Contemporary issues and theories of motor control, motor learning, and neuroplasticity
MARGARET L. ROLLER, PT, MS, DPT, ROLANDO T. LAZARO, PT, PhD, DPT, GCS, NANCY N. BYL, PT, MPH, PhD, FAPTA and DARCY A. UMPHRED, PT, PhD, FAPTA
After reading this chapter the student or therapist will be able to:
1. Identify the evolution of motor control theories and discuss the utility of current theory in clinical practice.
2. Identify body structures and functions that contribute to the control of human posture and movement.
3. Relate the cognitive, associative, and autonomous stages of motor learning to behavior and skill performance.
4. Describe the variety of practice conditions that may be used to enhance motor learning within a practice session.
5. Apply motor learning variables related to person, task, and environment within the therapeutic setting.
6. Discuss neuroplasticity theories that explain how the nervous system adapts to demands placed on learning and performance.
7. Discuss the relationship among motor control, motor learning, and neuroplasticity in the production of functional movement behaviors.
This chapter introduces the reader to basic concepts of motor control, motor learning, and neuroplasticity. Figures and tables are provided within each section to emphasize and summarize concepts. A patient case example is used to illustrate concepts in this chapter as they apply to the evaluation and management of people with neurological conditions. This chapter provides a foundation for chapters in Section II: Rehabilitation Management of Clients with Neurological System Pathology, and acts as a foundation for interacting with and treating patients in any clinical setting.
Motor control
Motor control is defined as “the systematic transmission of nerve impulses from the motor cortex to motor units, resulting in coordinated contractions of muscles.”1
Theories and models of motor control
We begin this section with a summary and historical perspective of motor control theories (Table 4-1). The control of human movement has been described in many different ways. The production of reflexive, automatic, adaptive, and voluntary movements and the performance of efficient, coordinated, goal-directed movement patterns involve multiple body systems (input, output, and central processing) and multiple levels within the nervous system. Each model of motor control that is discussed in this section has both merit and disadvantage in its ability to supply a comprehensive picture of motor behavior. These theories serve as a basis for predicting motor responses during patient examination and treatment. They help explain motor skill performance, potential, constraints, limitations, and deficits. They allow the clinician to (1) identify problems in motor performance, (2) develop treatment strategies to help clients remediate performance problems, and (3) evaluate the effectiveness of intervention strategies employed in the clinic. Selecting and using an appropriate model of motor control is important for the analysis and treatment of clients with dysfunctions of posture and movement. As long as the environment and task demands affect changes in the CNS and the individual has the desire to learn, the adaptable nervous system will continue to learn, modify, and adapt motor plans throughout life.
TABLE 4-1
MOTOR CONTROL THEORY | AUTHOR AND DATE | PREMISE |
Reflex Theory | Sherrington 1906244 | Movement is controlled by stimulus-response. Reflexes are combined into actions that create behavior. |
Hierarchical Theories | Adams 1971245 | Cortical centers control movement in a top-down manner throughout the nervous system.Closed-loop mode: sensory feedback is needed and used to control the movement. Open-loop mode: movements are preprogrammed and no feedback is used. |
Dynamical Systems Theory | Bernstein 196710Turvey 1977246 Kelso and Tuller 1984247 Thelen 1987248 |
Movement emerges to control degrees of freedom.Patterns of movements self-organize within the characteristics of environmental conditions and the existing body systems of the individual. Functional synergies are developed naturally through practice and experience and help solve the problem of coordinating multiple muscles and joint movements at once. |
Motor Program Theory | Schmidt 1976249 | Adaptive, flexible motor programs (MPs) and generalized motor programs (GMPs) exist to control actions that have common characteristics. |
Ecological Theories | Gibson and Pick 2000250 | The person, the task, and the environment interact to influence motor behavior and learning. The interaction of the person with any given environment provides perceptual information used to control movement. The motivation to solve problems to accomplish a desired movement task goal facilitates learning. |
Systems Model | Shumway-Cook 200735 | Multiple body systems overlap to activate synergies for the production of movements that are organized around functional goals. Considers interaction of the person with the environment. |
Motor programs and central pattern generators
A motor program (MP) is a learned behavioral pattern defined as a neural network that can produce rhythmic output patterns with or without sensory input or central control.2 MPs are sets of movement commands, or “rules,” that define the details of skilled motor actions. An MP defines the specific muscles that are needed, the order of muscle activation, and the force, timing, sequence, and duration of muscle contractions. MPs help control the degrees of freedom of interacting body structures, and the number of ways each individual component acts. A generalized motor program (GMP) defines a pattern of movement, rather than every individual aspect of a movement. GMPs allow for the adjustment, flexibility, and adaptation of movement features according to environmental demands. The existence of MPs and GMPs is a generally accepted concept; however, hard evidence that an MP or a GMP exists has yet to be found. Advancements in brain imaging techniques may substantiate this theory in the future.2,3
In contrast to MPs, a central pattern generator (CPG) is a genetically predetermined movement pattern.4 CPGs exist as neural networks within the CNS and have the capability of producing rhythmic, patterned outputs resembling normal movement. These movements have the capability of occurring without sensory feedback inputs or descending motor inputs. Two characteristic signs of CPGs are that they result in the repetition of movements in a rhythmic manner and that the system returns to its starting condition when the process ceases.5 Both MPs and CPGs contribute to the development, refinement, production, and recovery of motor control throughout life.
Body structures and functions that contribute to the control of human posture and movement
Role of sensory information in motor control
Sensory information is necessary during the acquisition phase of learning a new motor skill and is useful for controlling movements during the execution of the motor plan.6–8 However, sensory information is not always necessary when performing well-learned motor behaviors in a stable and familiar context.6,7 Rothwell and colleagues7 studied a man with severe sensory neuropathy in the upper extremity. He could write sentences with his eyes closed and drive a car with a manual transmission without watching the gear shift. He did, however, have difficulty with fine motor tasks such as buttoning his shirt and using a knife and fork to eat when denied visual information. The importance of sensory information must be weighed by the individual, unconsciously filtering and choosing appropriate and accurate sensory inputs to use to meet the movement goal.
Sensory experiences and learning alter sensory representations, or cortical “maps,” in the primary somatosensory, visual, and auditory areas of the brain. Training, as well as use and disuse of sensory information, has the potential to drive long-term structural changes in the CNS, including the formation, removal, and remodeling of synapses and dendritic connections in the cortex. This process of cortical plasticity is complex and involves multiple cellular and synaptic mechanisms.9 Plasticity in the nervous system is discussed further in the third section of this chapter.
Coordination
The movement plan is customized by communications among the frontal lobes, basal ganglia, and cerebellum, with functional connections through the brain stem and thalamus. During this process specific details of the plan are determined. Postural tone, coactivation, and timing of trunk muscle firing are set for proximal stability, balance, and postural control. Force, timing, and tone of limb synergies are set to allow for smooth, coordinated movements that are accurate in direction of trajectory, order, and sequence. The balance between agonist and antagonist muscle activity is determined so that fine distal movements are precise and skilled. This process is complicated by the number of possible combinations of musculoskeletal elements. The CNS must solve this “degrees of freedom” problem so that rapid execution of the goal-directed movement can proceed and reliably meet the desired outcome.10 Once these movement details are complete the motor plan is executed by the primary motor area in the precentral gyrus of the frontal lobe.
Adaptation
Adaptation is the process of using sensory inputs from multiple systems to adapt motor plans, decrease performance errors, and predict or estimate consequences of movement choices. The goal of adaptation is the production of consistently effective and efficient skilled motor actions. When all possible body systems and environmental conditions are considered in the motor control process, it is easy to understand why there is often a mismatch between the movement plan that is chosen and how it is actually executed. Errors in movements occur and cause problems that the nervous system must solve in order to deliver effective, efficient, accurate plans that meet the task goal. To solve this problem the CNS creates an internal representation of the body and the surrounding world. This acts as a model that can be adapted and changed in the presence of varying environmental demands. It allows for the ability to predict and estimate the differences between similar situations. This ability is learned by practicing various task configurations in real-life environments. Without experience, accurate movement patterns that consistently meet desired task goals are difficult to achieve.11
Anticipatory control
Anticipatory control of posture and postural adjustments stabilizes the body by minimizing displacement of the center of gravity. Anticipatory control involves motor plans that are programmed to act in advance of movement. A comparison between incoming sensory information and knowledge of prior movement successes and failures enables the system to choose the appropriate course of action.3
Flexibility
A person should have enough flexibility in performance to vary the details of a simple or complex motor plan to meet the challenge presented by any given environmental context. This is a beneficial characteristic of motor control. When considering postural control, for example, a person will typically display a random sway pattern during standing that may ensure continuous, dynamic sensory inputs to multiple sensory systems.12 The person is constantly adjusting posture and position to meet the demand of standing upright (earth vertical), as well as to seek information from the environment. Rhythmic, oscillating, or stereotypical sway patterns that are unidirectional in nature are not considered flexible and are not as readily adaptable to changes in the environment. Lack of flexibility or randomness in postural sway may actually render the person at greater risk for loss of balance and falls.
Control of voluntary movement
Table 4-2 shows the body system processes involved in motor control, their actions, and the body structures included. The following section explains these processes in more detail.
