CHAPTER 82 Deep Brain Stimulation for Dystonia
Torsion dystonia is a neurological disorder characterized by twisting, repetitive movements that result in abnormal, often painful postures.1 Different muscle groups may be involved to a variable extent and severity. Dystonia is not one disease; rather, it is a neurological manifestation of many pathologic conditions, most of which are poorly characterized. Prevalence estimates for primary dystonia in the general population range from 2 to 50 cases per million for early-onset dystonia and from 30 to 7320 cases per million for late-onset dystonia.2 However, prevalence rates are significantly higher in some ethnic groups.2,3
Because of the limitations of available medical therapies, a variety of surgical interventions that target both the peripheral and central nervous systems have been attempted for dystonia.4,5 The dystonia literature is filled with case reports and small cohort studies, mostly relating mixed or conflicting outcomes. Long-term results in significant numbers of patients are virtually absent.
In the more recent past, the successful use of deep brain stimulation (DBS) for medically refractory Parkinson’s disease (PD) and essential tremor (ET) led to investigation of its utility in treating dystonia. In particular, the observation that pallidal interventions improve “off-state” dystonia in PD patients shifted attention from the thalamus to the globus pallidus interna (GPi) as the target of choice for treating primary dystonia.6 The result of these efforts has been development of the most effective treatment currently available for primary dystonia and one of the most successful applications of neuromodulation technology yet described. This chapter focuses on the current status of pallidal DBS for dystonia. Because of space constraints, discussion of alternative therapeutic targets for stimulation is limited.
Diagnosis and Classification of Dystonia
Dystonia may be classified in three ways: (1) by the anatomic distribution of the abnormal movements, (2) by the age at onset of symptoms (early versus late), and (3) by the absence or presence of a specific underlying cause (i.e., primary versus secondary).1 Focal dystonias (e.g., writer’s cramp, spasmodic torticollis) are limited to a single body region, segmental dystonia affects contiguous body parts, and widespread involvement of the axial and limb musculature characterizes generalized dystonia. Patients with early symptom onset (i.e., age < 26 years) are more likely to have a heritable form of dystonia and more likely to suffer generalized symptoms.1,3
A dystonia is classified as primary or idiopathic when no structural brain abnormality or specific toxic, metabolic, traumatic, or infectious cause is identified. The heritable forms of dystonia are traditionally included in this group. At least 13 different mutations are now associated with dystonia, with each mutation occurring at a unique gene locus.7 The most common form of genetic dystonia results from a GAG deletion of the gene encoding the protein torsin A.7 This mutation, referred to as DYT1, is associated with a form of childhood-onset dystonia formerly known as dystonia musculorum deformans or Oppenheim’s disease. DYT1-associated dystonia is inherited in an autosomal dominant pattern but with a penetrance of just 30% to 40%, thus suggesting that additional genetic or environmental factors, or both, contribute to expression of the dystonic phenotype.7
When a structural brain abnormality or specific underlying cause is identified, a dystonia is classified as secondary or symptomatic.1 Symptomatic dystonia is more prevalent than primary dystonia and may arise from a variety of causes, including static encephalopathy, stroke, traumatic brain injury, or any number of toxic, metabolic, or infectious disorders. Consequently, this is a heterogeneous patient population with varied pathophysiologies and responses to treatment.1
Medical Therapy for Dystonia
In most cases, medical therapy for dystonia is limited to symptom control and is marginally effective.8 Anticholinergic medications (e.g., trihexyphenidyl) are the mainstay of medical therapy but often yield only modest improvements and, at the high doses used for dystonia, may cause significant side effects such as drowsiness, blurred vision, and poor memory. Additional medications for dystonia include baclofen, benzodiazepines, and tetrabenazine. A minority of patients with symptomatic generalized dystonia will benefit from specific therapy targeted at the underlying disorder. Children and adolescents with “clinically pure” dystonia of unknown etiology should be evaluated for Wilson’s disease and undergo a trial of levodopa therapy because a small subpopulation with dopa-responsive dystonia will experience a profound and sustained response to this medication.8
Targeted injections of botulinum toxin (Botox) can alleviate focal dystonias, but this intervention is impractical in patients with generalized symptoms.8,9 Some patients will not respond to Botox initially, and in up to 10%, resistance may develop over time through the production of blocking antibodies.9
Surgical Therapy for Dystonia
Surgical intervention for dystonia should be considered when a patient’s symptoms are disabling and the response to medical therapy is either inadequate or limited by side effects. Historically, surgical interventions for dystonia have targeted both the peripheral and central nervous systems.4,5 Peripheral denervation procedures for focal dystonias have largely been supplanted by chemical denervation with Botox.9 Chronic intrathecal baclofen infusions can alleviate dystonia of the lower extremities, but this intervention may not be appropriate for dystonias affecting the arms and neck, and positive responses may not result in significant functional gains.10
Advances in stereotactic technique, the success of DBS for PD and ET, and the observation that pallidotomy improves off-medication dystonia in PD patients6 renewed interest in basal ganglia interventions for torsion dystonia in the 1990s. Stereotactic pallidotomy does improve symptoms of primary generalized dystonia (PGD)11; however, unilateral pallidotomy may not sufficiently treat generalized symptoms, and bilateral pallidotomy entails significant risk, including cognitive dysfunction, dysarthria, dysphagia, and limb weakness.12 Consequently, DBS, which is reversible and may be used bilaterally with relative safety, has emerged as a preferable alternative.
