CHAPTER 32 Connective Tissues
Animals use different proportions of matrix macromolecules to construct connective tissues with a range of mechanical properties to support their organs. Bone is a stiff, hard solid; blood vessel walls are flexible and elastic; and the vitreous body of the eye is a watery gel. Plant cell walls are conceptually similar to the animal extracellular matrix but are composed of completely different molecules. This chapter begins with a discussion of simple connective tissues but concentrates on cartilage, bone, development of the skeleton, and the mechanisms that repair wounds, finishing with a discussion of the plant cell wall.
Loose Connective Tissue
Loose connective tissue consists of a sparse extracellular matrix of hyaluronan and proteoglycans supported by a few collagen fibrils and elastic fibrils. In addition to fibroblasts, the cell population is heterogeneous, including both indigenous and emigrant connective tissue cells (see Fig. 28-3). The loose connective tissue underlying the epithelium in the gastrointestinal tract is a good example of this heterogeneity (Fig. 32-1A), with lymphocytes, plasma cells, macrophages, eosinophils, neutrophils, and mast cells, as well as fibroblasts and occasional fat cells (see Chapter 28 for details on these cells). This variety of defensive cells is appropriate for a location near the lumen of the intestine, which contains microorganisms and potentially toxic materials from the outside world. Loose connective tissue is also found in and around other organs. The optically transparent vitreous body of the eye is an extremely simple loose connective tissue in which fibroblasts produce a highly hydrated gel of hyaluronan and proteoglycans, supported by a loose network of type II collagen. Few defensive cells are required, as the interior of the eye is sterile.
Dense Connective Tissue
Collagen fibers, with or without elastic fibers, predominate over cells in dense connective tissue (Fig. 32-1B). Fibroblasts are present to manufacture extracellular matrix but are relatively sparse. Other connective tissue cells are even rarer, as these tissues are not usually exposed to microorganisms. Collagen fibers can be arranged precisely, as in tendons or cornea (see Fig. 29-3), or less so, as in the wall of the intestine or the skin. Tendons consist nearly exclusively of type I collagen fibers, all aligned along the length of the tendon to provide the tensile strength that is required to transmit forces from muscle to bone. The cornea that forms the transparent front surface of the eye is also well organized into orthogonal layers of collagen fibrils.
Dense connective tissues can also be elastic. For example, the walls of arteries (see Fig. 29-8) and the dermal layer of skin consist of both collagen and elastic fibers. Energy from each heartbeat stretches the elastic fibers in the walls of arteries. Recoil of these elastic fibers propels blood between heartbeats.
Cartilage
Cartilage (Fig. 32-2) is tough, resilient connective tissue that is well suited for a variety of mechanical roles. It covers the articular surfaces of joints and supports large airways, such as the trachea, and skeletal appendages, such as the nose and ears. Cartilage also forms the entire skeleton of sharks and the embryonic precursors of many bones in higher vertebrates. The mechanical properties of cartilage are attributable to abundant extracellular matrix consisting of fine collagen fibrils and high concentrations of glycosaminoglycans and proteoglycans (Fig. 32-3).
Chondrocytes synthesize and secrete macromolecules for the cartilage matrix, which eventually surrounds them completely. Chondrocytes replenish the matrix as the macromolecules turn over slowly, but their ability to remodel and repair the matrix is limited. No blood vessels penetrate cartilage, owing to production of several inhibitors of endothelial cell growth by chondrocytes. Thus, all nutrients must diffuse into cartilage from the nearest blood vessel in the perichondrium, a dense capsule of fibrous connective tissue that covers the surface of cartilage. This capsule contains mesenchymal stem cells (see Box 41-1) that are capable of differentiating into chondrocytes.
Glycosaminoglycans, including hyaluronan, constitute the second major class of matrix macromolecules. Molecules of the proteoglycan aggrecan attach to a hyaluronan backbone like the bristles of a test tube brush, forming so-called megacomplexes (see Fig. 29-14). Aggrecan also binds type II collagen. Highly charged glycosaminoglycans fill the extracellular space and attract water, the most abundant component of the matrix.
