNr for progressive myoclonic epilepsy. Epilepsia. 2007;48:1984.
48 Cooper IS, Amin I, Riklan M, et al. Chronic cerebellar stimulation in epilepsy. Clinical and anatomical studies. Arch Neurol. 1976;33:559.
49 Sussman NM, Goldman HW, Jackel A, et al. Anterior thalamic stimulation in medically intractable epilepsy. Part II. Preliminary clinical results. Epilepsia. 1988;29:677.
50 Hodaie M, Wennberg RA, Dostrovsky JO, et al. Chronic anterior thalamus stimulation for intractable epilepsy. Epilepsia. 2002;43:603.
51 Kerrigan JF, Litt B, Fisher RS, et al. Electrical stimulation of the anterior nucleus of the thalamus for the treatment of intractable epilepsy. Epilepsia. 2004;45:346.
52 Lee KJ, Jang KS, Shon YM. Chronic deep brain stimulation of subthalamic and anterior thalamic nuclei for controlling refractory partial epilepsy. Acta Neurochir Suppl. 2006;99:87.
53 Lim SN, Lee ST, Tsai YT, et al. Electrical stimulation of the anterior nucleus of the thalamus for intractable epilepsy: a long-term follow-up study. Epilepsia. 2007;48:342.
54 Osorio I, Overman J, Giftakis J, et al. High frequency thalamic stimulation for inoperable mesial temporal epilepsy. Epilepsia. 2007;48:1561.
54a Fisher R, Salanova V, Witt T, et al. Electrical stimulation of the anterior nucleus of thalamus for treatment of refractory epilepsy. Epilepsia. 2010;51:899-908.
55 Mirski MA, Ferrendelli JA. Interruption of the mammillothalamic tract prevents seizures in guinea pigs. Science. 1984;226:72.
56 Mirski MA, Fisher RS. Electrical stimulation of the mammillary nuclei increases seizure threshold to pentylenetetrazol in rats. Epilepsia. 1994;35:1309.
57 Nishida N, Huang ZL, Mikuni N, et al. Deep brain stimulation of the posterior hypothalamus activates the histaminergic system to exert antiepileptic effect in rat pentylenetetrazol model. Exp Neurol. 2007;205:132.
58 Oakley JC, Ojemann GA. Effects of chronic stimulation of the caudate nucleus on a preexisting alumina seizure focus. Exp Neurol. 1982;75:360.
59 Psatta DM. Control of chronic experimental focal epilepsy by feedback caudatum stimulations. Epilepsia. 1983;24:444.
60 La Grutta V, Sabatino M. Focal hippocampal epilepsy: effect of caudate stimulation. Exp Neurol. 1988;99:38.
61 Velasco M, Velasco F, Velasco AL, et al. Effect of chronic electrical stimulation of the centromedian thalamic nuclei on various intractable seizure patterns: II. Psychological performance and background EEG activity. Epilepsia. 1993;34:1065.
62 Velasco F, Velasco AL, Velasco M, et al. Deep brain stimulation for treatment of the epilepsies: the centromedian thalamic target. Acta Neurochir Suppl. 2007;97:337.
63 Limousin P, Krack P, Pollak P, et al. Electrical stimulation of the subthalamic nucleus in advanced Parkinson’s disease. N Engl J Med. 1998;339:1105.
64 Vercueil L, Benazzouz A, Deransart C, et al. High-frequency stimulation of the subthalamic nucleus suppresses absence seizures in the rat: comparison with neurotoxic lesions. Epilepsy Res. 1998;31:39.
65 Cooper IS, Upton AR, Amin I. Reversibility of chronic neurologic deficits. Some effects of electrical stimulation of the thalamus and internal capsule in man. Appl Neurophysiol. 1980;43:244.
66 Cooper IS, Upton AR, Amin I, et al. Evoked metabolic responses in the limbic-striate system produced by stimulation of anterior thalamic nucleus in man. Int J Neurol. 1984;18:179.
67 Cooper IS, Upton AR. Therapeutic implications of modulation of metabolism and functional activity of cerebral cortex by chronic stimulation of cerebellum and thalamus. Biol Psychiatry. 1985;20:811.
68 Upton AR, Cooper IS, Springman M, et al. Suppression of seizures and psychosis of limbic system origin by chronic stimulation of anterior nucleus of the thalamus. Int J Neurol. 1985;19-20:223.