TABLE 4-2
PROCESS | ACTION | BODY STRUCTURES INVOLVED |
Sensation | Sensory information, feedback from exteroceptors and proprioceptors | Peripheral afferent neurons, brain stem, cerebellum, thalamus, sensory receiving areas in the parietal, occipital, and temporal lobes |
Perception | Combining, comparing, and filtering sensory inputs | Brain stem, thalamus, sensory association areas in the parietal, occipital, visual, and temporal lobes |
Choice of movement plan | Use of the perceptual map to access the appropriate motor plan | Association areas, frontal lobe, basal ganglia |
Coordination | Determining the details of the plan including force, timing, tone, direction, and extent of the movement of postural and limb synergies and actions | Frontal lobe, basal ganglia, cerebellum, thalamus |
Execution | Execution of the motor plan | Corticospinal and corticobulbar tract systems, brain stem motor nuclei, and alpha and gamma motor neurons |
Adaptation | Compare movement with the motor plan and adjust the plan during performance | Spinal neural networks, cerebellum |
Role of the cerebellum
The primary roles of the cerebellum are to maintain posture and balance during static and dynamic tasks and to coordinate movements before execution and during performance. The cerebellum processes multiple neural signals from (1) motor areas of the cerebral cortex for motor planning, (2) sensory tract systems (dorsal spinal cerebellar tract, ventral spinal cerebellar tract) from muscle and joint receptors for proprioceptive and kinesthetic sense information resulting from movement performance, and (3) vestibular system information for the regulation of upright control and balance at rest and during movements. It compares motor plan signals driven by the cortex with what is received from muscles and joints in the periphery and makes necessary adjustments and adaptations to achieve the intended coordinated movement sequence. Movements that are frequently repeated “instructions” are stored in the cerebellum as procedural memory traces. This increases the efficiency of its role in coordinating movement. The cerebellum also plays a role in function of the reticular activating system (RAS). The RAS network exists in the brain stem tegmentum and consists of a network of nerve cells that maintain consciousness in humans and help people focus attention and block out distractions that may affect motor performance. Damage to the cerebellum, its tract systems, or its structure creates problems of movement coordination, not execution or choice of which program to run. The cerebellum also plays a role in language, attention, and mental imagery functions that are not considered to take place in motor areas of the cerebral cortex (see Table 4-2).
The cerebellum plays four important roles in motor control13:
1. Feed-forward processing: The cerebellum receives neural signals, processes them in a sequential order, and sends information out, providing a rapid response to any incoming information. It is not designed to act like the cerebral cortex and does not have the capability of generating self-sustaining neural patterns.
2. Divergence and convergence: The cerebellum receives a great number of inputs from multiple body structures, processes this information extensively through a structured internal network, and sends the results out through a limited number of output cells.
3. Modularity: The cerebellum is functionally divided into independent modules—hundreds to thousands—all with different inputs and outputs. Each module appears to function independently, although they each share neurons with the inferior olives, Purkinje cells, mossy and parallel fibers, and deep cerebellar nuclei.
4. Plasticity: Synapses within the cerebellar system (between parallel fibers and Purkinje cells, and synapses between mossy fibers and deep nuclear cells) are susceptible to modification of their output strength. The influence of input on nuclear cells is adjustable, which gives great flexibility to adjust and fine-tune the relationship between cerebellar inputs and outputs.
Role of the basal ganglia
The basal ganglia are a collection of nuclei located in the forebrain and midbrain and consisting of the globus pallidus, putamen, caudate nucleus, substantia nigra, and subthalamic nuclei. It has primary functions in motor control and motor learning. It plays a role in deciding which motor plan or behavior to execute at any given time. It has connections to the limbic system and is therefore believed to be involved in “reward learning.” It plays a key role in eye movements through midbrain connections with the superior colliculus and helps to regulate postural tone as a basis for the control of body positions, preparedness, and central set. Refer to Chapter 20 for additional information on the basal ganglia.
Information processing
The processing of information through the sensory input, motor output, and central integrative structures occurs by various methods to produce movement behaviors. These methods allow us to deal with the temporal and spatial components necessary for coordinated motor output and allow us to anticipate so that a response pattern may be prepared in advance. Serial processing is a specific, sequential order of processing of information (Figure 4-1) through various centers. Information proceeds lockstep through each center. Parallel processing is processing of information that can be used for more than one activity by more than one center simultaneously or nearly simultaneously. A third and more flexible type of processing of information is parallel-distributed processing.14 This type of processing combines the best attributes of serial and parallel processing. When the situation demands serial processing, this type of activity occurs. At other times parallel processing is the mode of choice. For optimal processing of intrinsic and extrinsic sensory information by various regions of the brain, a combination of both serial and parallel processing is the most efficient mode. The type of processing depends on the constraints of the situation. For example, maintaining balance after an unexpected external perturbation requires rapid processing, whereas learning to voluntarily shift the center of gravity to the limits of stability requires a different combination of processing modes.
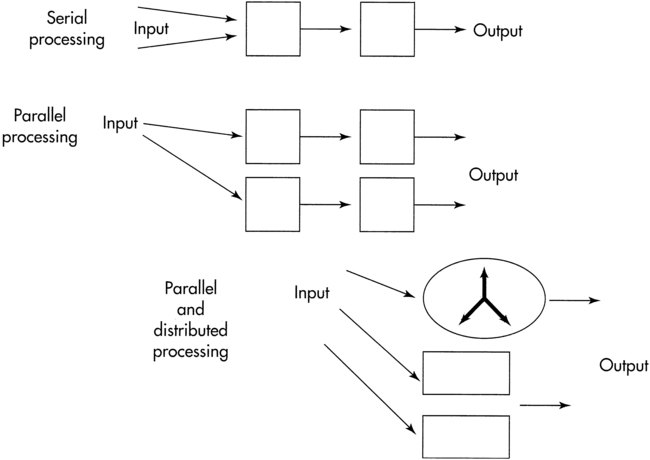

Movement patterns arising from self-organizing subsystems
Coordinated movement patterns are developed and refined via dynamic interaction among body systems and subsystems in response to internal and external constraints. Movement patterns used to accomplish a goal are contextually appropriate and arise as an emergent property of subsystem interaction. Several principles relate to self-organizing systems: reciprocity, distributed function, consensus, and emergent properties.15
Reciprocity implies information flow between two or more neural networks. These networks can represent specific brain centers, for example, the cerebellum and basal ganglia (Figure 4-2). Alternatively, the neural networks can be interacting neuronal clusters located within a single center, for example, the basal ganglia. One model to demonstrate reciprocity is the basal ganglia regulation of motor behavior through direct and indirect pathways to cortical areas. The more direct pathway from the putamen to the globus pallidus internal segment provides net inhibitory effects. The more indirect pathway from the putamen through the globus pallidus external segment and subthalamic nucleus provides a net excitatory effect on the globus pallidus internal segment. Alteration of the balance between these pathways is postulated to produce motor dysfunction.16,17 An abnormally decreased outflow from the basal ganglia is postulated to produce involuntary motor patterns, which produce excessive motion such as chorea, hemiballism, or nonintentional tremor. Alternatively, an abnormally increased outflow from the basal ganglia is postulated to produce a paucity of motions, as seen in the rigidity observed in individuals with Parkinson disease (see Chapter 20).
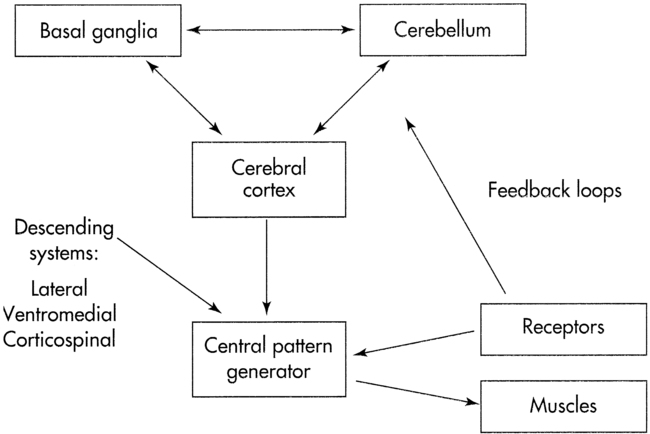

Distributed function presupposes that a single center or neural network has more than one function. The concept also implies that several centers share the same function. For example, a center may serve as the coordinating unit of an activity in one task and may serve as a pattern generator or oscillator to maintain the activity in another task. An advantage of distributing function among groups of neurons or centers is to provide centers with overlapping or redundant functions. Neuroscientists believe such redundancy is a safety feature. If a neuronal lesion occurs, other centers can assume critical functional roles, thereby producing recovery from CNS dysfunction.18–22
Emergent properties may be understood by the adage “the whole is greater than the sum of its parts.” This concept implies that brain centers, not a single brain center, work together to produce movement. An example of the emergent properties concept is continuous repetitive activity (oscillation). In Figure 4-3, A, a hierarchy is represented by three neurons arranged in tandem. The last neuron ends on a responder. If a single stimulus activates this network, a single response occurs. What is the response if the neurons are arranged so that the third neuron sends a collateral branch to the first neuron in addition to the ending on the responder? In this case (Figure 4-3, B), a single stimulus activates neuron No. 1, which in turn activates neurons No. 2 and No. 3, causing a response as well as reactivating neuron No. 1. This neuronal arrangement produces a series of responses rather than a single response. This process is also termed endogenous activity.
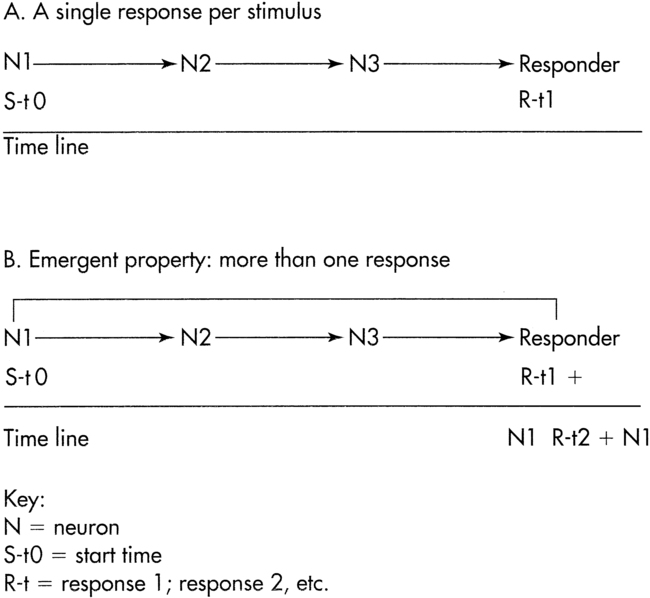

Controlling the degrees of freedom
Combinations of muscle and joint action permit a large number of degrees of freedom that contribute to movement. A system with a large number of degrees of freedom is called a high-dimensional system. For a contextually appropriate movement to occur, the number of degrees of freedom needs to be constrained. Bernstein10 suggested that the number of degrees of freedom could be reduced by muscles working in synergies, that is, coupling muscles and joints of a limb to produce functional patterns of movement. The functional unit of motor behavior is then a synergy. Synergies help to reduce the degrees of freedom, transforming a high-dimensional system into a low-dimensional system. For example, a step is considered to be a functional synergy pattern for the lower extremity. Linking together stepping synergies with the functional synergies of other limbs creates locomotion (interlimb coordination).