A hydrostatic mechanism allows cartilage to resist deformation (Fig. 32-3). Collagen fibrils provide tensile strength (i.e., resistance to stretching) but do not resist compression or bending. Glycosaminoglycans strongly attract water, resulting in an internal swelling pressure that pushes outward against collagen fibrils aligned parallel to the surface of the cartilage. The force of internal hydrostatic swelling pressure is balanced by the force produced by tension on the collagen fibrils. Remarkably, this internally stressed material can resist strong external forces such as those on the articular surfaces of joints. A macroscopic analog is a thin-walled plastic bottle filled with water. One can stand on the bottle provided that it is sealed, whereas neither the empty bottle nor the water could separately support any weight.
Differentiation and Growth of Cartilage
Many growth factors cooperate to influence the differentiation of precursor cells into chondrocytes, the proliferation of chondrocytes, and the production of cartilage matrix molecules. These include Indian hedgehog (Ihh), members of transforming growth factor-b family (TGF-b and bone morphogenetic factors), fibroblast growth factors (FGFs), parathyroid hormone–related protein (PTHrP), and insulin-like growth factors (IGF-I and IGF-II). Chondrocytes produce some of these growth factors (TGF-b, FGFs, and IGFs). During development, adjacent tissues can induce cartilage formation by secreting TGF-b and FGF. SOX9 is the key transcription factor mediating expression of cartilage-specific genes.
Diseases Involving Cartilage
Cartilage fails in common human diseases, including arthritis and ruptured intervertebral disks. Mutations in the genes for cartilage proteins and growth factors cause human disease (Appendix 32-1). Chondrocytes fail to proliferate in the absence of PTHrP or certain receptors for FGF, causing severe deformities of the skeleton. More than 25 different mutations of the human gene for type II collagen cause disorders of cartilage, ranging in severity from death in utero to dwarfism or osteoarthritis. Mutations in genes for minor cartilage collagens cause a variety of symptoms, including degenerative joint disease. A premature stop codon in chicken aggrecan causes lethal skeletal malformations.
Bone
For most vertebrates, bones provide mechanical support and serve as a storage site for calcium. The great strength and light weight of bones are attributable both to the mechanical properties of the extracellular matrix and to efficient overall design, including tubular form and lamination (Fig. 32-4). A superficial layer of compact bone surrounds a medullary cavity that is filled with marrow, fat, or both and is supported by struts of bone arranged precisely along lines of mechanical stress. External surfaces of bones are covered either by dense connective tissue, called periosteum, or by cartilage at joint surfaces. A monolayer of bone-forming cells called osteoblasts line the internal surfaces. Blood vessels supply the medullary cavity and penetrate compact bone through a network of channels. Although bone is durable and strong, continuous remodeling makes bone much more dynamic than it appears.
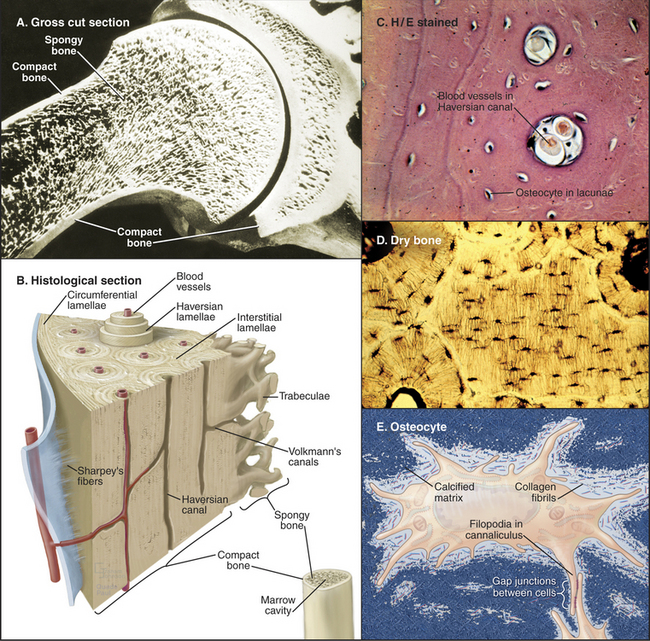
Figure 32-4 organization of long bones. A, Longitudinal section of a shoulder joint of a dried bone specimen. Struts of trabecular spongy bone reinforce compact bone in the cortex.B, A wedge of long bone. Circumferential lamellae form the outer layer just beneath the periosteum (blue) covering the surface. Osteons (Haversian systems) consist of concentric lamellae of calcified matrix and osteocytes arranged around a channel containing one or two capillaries or venules. Interstitial lamellae are fragments of osteons that remain after remodeling (Fig. 32-10). Radial vascular channels connect longitudinal vascular channels to the medullary cavity or periosteum. C, Light micrograph of a cross section stained with hematoxylin-eosin showing circumferential lamellae on the left and two Haversian canals. D, Light micrograph of a cross section of dried bone showing a central interstitial lamella surrounded by three osteons. Narrow canaliculi connect the lacunae housing osteocytes. E, An osteocyte surrounded by calcified matrix and extending filopodia into canaliculi.