69 Graves NM, Fisher RS. Neurostimulation for epilepsy, including a pilot study of anterior nucleus stimulation. Clin Neurosurg. 2005;52:127.
70 Brown WJ, Babb TL, Soper HV, et al. Tissue reactions to long-term electrical stimulation of the cerebellum in monkeys. J Neurosurg. 1977;47:366.
71 Henderson JM, O’Sullivan DJ, Pell M, et al. Lesion of thalamic centromedian–parafascicular complex after chronic deep brain stimulation. Neurology. 2001;56:1576.
72 Salcman M, Defendini R, Correll J, et al. Neuropathological changes in cerebellar biopsies of epileptic patients. Ann Neurol. 1978;3:10.
73 Pilitsis JG, Chu Y, Kordower J, et al. Postmortem study of deep brain stimulation of the anterior thalamus: case report. Neurosurgery. 2008;62:E530.
74 Deep-brain stimulation of the subthalamic nucleus or the pars interna of the globus pallidus in Parkinson’s disease. N Engl J Med. 2001;345:956.
75 Beric A, Kelly PJ, Rezai A, et al. Complications of deep brain stimulation surgery. Stereotact Funct Neurosurg. 2001;77:73.
76 Sansur CA, Frysinger RC, Pouratian N, et al. Incidence of symptomatic hemorrhage after stereotactic electrode placement. J Neurosurg. 2007;107:998.
77 Hamani C, Lozano AM. Hardware-related complications of deep brain stimulation: a review of the published literature. Stereotact Funct Neurosurg. 2006;84:248.
78 Hariz MI, Krack P, Alesch F, et al. Multicentre European study of thalamic stimulation for parkinsonian tremor: a 6 year follow-up. J Neurol Neurosurg Psychiatry. 2008;79:694.
79 Fernandez G, Hufnagel A, Helmstaedter C, et al. Memory function during low intensity hippocampal electrical stimulation in patients with temporal lobe epilepsy. Eur J Neurol. 1996;3:335.
80 Cohen MJ, Holmes GL, Campbell R, et al. Memory performance following unilateral electrical stimulation of the hippocampus in a child with right temporal lobe epilepsy. J Epilepsy. 1990;3:115.
81 Dostrovsky JO, Lozano AM. Mechanisms of deep brain stimulation. Mov Disord. 2002;17(suppl 3):S63.
82 Schlag J, Villablanca J. A quantitative study of temporal and spatial response patterns in a thalamic cell population electrically stimulated. Brain Res. 1968;8:255.
83 Bikson M, Lian J, Hahn PJ, et al. Suppression of epileptiform activity by high frequency sinusoidal fields in rat hippocampal slices. J Physiol. 2001;531:181.
84 Durand D. Electrical stimulation can inhibit synchronized neuronal activity. Brain Res. 1986;382:139.
85 Durand DM, Jensen A, Bikson M. Suppression of neural activity with high frequency stimulation. Conf Proc IEEE Eng Med Biol Soc. 2006;1:1624.
86 Ghai RS, Bikson M, Durand DM. Effects of applied electric fields on low-calcium epileptiform activity in the CA1 region of rat hippocampal slices. J Neurophysiol. 2000;84:274.
87 Jensen AL, Durand DM. Suppression of axonal conduction by sinusoidal stimulation in rat hippocampus in vitro. J Neural Eng. 2007;4:1.
88 Kayyali H, Durand D. Effects of applied currents on epileptiform bursts in vitro. Exp Neurol. 1991;113:249.
89 Lian J, Bikson M, Sciortino C, et al. Local suppression of epileptiform activity by electrical stimulation in rat hippocampus in vitro. J Physiol. 2003;547:427.
90 Nakagawa M, Durand D. Suppression of spontaneous epileptiform activity with applied currents. Brain Res. 1991;567:241.
91 Warren RJ, Durand DM. Effects of applied currents on spontaneous epileptiform activity induced by low calcium in the rat hippocampus. Brain Res. 1998;806:186.
92 Gluckman BJ, Neel EJ, Netoff TI, et al. Electric field suppression of epileptiform activity in hippocampal slices. J Neurophysiol. 1996;76:4202.
93 Gluckman BJ, Nguyen H, Weinstein SL, et al. Adaptive electric field control of epileptic seizures. J Neurosci. 2001;21:590.