Functional synergy implies that muscles are activated in an appropriate sequence and with appropriate force, timing, and directional components. These components can be represented as fixed or “relative” ratios, and the control comes from input given to the cerebellum from higher centers in the brain and the peripheral or spinal system and from prior learning (see Chapter 21).20,22,23 The relative parameters are also termed control parameters. Scaling control parameters leads to a change in motor behavior to accomplish the task. For example, writing your name on the blackboard exemplifies scaling force, timing, and amplitude. Scaling is the proportional increase or decrease of the parameter to produce the intended motor activity.
Timing of muscle on/off activation for antagonistic muscles such as biceps and triceps, or hamstrings and quadriceps, needs to be accurate for coordination and control of movement patterns. If one muscle group demonstrates a delayed onset or maintains a longer duration of activity, overlapping with triceps “on” time, the movement will appear uncoordinated. Patients with neurological dysfunction often demonstrate alterations in the timing of muscle activity within functional synergies and in coupling functional synergies to produce movement.24,25 These functional movement synergies are not hardwired but represent emergent properties. They are flexible and adaptable to meet the challenges of the task and the environmental constraints.
Finite number of movement strategies
The concept of emergent properties could conceivably imply an unlimited number of movement strategies available to perform a particular task. However, limiting the degrees of freedom decreases the number of strategies available for selection. In addition, constraints imposed by the internal environment (e.g., musculoskeletal system, cardiovascular system, metabolic activity, cognition) and external environment (e.g., support surface, obstacles, lighting) limit the number of movement strategies. Horak and Nashner26 observed that a finite number of balance strategies were used by individuals in response to externally applied linear perturbations on a force plate system. With use of a life span approach, VanSant27 identified a limited number of movement patterns for the upper limb, head-trunk, and lower limb for the task of rising from supine to standing.
Variability of movements implies normalcy
Motivation to accomplish a task in spite of functional limitations and neuropathological conditions can also shift the individual’s CNS to select different patterns of motor behavior. The musculoskeletal system, by nature of the architecture of the joints and muscle attachments, can be a constraint on the movement pattern. An individual with a functional contracture may be limited in the ability to bend a joint only into a desired range, thereby decreasing the movement repertoire available to the individual. Such a constraint produces adaptive motor behavior. Dorsiflexion of the foot needs to meet a critical degree of toe clearance during gait. If there is a range of motion limitation in dorsiflexion, then biomechanical constraints imposed on the nervous system will produce adaptive motor behaviors (e.g., toe clearance during gait). Changes in motor patterns during the task of rising from supine to standing are observed when healthy individuals wear an orthosis to limit dorsiflexion.28 The inability to easily open and close the hand with rotation may lead to adaptations that require the shoulder musculature to place the hand in a more functional position. This adaptation uses axial and trunk muscles and will limit the use of that limb in both fine and gross motor performance. Refer to Chapter 23.
Preferred, nonobligatory movement patterns that are stable yet flexible enough to meet ever-changing environmental conditions are considered attractor states. Individuals can choose from a variety of movement patterns to accomplish a given task. For example, older adults may choose from a variety of fall-prevention movement patterns when faced with the risk of falling. The choice of motor plan may be negatively influenced by age-related declines in the sensory input systems or a fear of falling. For example, when performing the Multi-Directional Reach Test,29 an older adult may choose to reach forward, backward (lean), or laterally without shifting the center of gravity toward the limits of stability. This person has the capability of performing a different reaching pattern if asked, but prefers a more stable pattern.
Obligatory and stereotypical movement patterns also arise from external constraints imposed on the organism. Consider the external constraints placed on a concert violin player. These external constraints include, for example, the length of the bow and the position of the violin. Repetitive movement patterns leading to cumulative trauma disorder in healthy individuals can lead to muscular and neurological changes.30–33 Over time, changes in dystonic posturing and changes in the somatosensory cortex have been observed. Although one hypothesis considers that the focal dystonia results from sensory integrative problems, the observable result is a stereotypical motor problem.
Errors in motor control
Errors occur in the perception of sensory information, in selection of the appropriate MP, in selection of the appropriate variable parameters, or in the response execution. Patients with neurological deficits may demonstrate a combination of these errors. Therefore an assessment of motor deficits in clients includes analysis of these types of errors. If a therapist observes a motor control problem, there is no guarantee that the central problem arises from within the motor system. Somatosensory problems can drive motor dysfunction; cognitive and emotional problems express themselves through motor output. Thus it is up to the movement specialist to differentiate the cause of the problem through valid and reliable examination tools (see Chapter 8). Once the cause of the motor problem has been identified, selection of interventions should lead to more outcomes.
Motor control section summary
Motor control theories have been developed and have evolved over many years as our understanding of nervous system structure and function has become more advanced. The control of posture and movement is a complex process that involves many structures and levels within the human body. It requires accurate sensory inputs, coordinated motor outputs, and central integrative processes to produce skillful, goal-directed patterns of movement that achieve desired movement goals. We must integrate and filter multiple sensory inputs from both the internal environment of the body and the external world around us to determine position in space and choose the appropriate motor plan to accomplish a given task. We combine individual biomechanical and muscle segments of the body into complex movement synergies to deal with the infinite “degrees of freedom” available during the production of voluntary movement. Well learned motor plans are stored and retrieved and modified to allow for flexibility and variety of movement patterns and postures. When the PNS or CNS is damaged and the control of movement is impaired, new, modified, or substitute motor plans can be generated to accomplish goal-directed behaviors, remain adaptable to changing environments, and produce variable movement patterns. The process of learning new motor plans and refining existing behaviors by driving neuroplastic changes in the nervous system is discussed in the next sections of this chapter. The control of posture and balance is also discussed in Chapter 22.
Motor learning
Motor learning results in a permanent change in the performance of a skill because of experience or practice.34 The end result of motor learning is the acquisition of a new movement, or the reacquisition and/or modification of movement.35 The patient must be able to prepare and carry out a particular learned movement36 in a manner that is efficient (optimal movement with the least amount of time, energy, and effort),37 consistent (same movement over repeated trials),38 and transferrable (ability to perform movement under different environments and conditions) to be considered to have learned a skill.
Motor skills can be categorized as discrete, continuous, or serial. Discrete motor skills pertain to tasks that have a specific start and finish. Tasks that are repetitive are classified as continuous motor skills. Serial skills involve several discrete tasks connected in a particular sequence that rapidly progress from one part to the next.37 The category of a particular motor skill is a major factor in making clinical decisions regarding the person-, task-, and environment-related variables that affect motor learning. This is discussed later in the chapter.
An illustration of motor learning principles
Observe the sequential activities of the child walking off the park bench in Figure 4-4, A through C. A clear understanding of this relationship of walking and falling is established. In frame A, the child is running a feed-forward program for walking. The cerebellum is procedurally responsible for modulating appropriate motor control over the activity and will correct or modify the program of walking when necessary to attain the directed goal. Unfortunately, a simple correction of walking is not adequate for the environment presented in frame B. The cerebellum has no prior knowledge of the feedback presented in this second frame and thus is still running a feed-forward program for stance on the left leg and swing on the right leg. The cerebellum and somatosensory cortices are processing a massive amount of mismatched information from the proprioceptive, vestibular, and visual receptors. In addition, the dopamine receptors are activated during the goal-driven behaviors, creating a balance of inhibition and excitation. Once the executive or higher cognitive system recognizes that the body is falling (which has been experienced from falling off a chair or bed), a shift in motor control focus from walking to falling must take place. To prepare for falling, the somatosensory system must generate a sensory plan and then relay that plan to the motor system through the sensorimotor feedback loops. The frontal lobe will tell the basal ganglia and the cerebellum to brace and prepare for impact. The basal ganglia are responsible for initiating the new program, and the cerebellum carries out the procedure, as observed in Figure 4-4, C. The child succeeds at the task and receives positive peripheral and central feedback in the process. It is possible that this experience has created a new procedural program that in time will be verbally labeled “jumping.” The entire process of the initial motor learning takes 1 to 2 seconds. Because of the child’s motivation and interest (see Chapter 5), the program is practiced for the next 30 to 45 minutes. This is the initial acquisition phase and helps the nervous system store the MP to be used for the rest of the child’s life. If this program is to become a procedural skill, practice must continue within similar environments and conditions. Ultimately the errors will be reduced and the skill will be refined. Finally, with practice, the program will enter the retention phase as a high-level skill. The skill can be modified in terms of force, timing, sequencing, and speed and is transferrable to different settings. This ongoing modification and improvement are the hallmarks of true procedural learning. Modifications within the program will be a function of the plasticity that occurs within the CNS throughout life as the child ages and changes body size and distribution. Similar plasticity and the ability to change, modify, and reprogram motor plans will be demanded by individuals who age with chronic sensorimotor limitations. Unfortunately, in many of these individuals, the CNS is not capable of producing and accommodating change, which creates new challenges as they age with long-term movement dysfunctions (see Chapters 27, 32, and 35).