(Micrographs courtesy of D. W. Fawcett, Harvard Medical School, Boston, Massachusetts.)
Extracellular Matrix of Bone
Bone is a composite material consisting of collagen fibrils (providing tensile strength) embedded in a matrix of calcium phosphate crystals (providing rigidity) (Fig. 32-4E). Macroscopic analogs of the bone matrix are concrete reinforced by steel rods and fiberglass consisting of a brittle plastic reinforced by glass fibers. Each of these composites is stronger than its separate components. Simple extraction experiments illustrate the contributions of the two components. After removal of calcium phosphate with a calcium chelator, bone is so rubbery that it bends easily. After destruction of collagen by heating, bone is hard but brittle.
Fibrils of type I collagen, the dominant organic component of the matrix (Table 32-1), are arranged in sheets or a meshwork. Covalent cross-links between the collagen molecules in fibrils make them inextensible. The matrix contains more than 100 minor proteins, including growth factors and adhesive glycoproteins, but few proteoglycans.
Name | Content | Functions |
---|---|---|
Bone morphogenic proteins | Minor | TGF-β homologs; cartilage stimulation and bone development and repair |
Collagen type I | 90% | Forms fibrils in the bone matrix |
Osteocalcin | 1%–2% | Network of aspartic acid and γ-carboxylated glutamic acid side chains bind hydroxyapatite; promotes calcification; attracts osteoclasts and osteoblasts |
Osteonectin | 2% | Synthesized in developing and regenerating bone; binds collagen and hydroxyapatite; may nucleate hydroxyapatite crystallization in bone matrix |
Osteopontin | Minor | RGD sequence; binds osteoclast integrins to bone surface |
Proteoglycans | Minor | Decorin, biglycan, osteoadherin; may bind TGF-β |
Sialoproteins | 2% | RGD sequence; binds osteoclast integrins to bone surface |
Bone Cells
Bone is an active tissue that is maintained by a balance of cellular activities. Osteoblasts and osteocytes produce extracellular matrix and establish conditions for its calcification. Osteoclasts resorb bone, as is required for growth and remodeling. An imbalance of these opposing cellular activities causes human diseases. Osteoblasts arise from the same mesenchymal stem cells that give rise to fibroblasts and chondrocytes (see Fig. 28-3). Osteoclasts form by fusion of blood monocytes.
A monolayer of osteoblasts on the surface of growing bone tissue uses a well-developed secretory pathway to synthesize and secrete organic components of the matrix (Fig. 32-5). Unmineralized bone matrix consists largely of type I collagen but includes factors that promote crystallization of calcium phosphate on the surface of these fibrils. Osteoblasts also control the differentiation, but not the activity, of osteoclasts (see Fig. 32-6).
Once an osteoblast has enclosed itself within bone matrix, it is called an osteocyte. Osteocytes are connected to each other by long, slender filopodia that run through narrow channels in the matrix (see Fig. 32-4D-E). Gap junctions between the processes of osteocytes provide a continuous network of intercellular communication that stretches from cells adjacent to blood vessels to the most deeply embedded osteocyte. Osteocytes can lay down or resorb matrix in their immediate vicinity.
Osteoblasts differentiate from mesenchymal cells under the control of growth factors, including Indian hedgehog, bone morphogenetic proteins (BMPs; see Fig. 24-8), and Wnts (see Fig. 30-8). Humans with loss-of-function mutations in a Wnt coreceptor have few osteoblasts and low bone density, while loss-of-function mutations in a BMP competitor have the opposite effect. Inside osteoblasts, Runx2/Cbfa1 is the master transcription factor controlling the expression of genes that are required to make bone matrix. Mouse embryos lacking Runx2/Cbfa1 have no osteoblasts or osteoclasts. They make a cartilage skeleton that never transforms to bone. Humans and mice with just one active Runx2/Cbfa1 gene lack collarbones and experience a delay in the fusion of joints between skull bones. This syndrome is the most common human skeletal defect. Runx2/Cbfa1 is part of a network of transcription factors with positive and negative influences on osteoblast differentiation and function.