94 Richardson KA, Gluckman BJ, Weinstein SL, et al. In vivo modulation of hippocampal epileptiform activity with radial electric fields. Epilepsia. 2003;44:768.
95 Vitek JL. Mechanisms of deep brain stimulation: excitation or inhibition. Mov Disord. 2002;17(suppl 3):S69.
96 Lee KH, Roberts DW, Kim U. Effect of high-frequency stimulation of the subthalamic nucleus on subthalamic neurons: an intracellular study. Stereotact Funct Neurosurg. 2003;80:32.
97 McIntyre CC, Savasta M, Walter BL, et al. How does deep brain stimulation work? Present understanding and future questions. J Clin Neurophysiol. 2004;21:40.
98 Perlmutter JS, Mink JW, Bastian AJ, et al. Blood flow responses to deep brain stimulation of thalamus. Neurology. 2002;58:1388.
99 Windels F, Bruet N, Poupard A, et al. Effects of high frequency stimulation of subthalamic nucleus on extracellular glutamate and GABA in substantia nigra and globus pallidus in the normal rat. Eur J Neurosci. 2000;12:4141.
100 Ziai WC, Sherman DL, Bhardwaj A, et al. Target-specific catecholamine elevation induced by anticonvulsant thalamic deep brain stimulation. Epilepsia. 2005;46:878.
101 Kamen EW, Heck BS. Fundamentals of Signals and Systems Using the Web and Matlab. Upper Saddle River, NJ: Pearson Prentice Hall; 2007.
102 Chee F, Fernando T, van Heerden PV. Closed-loop glucose control in critically ill patients using continuous glucose monitoring system (CGMS) in real time. IEEE Trans Inf Technol Biomed. 2003;7:43.
103 DeFelipe J. Chandelier cells and epilepsy. Brain. 1999;122:1807.
104 Ben-Ari Y. Seizures beget seizures: the quest for GABA as a key player. Crit Rev Neurobiol. 2006;18:135.
105 Huguenard JR. Neuronal circuitry of thalamocortical epilepsy and mechanisms of antiabsence drug action. Adv Neurol. 1999;79:991.
106 Lown B, Axelrod P. Implanted standby defibrillators. Circulation. 1972;46:637.
107 Mirowski M, Mower MM, Staewen WS, et al. Standby automatic defibrillator. An approach to prevention of sudden coronary death. Arch Intern Med. 1970;126:158.
108 Saoudi N, Appl U, Anselme F, et al. How smart should pacemakers be? Am J Cardiol. 1999;83:180D.
109 Chkhenkeli SA. Direct deep brain stimulation: a first step twoards the feedback control of seizures. In: Milton J, Jung P, editors. Epilepsy as a Dynamic Disease. Berlin: Springer-Verlag, 2003.
110 Lesser RP, Kim SH, Beyderman L, et al. Brief bursts of pulse stimulation terminate afterdischarges caused by cortical stimulation. Neurology. 1999;53:2073.
111 Motamedi GK, Lesser RP, Miglioretti DL, et al. Optimizing parameters for terminating cortical afterdischarges with pulse stimulation. Epilepsia. 2002;43:836.
112 Mizuno-Matsumoto Y, Motamedi GK, Webber WRS, et al. Wavelet-crosscorrelation analysis can help predict whether bursts of pulse stimulation will terminate afterdischarges. Clin Neurophysiol. 2002;113:33.
113 Pritchard PB3rd, Holmstrom VL, Giacinto J. Self-abatement of complex partial seizures. Ann Neurol. 1985;18:265.
114 Papini M, Pasquinelli A, Armellini M, et al. Alertness and incidence of seizures in patients with Lennox-Gastaut syndrome. Epilepsia. 1984;25:161.
115 Paulson GW. Inhibition of seizures. Dis Nerv Syst. 1963;24:657.
116 Osorio I, Frei MG, Manly BF, et al. An introduction to contingent (closed-loop) brain electrical stimulation for seizure blockage, to ultra-short-term clinical trials, and to multidimensional statistical analysis of therapeutic efficacy. J Clin Neurophysiol. 2001;18:533.
117 Peters TE, Bhavaraju NC, Frei MG, et al. Network system for automated seizure detection and contingent delivery of therapy. J Clin Neurophysiol. 2001;18:545.
118 NeuroPace Inc. Responsive Neurostimulation for the Treatment of Epilepsy. CA: Mountain View; 2008.
119 Echauz J, Esteller R, Tcheng T, et al. Long-term validation of detection algorithms suitable for an implantable device. Epilepsia. 2001;42(suppl 7):35.