Deep Brain Stimulation Procedure
Surgical Procedure
The implanted device is composed of four primary components (Fig. 82-1) that are implanted in two stages. During the first stage, the stimulating lead or leads are implanted into the GPi stereotactically and secured by means of an anchoring system that also covers the bur hole. The remaining two components (i.e., the extension cable or cables and pulse generator or generators) are implanted during the second procedure, which may be performed on the same day or shortly thereafter. It is acceptable to implant DBS leads bilaterally during the same procedure. Dystonia patients are typically much younger than patients with PD and ET and, in our experience, tolerate the bilateral frontal lobe penetrations without difficulty.
Anatomic Targeting
Stereotactic head frames remain the “gold standard” for implanting DBS leads; however, “frameless” technologies are being used for DBS surgery with greater frequency.13 We use axial and coronal fast spin echo/inversion recovery (FSE/IR) MRI for anatomic targeting because the images are acquired rapidly (6 to 9 minutes per scan) and provide superior resolution of the commissures and deep nuclei (Fig. 82-2). The thickness of the axial slices (3 mm) required to generate these high-resolution images increases our initial targeting error along the z-axis (i.e., depth), but this is compensated for by MER, which delineates the depth of specific structures along the implantation trajectory with a resolution of approximately 0.1 mm. The scanning parameters for FSE/IR MRI are presented in Table 82-1. These images alone are sufficient for implanting the DBS system under microelectrode guidance; however, additional image sets such as gadolinium-enhanced three-dimensionally acquired T1-weighted MRI (e.g., spoiled gradient echo [SPGR]) or computed tomography (CT), or both, may also be used. We have found that the volume error of fiducial registration is enhanced with SPGR MRI (unpublished results). Moreover, contrast enhancement demonstrates the cortical veins so that they may be avoided when selecting an entry point. CT provides the most geometrically accurate images for fiducial registration and may also be performed rapidly on the morning of surgery.
TABLE 82-1 Scanning Parameters for Axial Fast Spin Echo Inversion Recovery Images
Excitation time (Te) | 120 msec |
Relaxation time (Tr) | 10,000 msec |
Inversion time (Ti) | 2200 msec |
Bandwidth | 20.83 KHz |
Field of view | 24 cm |
Slice thickness | 3 mm |
Slice spacing | 0 mm |
Frequency | 192 Hz |
Phase | 160 |
Number of excitations | 1 |
Frequency direction | Anteroposterior |
Autocontrol frequency | Water |
Flow compensation direction | Slice direction |
We target the internal pallidal site first described by Leksell, which lies 19 to 22 mm lateral, 2 to 3 mm anterior, and 4 to 5 mm inferior to the midcommissural point (MCP).14 The coordinates for the MCP are determined by calculating the arithmetic mean of the coordinates for the anterior and posterior commissures, which may be determined directly. The calculated target point should be visualized on both axial and coronal images and should lie 2 to 3 mm superior and lateral to the optic tract (Fig. 82-2B). Our preferred trajectory rises at a 60- to 65-degree angle anterior and superior to the intercommissural plane and 0 to 10 degrees lateral to the vertical axis. This trajectory allows one to avoid the ipsilateral lateral ventricle and still use nearly parasagittal trajectories, which facilitates the process of mapping the intraoperative MER data to human stereotactic atlases (see later).