Osteoclasts are multinucleated giant cells specialized for bone resorption (Fig. 32-6). They attach like a suction cup to the surface of bone. Interactions of a plasma membrane integrin (aVb3) with bone matrix proteins (osteopontin and sialoprotein) help to create a leak-proof compartment on the bone surface. Osteoclasts amplify the plasma membrane lining this closed space, forming a “ruffled border” composed of microvilli enriched with a V-type H+ transporting adenosine triphosphatase (ATPase, see Fig. 8-5) and chloride channels (see Fig. 10-13). The combined activities of the H+ pump and chloride channels allow the cell to secrete hydrochloric acid into the sealed extracellular compartment on the bone surface. This closed space acts like an extracellular lysosome: Acid dissolves calcium phosphate crystals, and secreted proteolytic enzymes, including cathepsin K, digest collagen and other organic components. Degradation products are taken up by endocytosis and transported across the cell in vesicles for secretion on the free surface. Amino acids are reused, but collagen cross-linking groups are not, so they are excreted in the urine, where their concentration is a measure of bone turnover.
Bone marrow supporting cells, osteoblasts, and activated T lymphocytes produce two proteins, which stimulate blood monocytes to fuse and differentiate into multinucleated osteoclasts (Fig. 32-6). These key factors are macrophage colony–stimulating factor (M-CSF) and RANKL (RANK ligand, also called osteoprotegerin ligand [OPGL] or TRANCE). Both factors are produced locally in bone marrow as transmembrane proteins with the growth factor domain on the cell surface. These proteins control differentiation through binding to their receptors by either direct cell-to-cell contact or release of the active domain by proteolytic cleavage. First, M-CSF activates a cytokine receptor (see Fig. 24-6) on macrophages. The resulting stimulation of a JAK-STAT pathway (see Fig. 27-9) turns on expression of genes required for the monocyte to differentiate into a preosteoclast. An important change is the expression of a receptor called RANK (receptor for activation of NF-kB, a member of the TNF receptor family; see Fig. 24-10). Once this receptor is expressed, RANKL can activate preosteoclasts through the transcription factor NF-kB (see Fig. 15-22C) to express the proteins that are required for cell fusion and further differentiation into an osteoclast. Mice that lack RANKL form no osteoclasts, so bone resorption fails.
Formation and Growth of the Skeleton
Both genetic and environmental information direct formation of the skeleton. Genetic information predominates in the master plan and initial development of skeletal tissues, as the size and shape of bones are characteristic for each species. Subsequently, environmental information is important in remodeling of the skeleton in response to use. Mutations in genes for structural and informational molecules have provided valuable clues about the genetic blueprint for the skeleton, but the understanding of these complex regulatory pathways is far from complete (Appendix 32-1).
Genetic information is read out on at least two levels. First, master genetic regulators—including transcription factors encoded by HOX (homeobox) and PAX (paired box) genes—specify the developmental fate of each embryonic segment. Homeoboxes are DNA sequences that encode a family of 60-residue protein domains that bind DNA (see Fig. 15-17). The human genome contains 39 HOX genes arrayed in four linear arrays, similar to those in other animals, including flies and nematodes. HOX genes were discovered in flies as a result of mutations that cause “homeotic conversion,” whereby the fate of one segment is converted into another, sometimes with bizarre results, such as the substitution of a leg for an antenna. The same thing happens in vertebrates: Mouse embryos express Hoxd-4 in the second cervical (neck) vertebra and more posterior segments. Mutation of Hoxd-4 results in a failure of the second cervical vertebra to form normally. Instead, it takes on some of the features of the first cervical vertebra. Mutations in other HOX genes cause congenital malformations in humans. HOX transcription factors control the expression of downstream genes, including growth factors, but the pathways from HOX genes to determinants of three-dimensional architecture are incompletely understood.