Stages of motor learning
Several authors have developed models to describe the stages of motor learning. These models are presented in Table 4-3. Regardless of the model, it is widely accepted that the process of learning a motor task occurs in stages. During the initial stages of learning a motor skill, the intent of the learner is to understand the task. To be able to develop this understanding requires a high level of concentration and cognitive processing. In the middle and later stages, the individual learns to refine the movement, improve efficiency and coordination, and perform the skill within different environmental contexts. The later stages are characterized by automaticity and a decreased level of attention needed for successful completion of the task. It is important to emphasize early that because the activities performed by a learner during each stage of learning will be different, the role of the clinician, the types of learning activities, and the clinical environment must also be different.
TABLE 4-3
STAGES OF MOTOR LEARNING—THREE MODELS
MOTOR LEARNING MODEL | STAGE ONE | STAGE TWO | STAGE THREE |
Fitts and Posner (1967)39 | Cognitive | Associative | Autonomous |
Bernstein (1967)10 | Novice | Advanced | Expert |
Gentile (1998)46 | Acquire the plan | Develop consistency and adaptability |
The learning model described by Fitts and Posner39 consists of a continuous progression through three stages: cognitive, associative, and autonomous.
Focused practice with repetition over time leads to the automatic performance of motor skills in the autonomous stage of learning. The individual is in control of the learned movement plan and is able to use it with little cognitive attention while involved in other activities. Skills are performed with preferred, appropriate, and flexible speed, amplitude, direction, timing, and force. Consistency of performance is a hallmark of this phase, as is the ability to detect and self-correct performance errors. Individuals who do not have the cognitive skill to remember the learning can go through a much longer repetitive practice schedule to learn the motor skill, but there will be very little carryover into other functional movements or activities.40–42
In summary, the overall process of the stages of motor learning as introduced by Fitts and Posner39 suggests that first a basic understanding of a task be established, along with a motor pattern. Practice of the task then leads to problem solving and a decrease in the degrees of freedom during performance, resulting in improved coordination and accuracy. As the learner continues to practice and solves the motor task problem in different ways and with different physical and environmental constraints, the movement plan becomes more flexible and adaptable to a wide range of task demands.
Bernstein10 presented a more biomechanical perspective as he addressed the problem of degrees of freedom during motor learning. He also broke the motor learning process down into three stages: novice, advanced, and expert. He proposed that these three stages are necessary to allow a learner to reduce the large number of degrees of freedom that are inherent in the musculoskeletal system, including structure and function of muscles, tendons, joints. He proposed that as a person learns a new motor skill, he or she gains coordination and control over the multiple interacting variables that exist in the human body to master the target skill.
In Bernstein’s expert stage, degrees of freedom are now released and reorganized to allow the body to react to all of the internal and external mechanisms that may act on it at any given time. At the same time, enhanced coactivation of proximal structures is learned and used to allow for greater force, speed, and dexterity of limb movements.43
Gentile presented a two-stage model of motor learning.44,45 She considered motor learning from the goal of the learner and strongly considered how environmental conditions influence performance and learning.
Stage one requires the client to problem solve strategies to get the idea of a movement and establish a motor pattern that will successfully meet the demands of the task. As with the models presented previously, this process demands conscious attention to the components of the task and environmental variables to formulate a “map” or framework of the movement pattern. Once this framework is established, the client has a mechanism for performing the task; however, errors and inconsistency in performance accuracy are often present.46
Variables that affect motor learning
The ecological model (constraints theory) of motor control and learning states that motor learning involves the person, the task, and the environment.47 For a purposeful and functional movement to occur, the individual must generate movement to successfully meet the task at hand, as well as the demands of the environment where the task must be performed. For motor learning to be successful, several variables related to each of these three constructs must be taken into account.
Variables related to the individual
The clinician must first differentiate general motor performance factors that are under the control of the individual’s cognitive and emotional systems and those that are controlled by the motor system itself. These concepts are presented in Figure 4-5. There are many cognitive factors such as arousal, attention, and memory, as well as cortical pathways related to declarative or executive learning, that have specific influences over behaviors that are observed after neurological insult.48,49 Other factors such as limbic connections to cortical pathways affected by motivation, fear and belief, and emotional stability and instability also dramatically affect motor performance and declarative learning. Some of these factors may also limit activity and participation. Therapists need to learn how to discriminate among motor output, somatosensory input, cortical processing, and limbic emotional state problems and identify how the latter two systems affect motor output. With that differentiation, clinicians should also be able to separate specific motor system deficits from motor control problems arising from dysfunction within other areas of the CNS. Last, the patient’s fitness level; current limitations in strength, endurance, power, and range of motion; or pain level may also influence learning.35,50–55
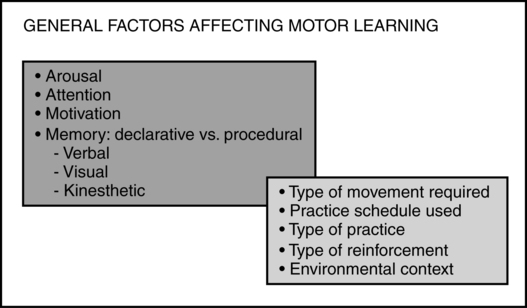

Variables related to the task
Practice.
Practice is defined as “repeated performance in order to acquire a “skill.”56 As the definition implies, several repetitions of the task are usually required to be able to achieve skillful performance of a task. With other variables being constant, more practice results in more learning.35 To be effective, these repetitions must involve a process of problem solving rather than just repetition of the activity.57 The therapist can manipulate several variables related to practice to optimize motor learning of an individual with a movement dysfunction secondary to a neurological insult.
Practice conditions.
According to apportionment of practice in relation to rest periods, massed tasks or exercises can be classified as massed practice or distributed practice. Massed practice is when the rest period is much shorter in relation to the amount of time the task or exercise is practiced.58 This is contrasted against distributed practice, in which the time between practice sets is equal to or greater than the amount of time devoted to practicing a particular task or activity, such that the rest period is spread out throughout the practice.59 In terms of neurological physical therapy practice, it is important to consider the effect of physical and mental fatigue when training. For example, physical fatigue sets in during massed practice of a particular balance exercise activity in standing and may cause a patient to fall. Moreover, individuals who are cognitively impaired may not respond positively to sustained activity that requires considerable concentration and therefore might fail in the performance of the skill. On the other hand, to be functional and useful in daily life, certain activities have to be performed without significant amounts of rest periods. For example, taking significant rest breaks when ambulating for even a short distance limits an individual’s ability to use walking in a functional manner. Sometimes a patient needs more rest periods in the initial stages of learning a skill to compensate for impairments in muscular endurance or cardiopulmonary function, with the intent of decreasing these rest periods to achieve skill performance that reflect how that activity is used in real-life situations. Therefore therapists should consider the skill demands and the desired results when choosing one practice type versus another.59
Complete tasks or activities can usually be divided into smaller subcomponents. The way those subcomponents are practiced relative to the entire task or activity can also be manipulated to optimize motor learning. To practice the entire task or parts of the task, whole learning, pure-part learning, progressive-part learning, or whole-part learning may be used. Figure 4-6 summarizes these concepts.
Whole-part learning can be used when the skill or activity can be practiced between the whole and the parts. In the clinical environment, a common application of this concept is whole to part to whole learning.60 First the therapist has the client try the whole activity, such as coming to stand or reaching out to turn the door handle. Next, the therapist has the client practice a component part. Finally the whole activity is practiced as a functional pattern. In this way therapists work on the functional activity, then work on correcting the impairment or limitation, such as power production, range, or balance, and then go back to the functional activity in order to incorporate the part learning into the whole. An example might be asking a patient to first stand up from a chair. As he tries to stand he generates too much power, holds his breath, and cannot repeat the activity more than once. The therapist decides to practice a component part by first assisting the patient to a relaxed standing posture, then having him eccentrically begin to sit into a partial squat, and then having him return to standing. As the patient practices, he will increase the range of lengthening and eventually will sit and return to stand. Once that is accomplished, he will continue to practice sit to stand to sit to stand as a whole activity.
According to the sequence in which component tasks are practiced, blocked or random practice may be used. In blocked practice the patient first practices a single task over and over before moving to the next task. On the other hand, in random practice, the component tasks are practiced without any particular sequence. The contextual interference effect explains the difference in motor performance found when comparing these two types of practice. Studies have shown performance may be enhanced by using blocked practice; however, learning is not enhanced by using this type of practice. Random practice has been shown to enhance learning because this type of practice forces the learners to come up with a motor solution each time a task is performed.61,62
Feedback.
KR pertains to feedback given at the conclusion of the task (therefore also called terminal feedback) and provides the patient information about the success of his or her actions with respect to the activity. KR can be classified as faded, delayed, or summary. In faded feedback the therapist provides more information in the beginning stages of learning of the skill and slowly withdraws that information as the patient demonstrates improvement in the performance of the task. With delayed feedback, information is given to the patient when a period of time has elapsed after the task has been completed. The intent of this pause between the termination of task and feedback is to give the patient some time to process the activity and generate possible solutions to the difficulties encountered in the previous performance of the task. In contrast, summary feedback is provided after the patient has performed several trials of a particular task without receiving feedback. Previous studies showed that subjects who were given more frequent feedback performed better during the task acquisition stage of learning but worse on retention tests compared with those who received summary feedback.63,64
Additional concepts related to long-term learning are presented in Figure 4-7.