Microelectrode Recording
We use single-cell MER to refine our anatomic targeting. The finer details of our MER technique are beyond the scope of this report but are provided elsewhere.15 The need for MER is hotly debated; however, we find that MER provides important information that other neurophysiologic localization techniques simply do not. First, MER delineates the borders and expanses of the globus pallidus externa (GPe) and GPi along a given trajectory with a spatial resolution of approximately 100 µm. These data may be mapped onto scaled sagittal sections of human stereotactic atlases to determine the anatomic location of the recording trajectory. Acceptable trajectories for implantation include a 3- to 4-mm span of the GPe and at least 7 mm of the GPi. Such a trajectory must pass through the heart of the GPi and will allow three to four contacts to be positioned comfortably within the nucleus, depending on the lead used (Fig. 82-3).16 Second, the detection of kinesthetic cells confirms that the trajectory is traversing the sensorimotor subregion of the GPi, the physiologically defined target for the procedure. Third, delineating the inferior border of the GPi refines the depth of implantation. Fourth, identifying the optic tract 2 to 3 mm inferior to the GPi exit point confirms that the trajectory is exiting the nucleus inferiorly, not posteriorly into the internal capsule. Identification of the optic tract provides an additional level of confidence that the lead will be well positioned, but this should not be viewed as an absolute requirement for implantation because the optic tract may not be identified in many cases.
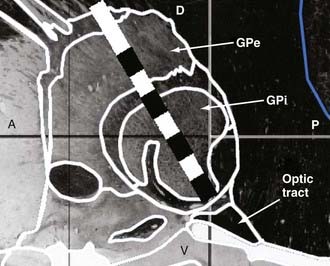
FIGURE 82-3 Pallidal lead implantation. Our preferred lead position within the globus pallidus is depicted. A schematic of the model 3387 lead (Medtronic Inc., Minneapolis, MN), which has four 1.5-mm long cylindrical contacts with 1.5-mm interelectrode spacing, is superimposed on a sagittal image, 20 mm lateral of midline, derived from the Schaltenbrand and Wahren Atlas.16 With the deepest contact (contact 0) positioned at the inferior border of the globus pallidus interna (GPi), three contacts can fit within the nucleus. A, anterior; D, dorsal; GPe, globus pallidus externa; P, posterior; V, ventral.
Macroelectrode Stimulation
The DBS lead is inserted along the desired trajectory, with the deepest contact (contact 0) left at the physiologically defined inferior border of the GPi. C-arm fluoroscopy is used to confirm that the lead has traveled to the desired point relative to the frame (Fig. 82-4). Before it is secured, the acute effects of stimulation via the lead are tested. Testing is performed in bipolar mode with the following parameters: pulse width, 90 µsec; frequency, 130 Hz; and amplitude, 0 to 4 V. Stimulation amplitudes greater than 4 V are not used because we have never required amplitudes this great for therapy. The initial test is performed with the deepest pair of contacts (i.e., 0 to 1+) because these are most likely to generate adverse effects (AEs). If no AEs are observed, testing continues in a ventral to dorsal sequence. Unlike PD, dystonia requires days to weeks of stimulation therapy before improvements are apparent. Therefore, a lack of improvement in response to intraoperative stimulation should not be viewed as an indicator of poor lead placement. Rather, one must have faith that if the MER data are consistent with good placement and no AEs are observed with up to 4 V of stimulation, the lead is well positioned.
The lead is secured at the skull with a “cap” that also covers the bur hole. Fluoroscopy is used to confirm that the lead was not displaced from its desired position during fixation. The remaining length of the lead is encircled around the bur hole cap and left in the subgaleal space. The incision is irrigated with antibiotic-containing saline and closed anatomically. After removing the stereotactic frame, the patient is transported to the radiology department, where postoperative MRI is performed to confirm that the leads are well positioned and no hemorrhage has taken place (Fig. 82-5). Patients are observed overnight in the neurosurgical intensive care unit and discharged the following day.
Programming the Device
The device or devices are activated 2 to 4 weeks after lead implantation to allow the surgical incisions to heal. There is no consensus regarding the optimal settings for treating dystonia because few systematic evaluations of varying stimulus parameters have been conducted. Instead, therapy is currently guided by published case series, which report positive responses with wide pulses (210 to 400 µsec) and high frequencies (≥130 Hz).17–21 Although effective, these parameters rapidly deplete the pulse generators and necessitate frequent replacement (12 to 24 months). In our experience, stimulation at lower frequencies (60 to 80 Hz) may be just as effective as high-frequency stimulation.22,23 Because these settings deliver less electrical energy to the brain, AEs are less likely to occur and the life span of the pulse generators is prolonged.23,24
Patients return every 2 to 4 weeks for evaluation during the first 3 months and then every 3 to 6 months after that. During each visit the patient is assessed with a variety of standardized clinical rating scales, including the Burke-Fahn-Marsden Dystonia Rating Scale (BFMDRS).25
Clinical Results
Initial case reports of pallidal DBS for dystonia were published in 1999. Coubes and coauthors reported the case of an 8-year-old girl with PGD whose symptoms were so severe that she required sedation and mechanical ventilation.17 Thirty-six months after surgery, she had returned to school with nearly normal neurological function. Kumar and associates also reported dramatic improvement in one patient with severe PGD and correlated the clinical response to normalization of motor cortical activity on positron emission tomography.26 Later, Krauss and coworkers noted 78% and 70% improvement in the BFMDRS scores of two patients with PGD 2 years after surgery.18
The results of larger case series support these preliminary findings. Yianni and coauthors reported their results in treating 25 patients with various forms of dystonia and found that all patient subgroups were improved by pallidal DBS.19 Coubes and colleagues reported a mean 79% improvement in the BFMDRS motor subscore and a mean 65% improvement in the disability subscore 2 years after surgery in 31 patients with PGD.20 They noted that patients improved steadily over a period of 12 to 24 months and that children fared marginally better than adults. According to a recent review by Ostrem and Starr, at least 249 individual cases of pallidal DBS for primary dystonia have been reported in the literature to date.21 Most are included in small, open-label case series, and most report improvements in BFMDRS scores of 60% to 70%.