Second, systematically circulating and locally secreted growth factors control the proliferation and differentiation of the cells of cartilage and bone. Mutations in these factors and their receptors also cause surprisingly specific human skeletal malformations. Circulating growth hormone produced by the pituitary gland is a major determinant of skeletal size. Individuals who are deficient in growth hormone are short in stature. Locally produced growth factors, including bone morphogenetic proteins (BMPs) and fibroblast growth factors (FGFs) and their receptors, control the development and growth of cartilage and bone during embryogenesis, in addition to stimulating repair after fractures. FGF receptors are tyrosine kinases. BMPs are related in structure and mechanism to TGF-b and are expressed in tissues other than bone and cartilage (see Fig. 24-8). BMPs are part of a system of positive and negative factors that regulates formation of cartilage, bone, and joints. For example, a BMP called GDF-5 specifies the position of joints, but joints form only if noggin protein, an inhibitor of other BMPs, is present.
Embryonic Bone Formation
Bone always forms by replacement of preexisting connective tissue. During embryonic development, flat bones, such as the skull and shoulder blades, form from neural crest cell precursors in loose connective tissue (Fig. 32-7). Somehow, one-dimensional information in the genome is read out as the three-dimensional pattern of a skull. Growth factors, vitamins (e.g., retinoic acid), and local matrix molecules, such as glycosaminoglycans all influence the differentiation of these cells into osteoblasts at specific locations in connective tissue. Osteoblasts lay down struts of bone matrix in the loose connective tissue. As new bone is laid down on the surface of these bone spicules, some osteoblasts are trapped and become osteocytes. A similar process heals fractures.
During embryonic and postnatal development, ge-netic information precisely controls changes in the size and proportions of flat bones. For example, for the skull to increase in size both externally and internally, osteoclasts on the outer surface lay down new bone at the same rate at which osteoclasts resorb old bone inside (Fig. 32-9). These cellular activities are carefully coordinated to change the proportions of the skull as the individual matures.
Long bones, such as the humerus, begin as cartilage models that are replaced by bone (Fig. 32-8). The initial steps are only vaguely understood at the cellular and molecular levels. Multiple, genetically programmed factors induce clusters of mesenchymal cells at specific locations to differentiate into chondrocytes that secrete type II collagen and glycosaminoglycans. This produces a miniature cartilaginous version of the adult bone.
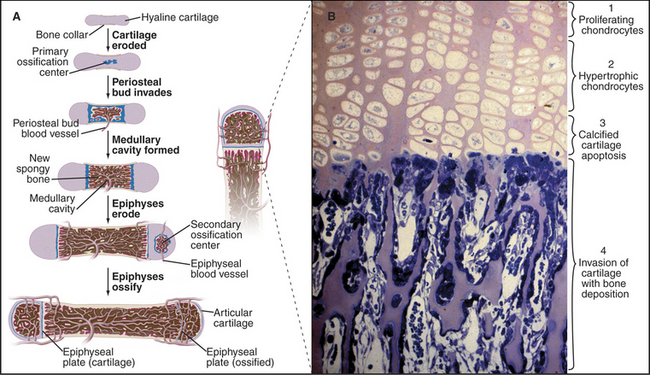
Figure 32-8 formation of a long bone by replacement of cartilage. A, The shaft grows in diameter as osteoblasts lay down bone (tan) on the outer surface of the primary collar of bone and osteoclasts remove bone from the inner surface to form and maintain the marrow cavity. The bone grows in length by interstitial expansion of the cartilage in the epiphyseal plate and its replacement by bone.B, Light micrograph of a section of an epiphyseal plate stained with toluidine blue. Cartilage growth, differentiation, and replacement by bone occur in several zones. Proliferation of chondrocytes and their production of matrix (pink) containing type II collagen are solely responsible for the longitudinal growth of the bone (1). Hypertrophic chondrocytes enlarge and make type X collagen, as well as matrix metalloproteinases that resorb some of the surrounding matrix (2). Chondrocytes die by apoptosis (see Chapter 46), and the matrix calcifies (3). Blood vessels and osteoblasts move into spaces vacated by chondrocytes and lay down bone (blue) on the surface of calcified cartilage (4).
(Micrograph courtesy of R. Dintzis and from the work of D. Walker, Johns Hopkins Medical School, Baltimore, Maryland.)