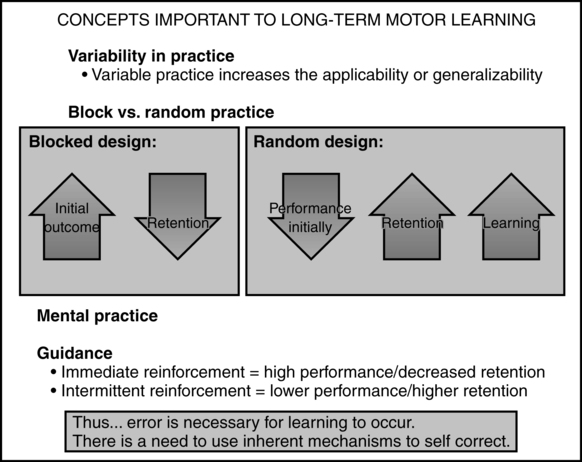

Variables related to the environment
Therapists can alter the environmental conditions to optimize motor learning. Gentile44,45 described the manipulation of the environment in which a task is performed to make an activity more appropriate for what the patient is able to do. A closed environment is stationary; it allows the patient to practice the skill in a predictable manner, with minimal distractions from the environment. On the other hand, an open environment is one that is in motion or unpredictable. In patients with neurological dysfunctions, clinicians may decide to have a patient practice a skill in a closed environment to allow the patient to plan the movement in advance and to perform the movement with minimal distractions or challenges. An example of this would be performing gait training in a quiet and empty therapy gym. As the patient improves, it may be important to practice this activity in an open environment to provide a real-world application of a task. Going back to the previous example, the therapist may have the patient ambulate in an open environment such as a busy gym with crowds and noise, a crowded cafeteria, or a moving walkway.
If prior procedural learning has occurred, then creating an environment that allows the program to run in the least restrictive environment should lead to the most efficient outcome in the shortest time.51,52 If a patient needs to learn a new program, such as walking with a stereotypical extension pattern, then goal-directed, attended practice with guided feedback is necessary. It may be easier to bring back an old ambulatory pattern by creating an environment to elicit that program than to teach a client to use a new inefficient movement program.53–55
If the client has permanent damage to either the basal ganglia or the cerebellum, then retaining the memory of new MPs may be difficult and substitution approaches may become necessary. Through evaluation the clinician needs to determine whether anatomical disease or a pathological condition is actually causing procedural learning problems and whether identifying and teaching a substitution pattern or teaching the patient to compensate with an old pattern will allow the individual to succeed at the task. However, therapists should never forget that the plasticity of the CNS can promote significant recovery and adaptation through the performance of attended, goal-directed, repetitive behavior.65,66
Systems interactions: motor responses represent consensus of central nervous system components
Motor behavior reflects not only motor programming but also the interaction of cognitive, affective, and somatosensory variables. Without a motor system, neither the cognitive nor the emotional systems have a way to express and communicate inner thoughts to the world. The cognitive and emotional systems can positively or negatively affect motor responses. The significance of the somatosensory or perceptual-cognitive cortical system must be emphasized. The somatosensory association areas play a critical role in the ideational and constructional aspects of the MP itself. When there are deficits within this system, clients will often demonstrate significant distortions in motor control even without a specific motor impairment. An example of this problem might be an individual who had a stroke and developed a “pusher syndrome.” The motor behavior shown by this client would be pushing off vertical generally in a lateral or posterolateral direction.67 Physically correcting the client’s posture to vertical or asking the patient to self-correct will not eliminate the original behavior. Pusher syndrome does not stem from a motor problem but rather from a perceptual problem of verticality from thalamic nuclei radiating false information to the somatosensory cortices. Although a therapist might want to augment intervention by trying to push the patient to vertical, the patient will resist that movement pattern. Functional training becomes frustrating to both the patient and the therapist because the impairment does not fall within the motor system itself. Reliance on the use of vision and environmental cues might be the best intervention strategy for this type of problem because the impairment is within the sensory processing centers.68 Asking the patient to find midline and reach across midline, then acknowledging success, along with a lack of falling help the somatosensory system to relearn and thus begin to inherently correct to vertical. Verbalizing to the patient that you (the therapist) acknowledge that she or he feels as if she or he is falling when placed in the vertical position demonstrates to the client that you have accepted the patient and his or her perceptions. Simultaneously maintaining tactile contact to prevent the patient from falling effectively lets the limbic system relax and reduces its need to trigger motor reactions. This example creates conflict between the cognitive system’s information from the thalamus and motor system feedback. The thalamus is saying vertical is “X,” and the motor system is saying “if X then I am falling.” When the goal is not to fall, then the cognitive system will generally override the thalamic information and learn to accept a new concept of vertical. Taking all these variables into the treatment environment optimizes the potential that the patient will self-correct during a functional activity such as reaching with weight shift.
Principles of neuroplasticity: implications for neurorehabilitation
Rehabilitation, research, and practice
Rehabilitation is the process of maximizing functional learning. The integration of basic neuroscience into clinical practice is critical for guiding the questioning of researchers and maximizing the recovery of patients. The 1990s were referred to as the “Decade of the Brain.” For the last 20 years, researchers have made enormous advances in understanding the adaptability of the CNS. Because of this revolution, clinicians must focus on recovery rather than compensation. There is sufficient evidence that the CNS not only develops and matures during adolescence, but also recovers from serious disease and injury and maintains sensory, motor, and cognitive competency through spontaneous healing, appropriate medical management, physical exercise, balanced nutrition, and learning. Across the life span, individuals can maximize independence and quality of life by taking advantage of learning from enriched environments, task-specific training, and attended, progressive, goal-oriented, repetitive behaviors. In addition, the nervous system can adapt negatively to repetitive and abnormal patterns of movement based on structural anomalies, pain, abnormal biomechanics, or bad habits (see the section on motor learning in this chapter).
The paradigm shift in rehabilitative intervention strategies based on neuroplasticity has just begun. Basic science researchers cannot ignore the impact of their findings on the health and function of the consumer. Clinical researchers must collaborate in clinical studies to determine the impact of basic science findings with patients.69–71 Clinicians cannot simply provide the same, familiar treatment of yesterday because it is comfortable and easy and requires minimal effort. Physical therapy professionals must be dynamic, enthusiastic, evidence-based and committed to lifelong learning, ready to accept the challenge and unique opportunity to work with other members of the health care team to translate neuroscience to practice. Failure to translate basic science findings into clinical practice will significantly impair the potential for patient recovery.
During the last 45 years, three large conferences72–74 focused on these issues in neuroscience. In 1966 the Northwestern University Special Therapeutic Exercise Project (NUSTEP) conference in Chicago, Illinois, brought researchers, basic scientists, educators, and master clinicians together for 6 weeks to identify commonalities in approaches to interventions and to integrate basic science into those commonalities. A huge shift from specific philosophies to a bodily systems model occurred in 1990 at Norman, Oklahoma, the site of the Second Special Therapeutic Exercise Project conference (II STEP). During the next 15 years, concepts of motor learning and motor control were beginning to affect the methodology and intervention philosophies of both occupational and physical therapy. Simultaneously, newer approaches such as locomotion training with partial weight bearing on a treadmill,75,76 task-specific training,77,78 constraint-induced movement training,79,80 neuroprotective effect of exercise,81 mental and physical practice,82,83 patient-centered therapy,84–86 and sensorimotor training87 were frequently seen in peer-reviewed literature. The third STEP conference, Summer Institute on Translating Evidence into Practice (III STEP), occurred in July 2005 in Salt Lake City, Utah. At this conference, unique clinical models for intervention were embraced that will direct professional education for decades. Changes in practice over the next 15 years will lead to embracing many older intervention techniques with current evidence-based practice.
There are a variety of challenges to implementing effective, neuroscience-based interventions. The first is the patient. Patient-centered therapy is critical for effective therapeutic outcomes. The patient can be both the obstacle to successful recovery88,89 and the critical link to success.90,91 To achieve optimum neural adaptation, the patient must be engaged in attended, goal-directed, novel, progressive behaviors. There is no measurable neural adaptation with passive movements or passive stimuli. For a change in neural response to be achieved, the stimulus needs to be novel or a surprise and the individual has to attend to the stimulus, make a decision about what to do, and receive some feedback regarding the appropriateness or accuracy of the outcome.92 This progressive decision making has to be done repetitively and progressed in difficulty over time. These behaviors may be difficult to achieve when a person is depressed, feels hopeless, lacks motivation or cognition, or has emotional instability or there is neglect of one or more parts of the body.
Another obstacle to bringing scientific evidence into practice is the barrier created by living in a society in which the economics of health care rather than the science or the patient benefits drive the delivery of services (see Chapter 10). When a physician or a therapist recommends a new approach to intervention, the third-party payer may deny payment for service because it is “experimental.” Furthermore, third-party payers may deny the opportunity to apply findings from animal studies to human subjects. Another example of constraint from the third-party payer is the timing of intervention. Despite the evidence that the CNS can be modified under conditions of goal-oriented, repetitive, task-relevant behaviors even years poststroke, insurance companies deny coverage of service late in the recovery process. The insurance company may interpret “medically necessary services” as the services provided during the first 30 days postinjury, the time after a cerebrovascular accident when the greatest spontaneous recovery occurs. Furthermore, even though neural adaptation research confirms that enriched environmental conditions and sensory inputs can facilitate both greater and continued recovery, the insurance company may claim that the services93–95 are simply for maintenance. Thus, as the science of neuroplasticity continues to develop, it is critical to improve the interface among the scientist, the practitioner, the patient, and the third-party payer. Clinicians and researchers must regularly inform third-party payers about current research evidence.
Integration of sensory information in motor control
The sensory system provides an internal representation of both the inside and outside worlds to guide the movements that make up our behavioral repertoire. These movements are controlled by the motor systems of the brain and the spinal cord. Our perceptual skills are a reflection of the capabilities of the sensory systems to detect, analyze, and estimate the significance of physical stimuli. (See the section on augmented therapeutic intervention in Chapter 9 for a detailed discussion of each sensory system.) Our agility and dexterity represent a reflection of the capabilities of the motor systems to plan, coordinate, and execute movements. The task of the motor systems in controlling movement is the reverse of the task of sensory systems in generating an internal representation. Perception is the end product of sensory processing, whereas an internal representation (an image of the desired movement) is the beginning of motor processing.