Two prospective studies demonstrated that activation of the devices and not mere insertion of the leads is required to realize clinical improvement. Vidailhet and associates examined 22 PGD patients treated with bilateral pallidal DBS at three French centers.27 Double-blind evaluations conducted 3 months after surgery showed significantly better motor function with stimulation than without. One year after surgery, the mean BFMDRS motor score was improved 51%, with a third of the patients experiencing greater than 75% improvement. They found that phasic symptoms improved more rapidly than fixed dystonic postures. A more recent report documented that the motor improvement in this cohort has been maintained for 3 years.28
In 2006, Kupsch and coauthors published the only prospective, randomized, double-blind, sham stimulation–controlled study of pallidal DBS for dystonia.29 Forty patients with primary segmental or generalized dystonia underwent pallidal DBS surgery at multiple centers in Germany. Twenty patients were randomized to therapeutic stimulation and 20 to sham stimulation for a period of 3 months, at which time their clinical status was assessed by blinded raters using the BFMDRS. The BFMDRS motor subscores in the patients who received therapeutic stimulation improved 40% at 3 months as compared with 5% in the control group. The control group was then provided therapeutic stimulation, with a resulting equivalent improvement (37%) over the subsequent 3 months.
Indicators of Response to Deep Brain Stimulation
Besides the relatively crude classification of primary generalized or segmental versus secondary dystonia, little is known about the clinical factors that presage a positive response to pallidal DBS. In 2008, Isaias and coworkers addressed this issue by examining the clinical results of 39 consecutive PGD patients treated at one institution and monitored for at least 1 year.30 Overall, motor improvement 1 year postoperatively was equivalent to that reported previously; however, the authors found that the 6 patients in their series who had fixed skeletal deformities (FSDs) at the time of surgery experienced a more limited improvement than did the 33 patients without FSDs. By subdividing the BFMDRS motor scores anatomically, they further demonstrated that limited improvement occurred at the legs and axis, consistent with the fact that all 6 suffered from scoliosis. Two of these 6 improved further after corrective spinal procedures, but their final results still did not equal those observed in the remaining patients without FSDs.
Tisch and colleagues examined the relationship of lead position relative to the intercommissural plane and clinical outcome and found that leads positioned more posteriorly and ventrally within the GPi yielded greater BFMDRS motor score improvement than did leads positioned more anteriorly and dorsally.31 These results confirmed and expanded on previous results published by Starr and associates32 and Hamani and coworkers33 and suggest that Leksell’s posteroventral GPi target remains the optimal site within the pallidum for treating dystonia, whether by stimulation or ablation.
Stimulation Frequency
The impressive responses generated with pallidal DBS for PGD were achieved with stimulation frequencies of 130 Hz or greater and pulse durations of at least 140 µsec. Such parameters rapidly deplete the implanted pulse generators and necessitate frequent surgical replacement (i.e., every 12 to 36 months). To address this difficulty, Alterman and colleagues conducted an evaluation of 15 consecutive PGD patients who were treated with pallidal stimulation at just 60 Hz and found that their clinical response was equivalent to that achieved with higher stimulation frequencies.23 More importantly, none of these patients have yet required surgery to replace a depleted generator or required increases in stimulation therapy with up to 4 years of follow-up.24 In addition to reducing the frequency at which these patients may require surgery, the finding that 60-Hz stimulation may be as effective as stimulation at higher frequencies raises important questions about the mechanisms through which pallidal DBS exerts its effects in both dystonia and PD.23
Longevity of Response
Little has been reported concerning the longevity of the response of PGD to pallidal DBS. As stated earlier, Vidhailet and associates reported stable results in their cohort of 22 PGD patients for up to 3 years.28 Our own results in 30 PGD patients who have been monitored for at least 2 years suggest that the clinical response to DBS is stable for up to 7 years.24
Pallidal Deep Brain Stimulation for Cervical Dystonia
Data supporting the use of pallidal DBS for cervical dystonia (CD) are not yet as developed as those available for PGD. Preliminary reports of small cases series suggest that CD is responsive to bilateral pallidal DBS, with improvements in the Toronto Western Spasmodic Torticollis Rating Scale (TWSTRS) ranging from 43% to 76%.21,34,35 Kiss and coworkers conducted the only multicenter, prospective, single-blind trial of pallidal DBS, but in only 10 CD patients.36 They reported significant improvement in the TWSTRS severity, disability, and pain subscales, as well as reduced symptoms of depression and enhanced quality-of-life measures. Overall, the response of CD to GPi-DBS appears to be less consistent than that observed with PGD. Larger and longer term analyses of DBS for CD can be expected in the next few years that will hopefully reveal the indicators of a positive clinical response, including optimal lead position and stimulation parameters.