Bone replaces this cartilage precursor in a series of steps that are coordinated locally by production of growth factors. Perichondrial cells and proliferating chondrocytes secrete parathyroid hormone-related protein (PTHrP), which promotes chondrocyte division and growth. In supporting roles, BMPs promote and FGFs inhibit chondrocytes growth and differentiation. More mature chondrocytes produce Indian hedgehog, which directs the terminal differentiation of neighboring chondrocytes. These hypertrophic chondrocytes cause the bone to grow longer as they grow in size, secrete type X collagen, and use matrix metalloproteinases to resorb some of their surrounding matrix. They direct the calcification of the cartilage matrix before undergoing apoptosis. Osteoblasts lay down bone matrix on the surface of the calcified cartilage. Cartilage is avascular, owing to expression of inhibitors of blood vessel growth, but hypertrophic cartilage ceases to inhibit endothelial cell growth. This allows FGF-2, TGF-b, and vascular endothelium growth factor to attract capillaries as part of the transformation of cartilage to bone.
For a long bone to maintain its shape as it grows in size, deposition and removal of bone tissue must be highly selective. For the shaft to grow in diameter, new bone is laid down on the outer surface by osteoblasts at the same time as old bone is removed inside by osteoclasts (Fig. 32-9). Bones grow longer as a result of interstitial growth of cartilage in the epiphyseal plate and its continual replacement by bone. Growth of long bones stops at puberty, when high concentrations of estrogen and testosterone stop proliferation of epiphyseal chondrocytes so that bone replaces this cartilage. This closure of the epiphyses occurs over several years in a predictable order, so one can judge the maturity of a child by examining epiphyses by radiographic studies. Genetic variations in this process of maturation give rise to differences in stature. Metabolic and endocrine disorders can also affect the timing of epiphyseal closure.
Bone Remodeling
Bone is amazingly dynamic and is remodeled continuously in response to stresses. Bone cells and matrix turn over every few years. Reorganization of bone requires two carefully coordinated steps: breakdown of preexisting bone by osteoclasts and replacement with new bone by osteoblasts. More than 100 years ago, Wolff realized that the strength of a bone depends on use. For example, bones of the racquet arm of tennis players are more robust than the bones of their other arm. Thus, mechanical forces on the bones must generate modulatory signals that control remodeling, but the molecular mechanisms are still uncertain.
Formation of the cylindrical units of long bones called osteons is a good example of well-coordinated remodeling. The process involves two steps (Fig. 32-10). First, osteoclasts resorb preexisting bone to form long, cylindrical, resorption channels in the same way that a plumber’s snake (such as the Roto-Rooter) clears debris from drain pipes. The second step is slower, as osteoblasts take weeks to fill in these channels by depositing concentric layers of lamellar bone against the walls. They lay down matrix at a rate of about 1 mm of thickness per day until bone completely surrounds the blood vessels trapped in the middle of the newly formed osteon. Because resorption channels cut randomly through the bone, fragments of older osteons are left behind during the remodeling of mature bone. These fragments are called interstitial lamellae. Resorption may release growth and differentiation factors from the mineralized matrix that provide a local stimulus for the next round of bone formation by new osteoblasts.
Bone Diseases
Osteogenesis imperfecta is the name of a variety of congenital fragile bone syndromes. Severely affected fetuses die in utero from multiple broken bones. Mildly affected individuals are born but suffer multiple fractures resulting in skeletal deformities. All of the patients have mutations in the gene for type I collagen. Some are deletions or insertions, which may be mild. Most patients with severe disease have point mutations leading to replacement of a glycine by a larger amino acid. This prevents the zipper-like folding of the collagen triple helix (see Fig. 29-1), even if only one chain is defective per molecule. This poisons assembly and accounts for the dominant phenotype. No one knows why these mutations in type I collagen do not affect other tissues, such as skin, which are rich in type I collagen.
Repair of Wounds and Fractures
Healing of minor skin wounds is a familiar occurrence that illustrates the mechanisms that control the assembly of connective tissue. Repair of connective tissue in the dermis underlying the epithelium proceeds in three stages: formation of a blood clot, assembly of provisional connective tissue, and remodeling of the connective tissue (Fig. 32-11).