120 Murro AM, Park Y, Greene D, et al. Closed loop neurostimulation in patient with intractable epilepsy. Paper presented at the annual meeting of the American Clinical Neurophysiology Society, New Orleans, 2002.
121 Esteller R, Echauz J, Pless B, et al. Real-time simulation of a seizure detection system suitable for an implantable device. Epilepsia. 2002;43(suppl 7):46.
122 Bergey GK, Britton JW, Cascino GD, et al. Implementation of an external responsive neurostimulator system (eRNS) in patients with intractable epilepsy undergoing intracranial seizure monitoring. Epilepsia. 2002;43(suppl 7):191.
123 Murro AM, Park Y, Bergey GK, et al. Multicenter study of acute responsive neurostimulation in patients with intractable epilepsy. Epilepsia. 2003;44(suppl 9):326.
124 Kossoff EH, Ritzl EK, Politsky JM, et al. Effect of an external responsive neurostimulator on seizures and electrographic discharges during subdural electrode monitoring. Epilepsia. 2004;45:1560.
125 Worrell G, Wharen R, Goodman R, et al. Safety and evidence for efficacy of an implantable responsive neurostimulator (RNS) for the treatment of medically intractable partial onset epilepsy in adults. Epilepsia. 2005;46:226.
126 Barkley GL, Smith B, Bergey GK, et al. Safety and preliminary efficacy of the RNS responsive neurostimulator for the treatment of intractable epilepsy in adults. Paper presented at the annual meeting of the American Epilepsy Society, San Diego, CA, 2006.
127 Ritzl EK, Kossoff EH, Bergey GK, et al. Complementing the responsive neurostimulator system with a patient operated data transmitter—on demand monitoring in the outpatient environment. Epilepsia. 2005;46(suppl 8):223.
128 Stacey WC, Litt B. Technology insight: neuroengineering and epilepsy: designing devices for seizure control. Nat Clin Pract Neurol. 2008;4:190.
129 Tanaka N, Fujii M, Imoto H, et al. Effective suppression of hippocampal seizures in rats by direct hippocampal cooling with a Peltier chip. J Neurosurg. 2008;108:791.
130 Imoto H, Fujii M, Uchiyama J, et al. Use of a Peltier chip with a newly devised local brain-cooling system for neocortical seizures in the rat. Technical note. J Neurosurg. 2006;104:150.
131 Rothman SM, Smyth MD, Yang XF, et al. Focal cooling for epilepsy: an alternative therapy that might actually work. Epilepsy Behav. 2005;7:214.
132 Yang XF, Duffy DW, Morley RE, et al. Neocortical seizure termination by focal cooling: temperature dependence and automated seizure detection. Epilepsia. 2002;43:240.
133 Joo EY, Han SJ, Chung SH, et al. Antiepileptic effects of low-frequency repetitive transcranial magnetic stimulation by different stimulation durations and locations. Clin Neurophysiol. 2007;118:702.
134 Bae EH, Schrader LM, Machii K, et al. Safety and tolerability of repetitive transcranial magnetic stimulation in patients with epilepsy: a review of the literature. Epilepsy Behav. 2007;10:521.
135 Fregni F, Otachi PT, Do Valle A, et al. A randomized clinical trial of repetitive transcranial magnetic stimulation in patients with refractory epilepsy. Ann Neurol. 2006;60:447.
136 Theodore WH, Hunter K, Chen R, et al. Transcranial magnetic stimulation for the treatment of seizures: a controlled study. Neurology. 2002;59:560.
137 Fisher RS, Chen DK. New routes for delivery of anti-epileptic medications. Acta Neurol Taiwan. 2006;15:225.
138 Ludvig N, Kuzniecky RI, Baptiste SL, et al. Epidural pentobarbital delivery can prevent locally induced neocortical seizures in rats: the prospect of transmeningeal pharmacotherapy for intractable focal epilepsy. Epilepsia. 2006;47:1792.
139 Lohman RJ, Liu L, Morris M, et al. Validation of a method for localised microinjection of drugs into thalamic subregions in rats for epilepsy pharmacological studies. J Neurosci Methods. 2005;146:191.