Pallidal Deep Brain Stimulation for Primary Cranial-Cervical Dystonia (Meige’s Syndrome)
The results of pallidal DBS for Meige’s syndrome have now been reported in 13 patients from a handful of centers.21 Ostrem and coauthors reported a mean BFMDRS improvement of 71% in six patients 6 months after surgery.37 Some of these patients experienced stimulation-induced bradykinesia in preoperatively unaffected limbs. The optimal stimulation parameters and targeting for Meige’s syndrome remain to be elucidated.
Pallidal Deep Brain Stimulation for Secondary Dystonia
Thus far, pallidal DBS has proved far less effective for secondary dystonia than for PGD.38–44 Patients with secondary dystonia represent a heterogeneous population with regard to etiology, clinical signs, and long-term prognosis. In addition, many of them have neurological deficits in addition to dystonia (e.g., seizures, spastic paresis, cerebellar and brainstem dysfunction, and developmental delay) that limit their functional response to DBS. Most studies report little or no benefit and even worsening of symptoms after DBS for secondary dystonia. Our own experience in treating patients with secondary dystonia of various causes confirms that responses in this group are more modest than the results obtained in patients with primary dystonia;38 however, we have operated on a 12-year-old boy with severe generalized dystonia secondary to perinatal anoxic brain injury who responded quickly (within 2 weeks) and dramatically to bilateral GPi-DBS (unpublished results). Despite his prolonged anoxia and the severity of his dystonia, his brain anatomy was well preserved. This patient is similar to patient 9 in the report by Zorzi and associates,43 whose BFMDRS score improved 65% after pallidal DBS surgery. The responses of these two patients, as well as reports of positive responses to DBS in patients with tardive dystonia,45–47 suggest that some individuals with secondary dystonia will respond favorably to DBS. Dramatic improvement in patients with pantothenate kinase–associated neurodegeneration (PKAN) have also been reported,48 and in one other case the benefits have lasted for 5 years.49 The preoperative indicators of a positive response in patients with secondary dystonia are currently unknown, but normal brain MRI findings may be a predictor of favorable outcome.50
Complications of Deep Brain Stimulation Therapy
Overall, both DBS surgery and chronic electrical stimulation of the internal pallidum are well tolerated in this population. In our series of 82 dystonia patients (73 primary, 9 secondary) operated on between December 2000 and August 2008, there have been no intracerebral hemorrhages or adverse neurological events. Perioperative infections necessitating the removal of nine devices developed in 8 patients (9.8%). Each patient was successfully treated with antibiotics and underwent reimplantation without adverse sequelae. Three patients (3.7%) fractured an extension cable, a complication that is reported to occur more frequently with dystonia than with PD or ET.51 In one of our cases, withdrawal of the patient’s medications at the time of surgery precipitated a dystonic crisis, for which the child required prolonged hospitalization, large doses of lorazepam (Ativan), and early activation of her DBS devices.
Alternative Targets for Deep Brain Stimulation for Torsion Dystonia
The dramatic results achieved with pallidal DBS for primary dystonia have thus far slowed the exploration of alternative targets for therapy. Kleiner-Fisman and coauthors reported significant improvements in both the BFMDRS and TWSTRS scores of two patients with primary, predominantly CD who were treated with bilateral DBS at the subthalamic nucleus.52 Two other patients improved marginally. Sun and colleagues reported that bilateral subthalamic DBS yielded immediate and sustained improvement in various forms of dystonia with significantly less electrical energy than required for treatment at the GPi.53 Of their series of nine patients with secondary dystonia of various causes treated with subthalamic DBS, Zhang and colleagues reported improvements in two patients with tardive dystonia and one patient with posttraumatic dystonia 3 months postoperatively.54 Finally, Goto and coworkers recently reported significant benefit from Vo (ventralis-oralis)-complex thalamic DBS in one patient with focal hand dystonia after previous reports that radiofrequency thalamotomy also improved both writer’s cramp and musician’s dystonia.55
Acknowledgment
I wish to thank Donald Weisz, Ph.D., for his assistance in the production of Figure 82-3.