Chemotactic factors attract phagocytes from the blood into the wound. These factors include PDGF, chemokines, peptides cleaved from fibrinogen by thrombin, and peptides from any contaminating bacteria. Neutrophils arrive first from the nearby blood vessels, having attached to activated endothelial cells (see Fig. 30-13) and migrated into the connective tissue and clot. They ingest any bacteria. Second, monocytes (using a similar mechanism) migrate into the clot and clear foreign material and any dead neutrophils. The environment in a wound promotes transformation of monocytes into macrophages, which synthesize and secrete cytokines and growth factors that mediate the cellular events that complete the repair process. In this way, platelets, monocytes, and fibroblasts form a relay, passing information from one cell to the next.
During the next phase of repair, macrophages, fibroblasts, and capillary endothelial cells migrate into the fibrin clot and reestablish the connective tissue. Endothelial cells form capillary loops that allow blood to flow and to provide oxygen. Initially, the endothelial cells are attracted by growth factors released by platelets, but macrophages and dissolution of fibrin provide a more sustained supply of chemoattractants and growth factors. Integrin receptors for fibronectin allow fibroblasts to migrate into the clot. They secrete more fibronectin as they move. Within the clot, PDGF and TGF-b from macrophages stimulate fibroblasts to secrete type III collagen, hyaluronan, SPARC (secreted protein acidic and rich in cysteine), and tenascin. Initially, this loose connective tissue is disorganized and weak. Hyaluronan predominates transiently, but after about five days, it is gradually replaced by proteoglycans and type I collagen.
Two events complete the repair of the matrix. First, fibroblasts differentiate into (smooth muscle–like) myofibroblasts, which contract the collagen matrix, closing the edges of the wound. This step is particularly important for large wounds. Second, fibroblasts remodel the provisional connective tissue to restore its original architecture with nearly normal physical strength. This requires resorption of provisional collagen fibrils by metalloproteinases (see Fig. 29-20) and assembly of more robust type I collagen fibrils.
While fibroblasts repair the connective tissue, the epithelium bordering the wound spreads by cell division and migration to cover the defect. This process of migration is initiated within hours of wounding. Both the loss of contacts with neighboring cells at the edge of the wound and the release of growth factors in the wound are thought to transform the static epithelial cells into migrating cells. Keratin filaments that predominate in the cytoskeleton of the skin epithelial cells are replaced with actin filaments. Hemidesmosomes that anchor the skin epithelial cells to the basal lamina are lost, and the cells migrate over the surface of the underlying matrix, which consists initially of fibrin and fibronectin and later of collagen. As they go, epithelial cells lay down a new basal lamina. Depending on the size of the defect, proliferation of epithelial cells might be required to complete coverage of the surface. When it is covered, the cells begin to differentiate into stratified epithelium.
Plant Cell Wall
The cell walls of land plants are composite materials consisting of cellulose, other polysaccharides, and glycoproteins (Fig. 32-12). Wood and cotton are two familiar examples of cell wall material that is left behind after plant cells have died. Like the extracellular matrix of animals, plant cell walls not only provide mechanical support but also may influence development. Two types of forces act on cell walls. Internally, the vacuole of the plant cell applies turgor pressure. Cell walls also resist a variety of external mechanical forces that tend to deform the cell.
The main constituent of cell walls is cellulose, the most abundant biopolymer on earth. It is a long, unbranched polymer of glucose (see Fig. 3-25). Several dozen cellulose polymers associate laterally into 5- to 7-nm bundles called microfibrils (Fig. 32-12B). Two types of branched polysaccharides—hemicellulose and pectin—associate with cellulose in microfibrils.
A complex of plasma membrane enzymes termed cellulose synthases synthesize cellulose. Arabidopsis has genes for about 30 different cellulose synthases. Genetic evidence suggests that active enzymes consist of two different synthase polypeptides. These transmembrane enzymes form a rosette of six particles that are visible by electron microscopy. Glucose polymers are initiated with a lipid anchor and then elongated by the rosettes, which extrude 36 cellulose polymers across the plasma membrane. Outside the cell, these polymers self-assemble into linear crystals called microfibrils. Hydrogen bonds constrain the glucose units to face in alternate directions in planar ribbons (see Fig. 3-25A). These ribbons self-assemble laterally into planar crystalline sheets, which stack vertically into paracrystalline bundles that are held together by C-H···O hydrogen bonds. Cellulose microfibrils in the cell wall are usually organized like barrel hoops perpendicular to the axis of cellular growth to allow for expansion. Cytoplasmic microtubules tend to have the same orientation. Cellulose synthesis moves the rosettes of cellulose synthase in the plane of the plasma membrane along paths defined by the cytoplasmic microtubules.