140 Stein AG, Eder HG, Blum DE, et al. An automated drug delivery system for focal epilepsy. Epilepsy Res. 2000;39:103.
141 Tamargo RJ, Rossell LA, Kossoff EH, et al. The intracerebral administration of phenytoin using controlled-release polymers reduces experimental seizures in rats. Epilepsy Res. 2002;48:145.
142 Gorostiza P, Isacoff E. Optical switches and triggers for the manipulation of ion channels and pores. Mol Biosyst. 2007;3:686.
143 Trafton A. Epilepsy breakthrough on horizon. Available at http://web.mit.edu/newsoffice/2006/epilepsy.html
144 Firpi H, Goodman ED, Echauz J. Epileptic seizure detection using genetically programmed artificial features. IEEE Trans Biomed Eng. 2007;54:212.
145 Saab ME, Gotman J. A system to detect the onset of epileptic seizures in scalp EEG. Clin Neurophysiol. 2005;116:427.
146 Lee HC, van Drongelen W, McGee AB, et al. Comparison of seizure detection algorithms in continuously monitored pediatric patients. J Clin Neurophysiol. 2007;24:137.
147 Litt B, Esteller R, Echauz J, et al. Epileptic seizures may begin hours in advance of clinical onset: a report of five patients. Neuron. 2001;30:51.
148 Haut SR, Hall CB, LeValley AJ, et al. Can patients with epilepsy predict their seizures? Neurology. 2007;68:262.
149 Wong S, Gardner AB, Krieger AM, et al. A stochastic framework for evaluating seizure prediction algorithms using hidden Markov models. J Neurophysiol. 2007;97:2525.
150 Mormann F, Andrzejak RG, Elger CE, et al. Seizure prediction: the long and winding road. Brain. 2007;130:314.
151 Schelter B, Winterhalder M, Drentrup HF, et al. Seizure prediction: the impact of long prediction horizons. Epilepsy Res. 2007;73:213.
152 Lehnertz K, Mormann F, Osterhage H, et al. State-of-the-art of seizure prediction. J Clin Neurophysiol. 2007;24:147.
153 Litt B, Echauz J. Prediction of epileptic seizures. Lancet Neurol. 2002;1:22.
154 Gardner AB, Worrell GA, Marsh E, et al. Human and automated detection of high-frequency oscillations in clinical intracranial EEG recordings. Clin Neurophysiol. 2007;118:1134.
155 DAlessandro M, Vachtsevanos G, Esteller R, et al. A multi-feature and multi-channel univariate selection process for seizure prediction. Clin Neurophysiol. 2005;116:506.
156 DAlessandro M, Esteller R, Vachtsevanos G, et al. Epileptic seizure prediction using hybrid feature selection over multiple intracranial EEG electrode contacts: a report of four patients. IEEE Trans Biomed Eng. 2003;50:603.
157 Miller JW. Are ictal fast ripples an electronic signature for the seizure-onset zone? Epilepsy Curr. 2007;7:7.
158 Traub RD. Fast oscillations and epilepsy. Epilepsy Curr. 2003;3:77.
159 Bragin A, Wilson CL, Staba RJ, et al. Interictal high-frequency oscillations (80-500 Hz) in the human epileptic brain: entorhinal cortex. Ann Neurol. 2002;52:407.
160 Bragin A, Engel JJr, Wilson CL, et al. High-frequency oscillations in human brain. Hippocampus. 1999;9:137.
161 Dzhala VI, Staley KJ. Mechanisms of fast ripples in the hippocampus. J Neurosci. 2004;24:8896.
162 Foster DJ, Wilson MA. Reverse replay of behavioural sequences in hippocampal place cells during the awake state. Nature. 2006;440:680.
163 Geisler C, Brunel N, Wang XJ. Contributions of intrinsic membrane dynamics to fast network oscillations with irregular neuronal discharges. J Neurophysiol. 2005;94:4344.
164 ONeill J, Senior T, Csicsvari J. Place-selective firing of CA1 pyramidal cells during sharp wave/ripple network patterns in exploratory behavior. Neuron. 2006;49:143.
165 Maier N, Nimmrich V, Draguhn A. Cellular and network mechanisms underlying spontaneous sharp wave-ripple complexes in mouse hippocampal slices. J Physiol. 2003;550:873.
166 Traub RD, Bibbig A. A model of high-frequency ripples in the hippocampus based on synaptic coupling plus axon-axon gap junctions between pyramidal neurons. J Neurosci. 2000;20:2086.