Alterman R, Miravite J, Shils J, et al. 60 Hertz pallidal deep brain stimulation for primary torsion dystonia. Neurology. 2007;69:681-688.
Bressman SB. Dystonia: phenotypes and genotypes. Rev Neurol (Paris). 2003;159:849-856.
Coubes P, Cif L, El Fertit H, et al. Electrical stimulation of the globus pallidus internus in patients with primary generalized dystonia: long-term results. J Neurosurg. 2004;101:189-194.
Defazio G, Abbruzzese G, Livrea P, et al. Epidemiology of primary dystonia. Lancet Neurol. 2004;3:673-678.
Eltahawy HA, Saint-Cyr J, Giladi N, et al. Primary dystonia is more responsive than secondary dystonia to pallidal interventions: outcome after pallidotomy or pallidal deep brain stimulation. Neurosurgery. 2004;54:613-619.
Fahn S. Idiopathic torsion dystonia. In: Calne DB, editor. Neurodegenerative Diseases. Philadelphia: WB Saunders; 1994:705-715.
Hamani C, Moro E, Zadikoff C, et al. Location of active contacts in patients with primary dystonia treated with globus pallidus deep brain stimulation. Neurosurgery. 2008;62:217-223.
Hua Z, Guodong G, Qinchuan L, et al. Analysis of complications of radiofrequency pallidotomy. Neurosurgery. 2003;52:89-99.
Isaias IU, Alterman RL, Tagliati M. Deep brain stimulation for primary dystonia: long-term outcomes. Arch Neurol. 2009;66:465-470.
Isaias IU, Alterman RL, Tagliati M. Outcome predictors of pallidal stimulation in patients with primary dystonia: the role of disease duration. Brain. 2008;131:1895-1902.
Kiss ZHT, Doig-Beyaert K, Eliasziw M, et al. The Canadian multicentre study of deep brain stimulation for cervical dystonia. Brain. 2007;130:2879-2886.
Kupsch A, Benecke R, Muller J, et al. Pallidal deep-brain stimulation in primary generalized or segmental dystonia. N Engl J Med. 2006;355:1978-1990.
Laitinen LV, Bergenheim AT, Hariz MI. Leksell’s posteroventral pallidotomy in the treatment of Parkinson’s disease. J Neurosurg. 1992;76:53-61.
Ostrem JL, Starr PA. Treatment of dystonia with deep brain stimulation. Neurotherapeutics. 2008;5:320-330.
Shils J, Tagliati M, Alterman R. Neurophysiological monitoring during neurosurgery for movement disorders. In: Deletis V, Shils J, editors. Neurophysiology in Neurosurgery. San Diego, CA: Academic Press; 2002:393-436.
Tagliati M, Blatt K, Bressman SB. Generalized torsion dystonia. In: Noseworthy J, editor. Neurological Therapeutics: Principles and Practice. London: Martin Dunitz; 2003:3548-3566.
Tisch S, Zrinzo L, Limousin P, et al. Effect of electrode contact location on clinical efficacy of pallidal deep brain stimulation in primary generalized dystonia. J Neurol Neurosurg Psychiatry. 2007;78:1314-1319.
Vidailhet M, Vercueil L, Houeto JL, et al. Bilateral deep brain stimulation of the globus pallidus in primary generalized dystonia. N Engl J Med. 2005;352:459-467.
1 Fahn S. Idiopathic torsion dystonia. In: Calne DB, editor. Neurodegenerative Diseases. Philadelphia: WB Saunders; 1994:705-715.
2 Defazio G, Abbruzzese G, Livrea P, et al. Epidemiology of primary dystonia. Lancet Neurol. 2004;3:673-678.
3 Bressman SB, Sabatti C, Raymond D, et al. The DYT1 phenotype and guidelines for diagnostic testing. Neurology. 2000;54:1746-1752.
4 Krack P, Vercueil L. Review of the functional surgical treatment of dystonia. J Eur Neurol. 2001;8:389-399.
5 Braun V, Richter HP. Selective peripheral denervation for spasmodic torticollis: 13-year experience with 155 patients. J Neurosurg. 2002;97:207-212.
6 Lozano AM, Lang AE, Galvez-Jimenez N, et al. Effect of GPi pallidotomy on motor function in Parkinson’s disease. Lancet. 1995;346:1383-1387.