Glycosyltransferases in the Golgi apparatus synthesize hemicellulose and pectin, which are transported in vesicles to the surface for secretion. Hemicellulose is a branched polysaccharide that coats microfibrils. Pectin is an acidic polysaccharide that forms a gel between microfibrils. Primary cell walls, laid down at the time of cellular growth and expansion, mature with the addition of glycoproteins and organic molecules, such as lignins (polymers of phenylpropanoid alcohols and acids), which contribute to the integrity of the “secondary” cell wall. Covalent and noncovalent bonds are thought to link cellulose and these other matrix molecules. The great strength and flexibility of tree branches illustrate the remarkable mechanical properties of mature cell walls.
Cellulose microfibrils are flexible and have a tensile strength greater than that of steel, so they do not stretch. For a plant tissue to expand, microfibrils must rearrange. Slippage and rearrangement of microfibrils are facilitated by expansins, a recently recognized class of matrix proteins unique to plants. Genetic defects in expansins inhibit the growth of plant tissues and the ripening of some fruits, such as tomatoes. Cell wall expansion apparently does not involve cleavage of sugar polymers, so it is speculated that expansins break noncovalent links between the polymers transiently, allowing turgor pressure to expand the volume of the cell. Expansins in grass pollen are one allergen responsible for hay fever.
Boyle WJ, Simonent WS, Lacey DL. Osteoclast differentiation and activation. Nature. 2003;423:337-342.
Cosgrove DJ. Assembly and enlargement of the primary cell wall in plants. Annu Rev Cell Dev Biol. 1997;13:171-201.
Cosgrove DJ. Loosening of plant cell walls by expansins. Nature. 2000;407:321-326.
Goldstein AL, Hannappel E, Kleinmann HK. Thymosin b4: Actin sequestering protein moonlights to repair injured tissues. Trends Mol Med. 2005;11:421-429.
Harada S, Rodan GA. Control of osteoblast function and regulation of bone mass. Nature. 2003;423:349-355.
Kohorn BD. Plasma membrane-cell wall contacts. Plant Physiol. 2000;124:31-38.
Kronenberg HM. Developmental regulation of the growth plate. Nature. 2003;423:332-336.
Mariani FV, Martin GR. Deciphering skeletal patterning: Clues from the limb. Nature. 2003;423:319-325.
Marx SJ. Hyperparathyroid and hypoparathyroid disorders. N Engl J Med. 2000;343:1863-1875.
Olsen BR, Reginato AM, Wang W. Bone development. Annu Rev Cell Dev Biol. 2000;16:191-220.
Ortega N, Behonick DJ, Werb Z. Matrix remodeling during endochondral ossification. Trends Cell Biol. 2004;14:86-93.
Pyeritz RE. Ehlers-Danlos syndrome. N Engl J Med. 2000;342:730-732.
Raisz LG. Pathogenesis of osteoporosis: Concepts, conflicts and prospects. J Clin Invest. 2005;115:3318-3325.
Reid JG. Cementing the wall: Cell wall polysaccharide synthesizing enzymes. Curr Opin Plant Biol. 2000;3:512-516.
Somerville C. Cellulose synthesis in higher plants. Annu Rev Cell Dev Biol. 2006;22:53-78.
Teitelbaum SL. Bone resorption by osteoclasts. Science. 2000;289:1504-1508.
Tolar J, Teitelbaum SL, Orchard PJ. Osteopetrosis. 2004;351:2839-2849.
Wasteneys GO, Galway ME. Remodelling the cytoskeleton for growth and form: An overview with some new views. Ann Rev Plant Biol. 2003;54:691-722.
Watanabe H, Yamada Y, Kimata K. Roles of aggrecan, a large chondroitin sulfate proteoglycan, in cartilage structure and function. J Biochem. 1998;124:687-693.
Zlezer E, Olsen BR. The genetic basis of skeletal disease. Nature. 2003;423:343-348.