Sensory psychophysics looks at the attributes of a stimulus: its quality, intensity, location, and duration. Motor psychophysics considers the organization of action, the intensity of the contraction, the recruitment of distinct populations of motor neurons, the accuracy of the movements, the coordination of the movements, and the speed of movement. In both the sensory and motor systems, the complexity of behaviors depends on the multiplicity of modalities available. In sensation, there are the distinct modalities of pain, temperature, light touch, deep touch, vibration, and stretch, whereas in the motor system can be found the modalities of reflex responses, rhythmic motor patterns within and between limbs, automatic and adaptive motor responses, and voluntary fine and gross movements.96–116 Although all motor movements require integration of sensory information for motor learning, once motor control is attained the system can run on very little feedback. The relationship of incoming sensory information is particularly complex in voluntary motor movements that constantly adapt to environmental variance. For voluntary motor movements, the motor system requires contraction and relaxation of muscles, recruitment of appropriate muscles and their synergies, appropriate timing and sequencing of muscle contraction and relaxation, the distribution of the body mass, and appropriate postural adjustments. As stated, once an MP is learned, it does not take the same amount of sensory information to run the program in a feed-forward manner within the motor system as long as the information to the cerebellum is able to run and adjust all aspects of the program. (See Chapter 21 and the section on motor control in this chapter.) To learn new programs, the CNS must go through the process of receipt of sensory input, perceptual processing, communication with the frontal lobes, and relays to basal ganglia and cerebellum, followed by intentional, goal-directed execution of the motor plan.
Within each movement, there must be adjustments to compensate for the inertia of the limbs and the mechanical arrangement of the muscles, bones, and joints both before and during movement to ensure and maintain accuracy. The control systems for voluntary movement include (1) the continuous flow of sensory information about the environment, position, and orientation of the body and limbs and the degree of contraction of the muscles; (2) the spinal cord; (3) the descending systems of the brain stem; and (4) the pathways of the motor areas of the cerebral cortex, cerebellum, and basal ganglia. Each level of control is based on the sensory information that is relevant for the functions it controls. This information is provided by feedback, feed-forward, and adaptive mechanisms. These control systems are organized both hierarchically and in parallel. These systems also control activation of sensations and motor movements as well as inhibition (e.g., globus pallidus). Furthermore, some parts of the brain are needed for new learning (e.g., cerebellum) and others for maintained learning (e.g., globus pallidus, hippocampus). The hierarchical but interactive organization permits lower levels to generate reflexes without involving higher centers, whereas the parallel system allows the brain to process the flow of discrete types of sensory information to produce discrete types of movements.117,118
Ultimately, the control of graded fine motor movements involves the sensory organ of the muscle, the muscle spindle, which contains the specialized elements that sense muscle length and the velocity or changes in spindle length. In conjunction with the tendon organ, which senses muscle tension, the muscle spindle provides the CNS with continuous information on the mechanical state of the muscle. Ultimately the firing of the muscle spindles depends on both muscle length and the level of gamma motor activation of the intrafusal fibers. Similarly, joint proprioceptors relay both closed and open chain input and mobility (range) information from within the joint structures to the CNS. This illustrates the close relationship between sensory and motor processing and the integral relationship between the two.119
Foundation for the study of neuroplasticity
The principal models for studying cortical plasticity have been based on the representations of hand skin and hand movements in the New World owl monkey (Aotus) and the squirrel monkey (Saimiri). These primate models have been chosen because their central sulci usually do not extend into the hand representational zone in the anterior parietal (S1) or posterior frontal (M1) cortical fields. In other primates the sulci are deep and interfere with accurate mapping. Albeit there are differences in hand use among primates, in all of the primates the hand has the largest topographical representation for the actual size of the extremity, the detail of this representation is distinct, and the hand has the greatest potential for skilled movements and sensory discrimination. However, the findings from studies of this cortical area are applicable across the different cortices as well as the other cornerstones of the brain such as the thalamus, basal ganglia, brain stem, and cerebellum.120,121 See Figure 4-8 to identify specific anatomical locations and their respective classifications.
To understand neural adaptation and to be able to apply the principles to practice, it is necessary to objectively measure the changes. Positive changes in neural structure can be measured by using a variety of imaging techniques (e.g., magnetic resonance imaging [MRI], functional MRI [fMRI], magnetoencephalography, magnetic source imaging [MSI]). The types of outcomes that can be expected electrophysiologically and functionally are summarized in Table 4-4. At this time, imaging techniques are applied primarily for research purposes or to rule out other pathology. The specific type of intervention to address the principles of neuroplasticity may vary, but the outcomes must be clearly documented.
TABLE 4-4
NEUROPROTECTIVE MOTOR ENRICHMENT FACTORS AFFECTING OUTCOMES
NEGATIVE PLASTICITY | POSITIVE PLASTICITY | |
Stimulation | Disuse, unskilled | Intensive, skilled |
Quality of sensory input | Noisy, nonspecific | Appropriate, specific |
Modulation | Not challenging | High stakes, novel, challenging |
Outcome | Negative behaviors | Positive behaviors |
Principles of neural adaptation
To achieve maximum neural adaptation, there are some basic principles to follow (Box 4-1). Learning is the key to neural adaptation. Plasticity is the mechanism for encoding, the changing of behaviors, and both implicit and explicit learning. During neural adaptation, the fundamental questions are as follows: As we learn, how does the brain change its representations of inputs and actions? What is the nature of the processes that control the progressive elaboration of performance abilities? In different individuals, what are the sources of variance for emergence of improved performance? What changes in cortical plasticity facilitate the development of “automatic” motor behaviors? Why are some behaviors hard to change? What limits plasticity processes? What are the critical elements of brain circuitry, genes, synapses, neural chemistry, neuronal networks, and neural connections for restoration of lost function? What guidelines need to be followed to drive the greatest change in brain structure and function? How do spontaneous compensatory behavioral strategies contribute to or interfere with restoring lost neuronal function? How does the unaffected side contribute to or interfere with neuroplastic changes and restoration of function? Does damage to the brain alter the neuronal response to learning (e.g., cascade of cellular activity for healing altered circuitry, new neural connections)?
1. Neural circuits must be actively engaged in learning-based activities if degradation and atrophy are to be prevented.
We know that if infants are deprived of sensory and motor experiences during development, the brain does not develop normally. For example, without exposure to light, there is a reduction in the number of neurons in the visual cortex.122 Similarly, if infants are not exposed to sound, there is a reduction in the neurons in the auditory cortex.123 Even in adults, when neural circuits are not used over an extended period of time, they begin to degrade, and the unused area of the brain is allocated to serve another part of the body.124 Similarly, if task performance is practiced, then the topography expands and becomes more detailed, as might occur in someone who is blind and reads Braille.125 It is also interesting to note that although a person is blind, the visual cortical areas may become active when the individual is reading Braille.126 Similarly a person who is deaf may demonstrate activation of the auditory cortex when visual stimuli are presented.
2. With learning, the distributed cortical representations of inputs and brain actions “specialize” in their representations of behaviorally important inputs and actions in skill learning.
There seems to be a minimal level of repetitive practice needed to acquire a new skill that will be maintained over time. In fact, this may lead to specialization or change in the underlying neurophysiological processing.127–129 This specialization develops in response to selective cortical neuron responses specialized to demands of sensory, perceptual, cognitive, and motor skill learning.130–133 This adaptation has been clearly documented in animal studies. For example, if an animal is trained to make progressively finer distinctions about specific sensory stimuli, then cortical neurons come to represent those stimuli in a progressively more specific and progressively “amplified” manner.
3. There are important behavioral conditions that must be met in the learning phase of plasticity.
a. If behaviorally important stimuli repeatedly excite cortical neuron populations, the neurons will progressively grow in number.
b. Repetitive, behaviorally important stimuli processed in skill learning lead to progressively greater specificity in the spectral (spatial) and temporal dimensions.
4. The growing numbers of selectively responding neurons discharge with progressively stronger temporal coordination (distributed synchronicity).
Through the course of progressive skill learning, a more refined basis for processing stimuli and generating actions critical to skilled tasks is enabled by the multidimensional changes in cortical responses. Consequently, specific aspects of these changes in distributed neuronal response are highly correlated with learning-based improvements in perception, motor control, and cognition.134–137 In these processes the brain is not simply changing to record and store content, but the cerebral cortex is also selectively refining its processing capacities to fit each task at hand by adjusting its spectral or spatial and temporal filters. Ultimately it establishes its own general processing capabilities. This “learning to learn” determines the facility with which specific classes of information can be stored, associated, and manipulated. These powerful self-shaping processes of the forebrain machinery are operating not only on a large scale during development but also during experience-based management of externally and internally generated information in adults. This self-shaping with experience allows the development of hierarchical organization of perception, cognition, motor, and executive management skills.
5. In learning, selection of behaviorally important inputs is a product of strengthening input coincidence-based connections (synapses).
a. A progressive amplification of cell numbers engaged by repetitive inputs.136–138
b. An increase in the temporal coordination of distributed neuronal discharges evoked by successive events to mark features of behaviorally important inputs is a consequence of a progressive increase in positive coupling between nearly simultaneously engaged neurons within cortical networks.136,139
c. A progressively more specific “selection” of all input features that collectively represent behaviorally important inputs, expressed moment by moment in time.138,139 Thus skill learning results in mapping temporal neighbors in representational networks at adjacent spatial locations when they regularly occur successively in time.65,140,141 Changes in activation patterns, dendritic growth, synapses, and neuronal activities may also be observed.
The basis of the functional creation of the detailed, representational cortical maps converting temporal to spatial representations is related to the Hebbian change principle.142 The Hebbian plasticity principle applies to the development of interconnections between excitatory and inhibitory inputs within the cortical pyramidal neurons and their connections to extrinsic inputs and outputs. On the basis of the Hebbian principle, the operation of coincidence-based synaptic plasticity in cortical networks results in the formation, strengthening, and continuous recruitment of neurons within neuronal “assemblies” that “cooperatively” represent behaviorally important stimuli.