7 Bressman SB. Dystonia: phenotypes and genotypes. Rev Neurol (Paris). 2003;159:849-856.
8 Tagliati M, Blatt K, Bressman SB. Generalized torsion dystonia. In: Noseworthy J, editor. Neurological Therapeutics: Principles and Practice. London: Martin Dunitz; 2003:3548-3566.
9 Greene P, Fahn S, Diamond B. Development of resistance to botulinum toxin type A in patients with torticollis. Mov Disord. 1994;9:213-217.
10 Ford B, Greene P, Louis ED, et al. Use of intrathecal baclofen in the treatment of patients with dystonia. Arch Neurol. 1996;53:1241-1246.
11 Ondo WG, Desaloms JM, Jankovic J, et al. Pallidotomy for generalized dystonia. Mov Disord. 1998;13:693-698.
12 Hua Z, Guodong G, Qinchuan L, et al. Analysis of complications of radiofrequency pallidotomy. Neurosurgery. 2003;52:89-99.
13 Bjartmarz H, Rehncrona S. Comparison of accuracy and precision between frame-based and frameless stereotactic navigation for deep brain stimulation electrode implantation. Stereotact Funct Neurosurg. 2007;85:235-242.
14 Laitinen LV, Bergenheim AT, Hariz MI. Leksell’s posteroventral pallidotomy in the treatment of Parkinson’s disease. J Neurosurg. 1992;76:53-61.
15 Shils J, Tagliati M, Alterman R. Neurophysiological monitoring during neurosurgery for movement disorders. In: Deletis V, Shils J, editors. Neurophysiology in Neurosurgery. San Diego, CA: Academic Press; 2002:393-436.
16 Schaltenbrand G, Wahren W. Atlas for Stereotaxy of the Human Brain. New York: Thieme; 1977.
17 Coubes P, Echenne B, Roubertie A, et al. Treatment of early-onset generalized dystonia by chronic bilateral stimulation of the internal globus pallidus. Apropos of a case. Neurochirurgie. 1999;45:139-144.
18 Krauss JK, Loher TJ, Weigel R, et al. Chronic stimulation of the globus pallidus internus for treatment of non-dYT1 generalized dystonia and choreoathetosis: 2-year follow-up. J Neurosurg. 2003;98:785-792.
19 Yianni J, Bain P, Giladi N, et al. Globus pallidus internus deep brain stimulation for dystonic conditions: a prospective audit. Mov Disord. 2003;18:436-442.
20 Coubes P, Cif L, El Fertit H, et al. Electrical stimulation of the globus pallidus internus in patients with primary generalized dystonia: long-term results. J Neurosurg. 2004;101:189-194.
21 Ostrem JL, Starr PA. Treatment of dystonia with deep brain stimulation. Neurotherapeutics. 2008;5:320-330.
22 Alterman R, Shils J, Miravite J, et al. A lower stimulation frequency can enhance tolerability and efficacy of pallidal deep brain stimulation for dystonia. Mov Disord. 2007;22:366-368.
23 Alterman R, Miravite J, Shils J, et al. 60 Hertz pallidal deep brain stimulation for primary torsion dystonia. Neurology. 2007;69:681-688.
24 Isaias IU, Alterman RL, Tagliati M. Deep brain stimulation for primary dystonia: long-term outcomes. Arch Neurol. 2009;66:465-470.
25 Volkmann J, Benecke R. Deep brain stimulation for dystonia: patient selection and evaluation. Mov Disord. 2002;17(suppl 3):S112-S115.
26 Kumar R, Dagher A, Hutchison WD, et al. Globus pallidus deep brain stimulation for generalized dystonia: clinical and PET investigation. Neurology. 1999;53:871-874.
27 Vidailhet M, Vercueil L, Houeto JL, et al. Bilateral deep brain stimulation of the globus pallidus in primary generalized dystonia. N Engl J Med. 2005;352:459-467.
28 Vidailhet M, Vercueil L, Houeto JL, et al. Bilateral, pallidal, deep-brain stimulation in primary generalised dystonia: a prospective 3 year follow-up study. Lancet Neurol. 2007;6:223-229.
29 Kupsch A, Benecke R, Muller J, et al. Pallidal deep-brain stimulation in primary generalized or segmental dystonia. N Engl J Med. 2006;355:1978-1990.
30 Isaias IU, Alterman RL, Tagliati M. Outcome predictors of pallidal stimulation in patients with primary dystonia: the role of disease duration. Brain. 2008;131:1895-1902.
31 Tisch S, Zrinzo L, Limousin P, et al. Effect of electrode contact location on clinical efficacy of pallidal deep brain stimulation in primary generalized dystonia. J Neurol Neurosurg Psychiatry. 2007;78:1314-1319.
