CHAPTER 14 Clinical Techniques of Cardiac Magnetic Resonance Imaging
Morphology, Perfusion, and Viability
TECHNICAL REQUIREMENTS: MOTION COMPENSATION
The ability to compensate for motion, both cardiac and respiratory in origin, is critical to successful MR imaging of the heart. As newer generations of cardiac scanners, with software and hardware advances, have become increasingly available, the motion obstacle can be better resolved. One of the most important obstacles for MRI to overcome has been the ability to image quickly enough to overcome the limited window of opportunity to produce snapshot images of a motionless heart. The implementation of electrocardiographic gating remains one of the most significant advances to facilitating imaging in this respect. This has been further optimized by the addition of k-space segmentation (Fig. 14-1).
In electrocardiographic triggering, data acquisition is synchronized to the cardiac cycle. The R wave is used as a starting point from which a defined trigger delay can be timed to acquire a portion of k-space. The trigger delay is typically set at mid-diastole, where minimal motion occurs.1 This partitioning allows each package of data to be acquired in a short time interval, ideally less than 50 ms, and therefore without motion degradation. In k-space segmentation, the full complement of k-space data is compiled by imaging over several successive cardiac cycles, each of which contributes its respective portion of information. Electrocardiographic triggering can be implemented in a prospective fashion, where the R wave is an actual trigger for data acquisition. More often, however, retrospective triggering is used, in which the R wave is a marker for sorting information already acquired through the entire R-R interval. The latter allows complete diastolic phase imaging, which is important in accurate functional assessment for which end-systolic and end-diastolic views of the cine sequence are required.
Electrocardiographic gating may be impaired in patients with large thoracic cavities, such as in emphysema, or when large pericardial effusions decrease the electrical signal. Another artifact, the magnetohydrodynamic effect, occurs when ions contained within blood pass through the magnetic field, inducing a voltage that distorts the electrocardiographic tracing. This particular limitation can be minimized with an optimized version of gating available through vector electrocardiography, which is less prone to distortion of the cardiac tracing by the magnetohydrodynamic effect.2
The second significant motion hurdle to overcome has been the elimination of respiratory artifacts. This has been addressed in abdominal imaging by using breath-holding during image acquisition, when possible, and has been adapted to MRI as well. Although this produces excellent image quality with fast imaging techniques such as turbo field echo and echo-planar imaging, there remain limitations. Breath-hold reproducibility can be low, resulting in potential slice misregistration in multislice acquisitions. This is particularly problematic in left ventricular function assessment on short-axis cine imaging. Instructing patients to breath-hold at end-expiration has been shown to provide the most consistent data and to minimize variability in position.3 Breath-hold imaging is also limited by the amount of time that can be spent on other aspects of the image quality, such as the signal-to-noise ratio (SNR) and spatial and temporal resolution. This limitation is in part offset by parallel imaging techniques that can speed up imaging and provide magnetic resonance (MR) currency of time, which can be used for optimization of other imaging parameters.
More recently, an alternate method for respiratory compensation has been developed in the form of the navigator echo technique (Fig. 14-2). First described by Ehman and Felmlee,4 this technique uses an interleaved column of excitation perpendicular to the direction of motion for assessment of tissue displacement. The navigator echo is typically prescribed at interfaces with high tissue contrast; such as the right hemidiaphragm, where signal is compared to a reference echo for displacement. Information on displacement can then be used to select echoes for image reconstruction that reflect nonmotion. The scan efficiency depends on operator-dependent factors such as stringency of gating criteria, with windows of 3 to 5 mm being typical for acceptance or rejection of echo information. The larger the acceptance window, the higher the efficiency and shorter overall acquisition time, but with consequent increase in motion blurring. Other factors that can be manipulated in the navigator technique include prospective versus retrospective acquisition and the number of navigator echoes acquired, with a resulting balance between image quality and time efficiency. By avoiding breath-holding in this technique, patient comfort and compliance are improved. One of the primary advantages of the navigator technique is the ability to spend time currency to improve SNR and spatial resolution.