6. Plasticity is constrained by anatomical sources and convergent-divergent spreads of inputs. Every cortical field has the following:
7. Plasticity is constrained by the time constants governing coincident input co-selection and by the time structures and potentially achievable coherence of extrinsic and intrinsic cortical input sources.
To effectively drive representational changes with coincident input-dependent Hebbian mechanisms, temporally coordinated inputs are prerequisite, given the short durations (milliseconds to tens of milliseconds) of the time constants that govern synaptic plasticity in the adaptive cortical machinery (see reference 145 for review). Consistently uncorrelated or low–discharge-rate inputs induce negative changes in synaptic effectiveness. In addition, stimuli occurring repetitively simultaneously can also degrade the representation. These negative effects also contribute importantly to the learning-driven “election” of behaviorally important inputs.
8. Cortical field–specific differences in input sources, distributions, and time-structured inputs create different representational structures.
a. There are significant differences in the activity from afferent inputs from the retina, skin, or cochlea generated in a relatively strictly topographically wired V1 (area 17), S1 proper (area 3b), or A1 (area 43) compared with the inferotemporal visual, insular somatosensory, dorsotemporal auditory, or prefrontal cortical areas that receive highly diffuse inputs (see Figure 4-8). In the former cases, heavy schedules of repetitive, temporally coherent inputs are delivered from powerful, redundant projections from relatively strictly topographically organized thalamic nuclei and lower-level, associated cortical areas. Whereas neighboring neurons can share some response properties, neurons or clusters of neurons respond selectively to learned inputs. These neurons are distributed widely across cortical areas and share less information with neighboring neurons. In the “lower” levels, afferent input projections from any given source are greatly dispersed. Highly repetitive inputs are uncommon, inputs from multiple diffuse cortical sources are more common as well as more varied, and complex input combinations are in play. These differences in input schedules, spreads, and combinations presumably largely account for the dramatic differences in the patterns of representation of behaviorally important stimuli at “lower” and “higher” levels.146
b. Despite these differences in representational organization across the cortex, the cortex does progressively differentiate cortical cells to accomplish specific operational tasks. There is a serial progression of differentiation to allow the development of functional organization that allows an individual to progressively master more and more elaborated and differentiated perceptual, cognitive, monitoring, and executive skills.
c. The sources of inputs and their field-specific spreads and boundary limits, the distributions of modulatory inputs differentiated by cortical layers in different cortical regions, the basic elements and their basic interconnections in the cortical processing machine, and crucial aspects of input combination and processing at subcortical levels are inherited (see reference 147 for review). Although these inherited aspects of sensory, motor, and cortical processing circuit development constrain the potential learning-based modification of processing within each cortical area, representation changes can occur as a result of environmental interaction and purposeful behavioral practice.
9. Temporal dimensions of behaviorally important inputs also influence representational “specialization.” In at least four ways, the cortex refines its representations of the temporal aspects of behaviorally important inputs during learning.
1) The cortex generates more synchronous representations of sequenced and coincident associative input perturbations or events, not only recording their identities but also marking their occurrences (for examples, see references 132, 136, 139, and 148 to 151). These changes in representation appear to be primarily achieved through increases in positive coupling strengths between interconnected neurons participating in stimulus- or action-specific neuronal cell assemblies.132,150,152–171 The strength of the interconnectedness increases representational salience as a result of downstream neurons being excited as a direct function of the degree of temporal synchronization of their inputs.
2) Increasing the power of the outputs of a cortical area drives downstream plasticity. Hebbian plasticity mechanisms operating within downstream cortical (or other) targets also have relatively short time constants. The greater the synchronicity of inputs, the more powerfully those change mechanisms are engaged. The strength of the interconnections also helps protect against noise. For example, by simple information abstraction and coding, the distributed neuronal representation of the “signal” (a temporally coordinated, distributed neuronal response pattern representing the input or action) is converted at the entry levels in the cortex into a form that is not as easily degraded or altered by “noise.” The strength of the interconnectedness also confers robustness of complex signal representation for spatially or spectrally incomplete or degraded inputs.
1) The cortex can select specific inputs through learning to exaggerate the representation of specific input time structures. Conditioning a monkey or a rat with stimuli that have a consistent, specific temporal modulation rate or interstimulus time, for example, results in a selective exaggeration of the responses of neurons at that rate or time separation. In effect, the cortex “specializes” for expected relatively higher-speed or relatively lower-speed signal event reception.
2) Both electrophysiological recording studies and theoretical studies suggest that cortical networks richly encode the temporal interval as a simple consequence of cortical network dynamics.172,173 It is hypothesized that the cortex accomplishes time interval and duration selectivity in learning by positively changing synaptic connection strengths for input circuits that can respond with recovery times and circuit delays that match behaviorally important modulation frequency periods, intervals, or durations. However, studies on including excessive, rapid, repetitive fine motor movements can sometimes lead to serious degradation in representation if the adjacent digits are driven nearly simultaneous in time. This may be associated with negative learning and a loss of motor control.174
1) The cortex links representations of immediately successive inputs that are presented in a learning context.
2) As a result of Hebbian plasticity, it establishes overlapping and neighboring relationships between immediately successive parts of rapidly changing inputs yet retains its individualized, distinct cortical representation. 65,175
1) The cortex generates stimulus sequence-specific (“combination-sensitive”) responses, with neuronal responses selectively modulated by the prior application of stimuli in the learned sequence of temporally separated events.
2) These “associative” or “combination-sensitive” responses have been correlated with evidence of strengthened interconnections between cortical cell assemblies representing successive event elements separated by hundreds of milliseconds to seconds in time.176,177 The mechanisms of origin of these effects have not yet been established.
10. The integration time (“processing time”) in the cortex is itself subject to powerful learning-based plasticity.
a. Cortical networks engage both excitatory and inhibitory neurons by strong input perturbations. Within a given processing “channel,” cortical pyramidal cells cannot be effectively reexcited by a following perturbation for tens to hundreds of milliseconds. These integration “times” are primarily dictated by the time for recovery from inhibition, which ordinarily dominates poststimulus excitability. This “integration time,” “processing time,” or “recovery time” is commonly measured by deriving a “modulation transfer function,” which defines the ability of cortical neurons to respond to identical successive stimuli within cortical “processing channels.” For example, these “integration” times normally range from about 15 to about 200 ms in the primary auditory receiving areas.178–180 Progressively longer processing times are recorded at higher system levels (e.g., in the auditory cortex, they are approximately a syllable in length, 200 to 500 ms in duration) in the “belt cortex” surrounding the primary auditory cortex.181
b. These time constants govern—and limit—the cortex’s ability to “chunk” (i.e., to separately represent by distributed, coordinated discharge) successive events within its processing channels. Both neurophysiological studies in animals and behavioral training studies in human adults and children have shown that the time constants governing event-by-event complex signal representation are highly plastic. With intensive training in the right form, cortical “processing times” reflected by the ability to accurately and separately process events occurring at different input rates can be dramatically shortened or lengthened.182–185
11. Plasticity processes are competitive.
a. If two spatially or spectrally different inputs are consistently delivered nonsimultaneously to the cortex, cortical networks generate input-selective cell assemblies for each input and actively segregate them from one another.139,184,186–188 Boundaries between such inputs grow to be sharp and are substantially intensity independent. Computational models of Hebbian network behaviors indicate that this sharp segregation of nonidentical, temporally separated inputs is accomplished as a result of a wider distribution of inhibitory instead of excitatory responses in the emerging, competing cortical cell assemblies that represent them.
b. This Hebbian network cell assembly formation and competition appear to account for how the cortex creates sharply sorted representations of the fingers in the primary somatosensory cortex.140,189 The Hebbian network probably accounts for how the cortex creates sharply sorted representations of native aural language-specific phonemes in lower-level auditory cortical areas in the auditory and speech processing system of humans. If inputs are delivered in a constant and stereotyped way from a limited region of the skin or cochlea in a learning context, that skin surface or cochlear sector is an evident competitive “winner.”136,190 By Hebbian plasticity, the cortical networks will co-select that specific combination of inputs and represent it within a competitively growing Hebbian cell assembly. The competitive strength of that cooperative cell assembly will grow progressively because more and more neurons are excited by behaviorally important stimuli with increasingly coordinated discharges. That means that neurons outside of this cooperative group have greater numbers of more coordinated outputs contributing to their later competitive recruitment. Through progressive functional remodeling, the cortex clusters and competitively sorts information across sharp boundaries dictated by the spectrotemporal statistics of its inputs. If it receives information on a heavy schedule that sets up competition for a limited input set, it will sort competitive inputs into a correspondingly small number of largely discontinuous response regions.191,192
c. Competitive outcomes are, again, cortical level dependent. The cortex links events that occur in different competitive groups if they are consistently excited synchronously in time. At the same time, competitively formed groups of neurons come to be synchronously linked in their representations of different parts of the complex stimulus and collectively represent successive complex features of the vocalization through the coordinated activities of many groups.
d. Neurons within the two levels of the cortex surrounding A1 (see Figure 4-8) have greater spectral input convergence and longer integration times that enable their facile combination of information representing different spectrotemporal details. Their information extraction is greatly facilitated by the learning-based linkages of cooperative groups that deliver behaviorally important inputs in a highly salient, temporally coordinated form to these fields. With their progressively greater space and time constants, still higher-level areas organize competitive cell assemblies that represent still more complex spectral and serial-event combinations. Note that these organizational changes apply over a large cortical scale. In skill learning over a limited period of training, participating neuronal members of such assemblies can easily be increased by many hundredfold, even within a primary sensory area such as S1, area 3b, or A1.136,139,174,184,193
e. In extensive training in complex signal recognition, more than 10% of neurons within temporal cortical areas can come to respond highly selectively to a specific, normally rare, complex training stimulus. The distributed cell assemblies representing those specific complex inputs involve tens or hundreds of millions of neurons and are achieved by enduring effectiveness changes in many billions of synapses.