32 Starr PA, Turner RS, Rau G, et al. Microelectrode-guided implantation of deep brain stimulators into the globus pallidus internus for dystonia: techniques, electrode locations, and outcomes. Neurosurg Focus. 2004;17(1):E4.
33 Hamani C, Moro E, Zadikoff C, et al. Location of active contacts in patients with primary dystonia treated with globus pallidus deep brain stimulation. Neurosurgery. 2008;62:217-223.
34 Hung SW, Hamani C, Lozano AM, et al. Long-term outcome of bilateral pallidal deep brain stimulation for primary cervical dystonia. Neurology. 2007;68:457-459.
35 Bittar RG, Yianni J, Wang SY, et al. Deep brain stimulation for generalized dystonia and spasmodic torticollis. J Clin Neurosci. 2005;12:12-16.
36 Kiss ZHT, Doig-Beyaert K, Eliasziw M, et al. The Canadian multicentre study of deep brain stimulation for cervical dystonia. Brain. 2007;130:2879-2886.
37 Ostrem JL, Marks WJJr, Volz MM, et al. Pallidal deep brain stimulation in patients with cranial-cervical dystonia (Meige syndrome). Mov Disord. 2007;22:1885-1891.
38 Alterman RL, Snyder BJ. Deep brain stimulation for torsion dystonia. Acta Neurochir Suppl. 2007;97:191-199.
39 Kupsch A, Kuehn A, Klaffke S, et al. Deep brain stimulation in dystonia. J Neurol. 2003;250(suppl 1):47-52.
40 Bronte-Stewart H. Surgical therapy for dystonia. Curr Neurol Neurosci Rep. 2003;3:296-305.
41 Yianni J, Bain PG, Gregory RP, et al. Post-operative progress of dystonia patients following globus pallidus internus deep brain stimulation. Eur J Neurol. 2003;10:239-247.
42 Vercueil L, Pollak P, Fraix V, et al. Deep brain stimulation in the treatment of severe dystonia. J Neurol. 2001;248:695-700.
43 Zorzi G, Marras C, Nardocci N, et al. Stimulation of the globus pallidus internus for childhood-onset dystonia. Mov Disord. 2005;20:1194-1200.
44 Eltahawy HA, Saint-Cyr J, Giladi N, et al. Primary dystonia is more responsive than secondary dystonia to pallidal interventions: outcome after pallidotomy or pallidal deep brain stimulation. Neurosurgery. 2004;54:613-619.
45 Franzini A, Marras C, Ferroli P, et al. Long-term high-frequency bilateral pallidal stimulation for neuroleptic-induced tardive dystonia. Report of two cases J Neurosurg. 2005;102:721-725.
46 Trottenberg T, Volkmann J, Deuschl G, et al. Treatment of severe tardive dystonia with pallidal deep brain stimulation. Neurology. 2005;64:344-346.
47 Sako W, Goto S, Shimazu H, et al. Bilateral deep brain stimulation of the globus pallidus internus in tardive dystonia. Mov Disord. 2008;23:1929-1931.
48 Castelnau P, Cif L, Valente EM, et al. Pallidal stimulation improves pantothenate kinase–associated neurodegeneration. Ann Neurol. 2005;57:738-741.
49 Krause M, Fogel W, Tronnier V, et al. Long-term benefit to pallidal deep brain stimulation in a case of dystonia secondary to pantothenate kinase–associated neurodegeneration. Mov Disord. 2006;21:2255-2257.
50 Vercueil L, Krack P, Pollak P. Results of deep brain stimulation for dystonia: a critical reappraisal. Mov Disord. 2002;17(suppl 3):S89-S93.
51 Yianni J, Nandi D, Shad A, et al. Increased risk of lead fracture and migration in dystonia compared with other movement disorders following deep brain stimulation. J Clin Neurosci. 2004;11:243-245.
52 Kleiner-Frisman G, Liang GS, Moberg PJ, et al. Subthalamic nucleus deep brain stimulation for severe idiopathic dystonia: impact on severity, neuropsychological status, and quality of life. J Neurosurg. 2007;107:29-36.
53 Sun B, Chen S, Zhan S, et al. Subthalamic nucleus stimulation for primary dystonia and tardive dystonia. Acta Neurochir Suppl. 2007;97:207-214.
54 Zhang J, Zhang K, Wang Z, et al. Deep brain stimulation in the treatment of secondary dystonia. Chin Med J. 2006;119:2069-2074.
55 Goto S, Shimazu H, Matsuzaki K, et al. Thalamic Vo-complex vs pallidal deep brain stimulation for focal hand dystonia. Neurology. 2008;70:1500-1501.