12. Learning is modulated as a function of behavioral state.
a. At “lower” levels of the cortex, changes are generated only in attended behaviors.137,138,146,193–195 Trial-by-trial change magnitudes are a function of the importance of the input to the animal as signaled by the level of attention, the cognitive values of behavioral rewards or punishments, and internal judgments of practice trial precision or error based on the relative success or failure of achieving a target goal or expectation. Little or no enduring change is induced when a well-learned “automatic” behavior is performed from memory without attention. It is also interesting to note that at some levels within the cortex, activity changes can be induced even in nonattending subjects under conditions in which “priming” effects of nonattended reception of information can be demonstrated.
b. The modulation of progressive learning is also achieved by the activation of powerful reward systems releasing the neurotransmitters norepinephrine and dopamine (among others) through widespread projections to the cerebral cortex. Norepinephrine plays a particularly important role in modulating learning-induced changes in the cortex.148,184,195
c. The cortex is a “learning machine.” During the learning of a new skill, neurotransmitters are released trial by trial with application of a behaviorally important stimulus or behavioral rewards. If the skill can be mastered and thereafter replayed from memory, its performance can be generated without attention (habituation). Habituation results in a profound attenuation of the modulation signals from these neurotransmitter sources; plasticity is no longer positively enabled in cortical networks.
13. Top-down influences constrain cortical representational plasticity.
Attentional control flexibly defines an enabling “window” for change in learning.182 Progressive learning generates progressively more strongly represented goals, expectations, and feedback196,197 across all representational systems that are undergoing change and to modulatory control systems weighing performance success and error. Strong intermodal behavioral and representational effects have also been recorded in experiments that might be interpreted as shaping expectations.198,199 These shaping expectations would be similar to those observed in a human subject using multisensory inputs such as auditory, visual, and somesthetic information to create integrated phonological representations, to create fine motor movement trajectory patterns that underlie precise hand control, or to make a vocal production.
14. The scale of plasticity in progressive skill learning is massive.
a. Cortical representational plasticity must be viewed as arising from multiple-level systems that are broadly engaged in learning, perceiving, remembering, thinking, and acting. Any behaviorally important input (or consistent internally generated activity) engages many cortical areas. Repetitive training drives all cortical areas to change.131,144,200 Different aspects of any acquired skill are contributed from field-specific changes in the multiple cortical areas that are remodeled in its learning.
b. In this kind of continuously evolving representational machine, perceptual constancy cannot be accounted for by locationally constant brain representations; relational representational principles must be invoked to account for it.131,201 Moreover, representational changes must obviously be coordinated level to level. It should also be understood that plastic changes are also induced extracortically. Although it is believed that learning at the cortical level is usually predominant, plasticity induced by learning within many extracortical structures significantly contributes to learning-induced changes that are expressed within the cortex.
15. Enduring cortical plasticity changes appear to be accounted for by local changes in neural anatomy.
Changes in synapse turnover, synapse number, synaptic active zones, dendritic spines, and the elaboration of terminal dendrites have been demonstrated to occur in a behaviorally engaged cortical zone.144,202–207 Through many changes in local structural detail, the learning brain is continuously physically remodeling its processing machinery, not only across the course of child development but also after behavioral training in an adult who has had a neural insult.
16. Cortical plasticity processes in child development represent progressive, multiple-staged skill learning.
a. There are two remarkable achievements of brain plasticity in child development. The first is the progressive shaping of the processing to handle the accurate, high-speed reception of the rapidly changing streams of information that flow into the brain. In the cerebral cortex, shaping appears to begin most powerfully within the primary receiving areas of the cortex. With early myelination, the main gateways for information into the cortex are receiving strongly coherent inputs from subcortical nuclei, and they can quickly organize their local networks on the basis of coincident input co-selection (Hebbian) plasticity mechanisms. The self-organization of the cortical processing machinery spreads outward from these primary receiving areas over time to ultimately refine the basic processing machinery of all the cortex. The second great achievement, which is strongly dependent on the first, is the efficient storage of massive content compendia in richly associated forms.
b. During development, the brain accomplishes its functional self-organization through a long parallel series of small steps. At each step, the brain masters a series of elementary processing skills and establishes reliable information repertoires that enable the accomplishment of subsequent skills. Second- and higher-order skills can be viewed as both elaborations of more basic mastered skills and the creation of new skills dependent on combined second- and higher-order processing. That hierarchical processing is enabled by greater cortical anatomical spreads, by more complexly convergent anatomical sources of inputs, and by longer integration (processing, recovery) times at progressively higher cortical system levels. This hierarchical but integrating processing allows for progressively more complex combinations of information integrated over progressively longer time epochs as one ascends across cortical processing hierarchies.
c. As the cortical machinery functionally evolves and consequently physically “matures” through childhood developmental stages, information repertories are represented in progressively more salient forms (i.e., with more powerful distributed response coordination). Growing agreement directly controls the power of emerging information repertoires for driving the next level of elaborative and combinatorial changes. It is hypothesized that saliency enables the maturation of the myelination of projection tracts delivering outputs from functionally refined cortical areas. More mature myelination of output projections also contributes to the power of this newly organized activity to drive strong, downstream plastic change through the operation of Hebbian plasticity processes.
d. As each elaboration of skill is practiced, in a learning phase, neuromodulatory transmitters enable change in the cortical machinery. The cortex functionally and physically adapts to generate the neurological representations of the skill in progressively more selective, predictable, and statistically reliable forms. Ultimately, the performance of the skill concurs with the brain’s own accumulated, learning-derived “expectations.” The skill can then be performed from memory, without attention. With this consolidation of the remembered skill and information repertoire, the modulatory nuclei enable no further change in the cortical machinery. The learning machine, the cerebral cortex, moves on to the next elaboration. In this way the cortex constructs highly specialized processing machinery that can progressively produce great towers of automatically performable behaviors and great progressively maturing hierarchies of information-processing machinery that can achieve progressively more powerful complex signal representations, retrievals, and associations. With this machinery in a mature and thereby efficiently operating form, there is a remarkable capacity for reception, storage, and analysis of diverse and complexly associated information.
e. The flexible, self-adjusting capacity for refinement of the processing capabilities of the nervous system confers the ability of our species to represent complex language structures. This self-adjusting capacity also allows humans to develop high-speed reading abilities; remarkably varied complex modern-era motor abilities; and abstract logic structures characteristic of a mathematician, software engineer, or philosopher. This nervous system refinement also creates elaborate, idiosyncratic, experience-based behavioral abilities in all of us.
Neuroplasticity and learning
How are learning sequences controlled? what constrains learning progressions?
A second powerful basis for sequenced learning is progressive myelination. At the time of birth, only the core “primary” extrinsic information entry zones (A1, S1, V1) in the cortex are heavily myelinated.208,209 Across childhood, connections to and interconnections between cortical areas are progressively myelinated, proceeding from these core areas out to progressively “higher” system levels. Myelination in the posterior parietal, anterior, and inferior temporal and prefrontal cortical areas is not “mature” in the human forebrain until 8 to 20 years of age. Even in the mature state, it is far less developed at the “highest” processing levels.
Although myelination is thought to be genetically programmed, some scientists hypothesize that myelination in the CNS is also controlled by emerging temporal response coherence and is achieved through temporally coordinated signaling from the multiple branches of oligodendrocytes that terminate on different projection axons in central tracts and networks. It has been argued that central myelination is positively and negatively activity dependent and that distributed synchronization may contribute to positive change.210 If the hypothesis that coherent activity controls myelination proves to be true, then the emerging temporal correlation of distributed representations of behaviorally important stimuli is generated level by level. This is done by changes in coupling in local cortical networks in the developing cortex. It would also directly drive changes in myelination for the outputs of that cortical area. These two events in turn would enable the generation of reliable and salient representational constructs at that higher level. By this kind of progression, skill learning is hypothesized to directly control progressive functional and physical brain development through the course of child development. This is accomplished both by refining (“maturing”) local interconnections through response dynamics of information processing machinery at successive cortical levels and by coordinated refinement (“maturing”) of the critical information transmission pathways that interconnect different processing levels.
Another constraint in the development of neural adaptation may be the development of mature sleeping patterns, especially within the first year of life.211 Sleep both enables the strengthening of learning-based plastic changes and resets the learning machinery by “erasing” temporary unreinforced and unrewarded input-generated changes produced over the preceding waking period.212–214 The dramatic shift in the percentage of time spent in rapid-eye-movement sleep is consistent with a strong early bias toward noise removal in an immature and poorly functionally unorganized brain. Sleep patterns change dramatically in the older child, in parallel with a strong increase in the daily schedule of closely attended, rewarded, and goal-oriented behaviors. This research will need to be explored in greater detail when these data are related to patients with CNS damage. This population often has poor breathing habits and capabilities that lead to decreased oxygenation and often broken sleep cycles. How much either impairment, breakdown, or the interaction of the two diminishes neuroplasticity has yet to be determined.
What facilitates the development of permanent “automatic” motor behaviors?
The creation and maintenance of cortical representations are functions of the animal’s or human’s level of attention at a task. Cortical representational plasticity in skill acquisition is self-limiting. Because the behavior comes to be more “automatic,” it is less closely attended, and representational changes induced in the cortex fade and ultimately disappear or reverse (unlearning effects).215,216 The element of behavioral performance that enables maintenance of the behavior with minimum involvement of the cortical learning machinery is probably stereotypical movement sequence repetition. As a movement behavior is practiced, an effective, highly statistically predictable movement sequence is adopted that enables the storage of the learned behavior in a permanent form that requires only minimal or no behavioral attention. If behavioral performance declines or behavioral or brain conditions change to render a task more difficult, attention to the behavior will again need to increase, producing an invigorated cortical response to the new learning challenge.
By this view, the cerebral cortex is clearly a learning machine. William James217 was the first to point out that the great practical advantage for a self-organizing cortex was the development of what he called “habits.” When a skill is overlearned, it will engage pathways that are so reliable that they can be followed without attention.