Limitations to navigator techniques include respiratory drift of the diaphragm beyond the acceptance window, which occurs in patients who fall asleep or are anxious during the early part of an examination but later settle into a different rhythm.5 Careful monitoring of patients’ breathing patterns and keeping them alert during the scan acquisition can minimize respiratory drift. Further improvements have been made to the navigator technique specifically for coronary MR angiography (MRA) by phase ordering.
TECHNIQUES
Anatomic Overview
Indications
Cardiac examinations generally begin with a morphologic overview of the heart, pericardium, mediastinum, and great vessels. T1-weighted images provide an excellent overview of this anatomy and thus remain standard in most MRI protocols. Tissue characterization can also be performed on T1-weighted images, looking for fat in entities such as arrhythmogenic right ventricular dysplasia or cardiac mass lesions. T2-weighted images, which can further characterize focal masses, are now demonstrating increased usefulness for several clinical diseases, often reflecting edema in the myocardium. This can be especially helpful in ischemic heart disease, in which differentiation of acute from chronic infarcts, which can look similar on viability sequences (see later), can be made by the presence of a bright T2 signal in the acute scar.6 Similarly, T2 edema can be identified in areas of disease involvement in acute myocarditis7 and nonischemic cardiomyopathy.8
MRI has also been demonstrated to be useful in the noninvasive assessment of cardiac iron overload. Patients with thalassemia, for example, may have transfusion-related iron overload. The presence of iron has prognostic implications for these patients with an increased risk of heart failure. Iron deposition in the heart is also an important cause for mortality in this patient population compared with other organs. Early detection can be difficult by serum ferritin measurements, which are not necessarily reflective of myocardial content, and routine biopsy of the myocardium is not feasible. T2*-weighted imaging can provide noninvasive measures of iron deposition in the myocardium. with correlation to deterioration in ventricular function.9
Technique Description
Turbo spin-echo (TSE) sequences are most commonly used for routine morphologic assessment. In contrast to other anatomic locations, the TSE sequence in cardiac imaging is modified by the implementation of a dual inversion preparatory pulse that produces black blood (BB) images. The absence of signal in the blood pool is achieved by using a selective and nonselective 180-degree inversion pulse, which is followed by a long inversion time chosen to null blood magnetization (Fig. 14-3). These preparatory pulses are followed by a gated TSE readout with k-space segmentation. This can be performed with either breath-holding or free-breathing.
The black blood pulse produces good myocardium–blood pool differentiation. These static images are of high SNR, with good tissue contrast (Fig. 14-4). Furthermore, image parameters are easily manipulated to allow for T1- or T2-weighted tissue characterization. If fat saturation is required, an additional selective 180-degree inversion pulse can be added with a short inversion time to null fat, also known as STIR (short tau inversion recovery).
Pitfalls and Solutions
Slow-flowing blood can result in poor blood suppression, producing an endocardial border of hyperintensity on T2-weighted images. When a thin layer of pseudo-T2 involvement is isolated to the endocardium, correlation should be made to other imaging sequences to help determine the true anatomic location of signal changes. If the changes are determined to be adjacent to the endocardium, then they are likely artefactual. This artefact is particularly notable in regions adjacent to wall motion abnormality, at the ventricular apex, or the interstices of trabeculae.11
Perfusion
Indications
Myocardial perfusion is important in determining the hemodynamic significance of coronary artery stenoses identified at angiography. Nuclear cardiology currently fulfills the role of perfusion assessment by single photon emission computed tomography (SPECT)12 and positron emission tomography (PET) imaging.13 However, both techniques share the relative limitation of spatial resolution and radiation exposure. SPECT imaging also suffers from soft tissue attenuation, whereas access to PET imaging, despite its usefulness, is limited at this time.
Cardiac MR strengths include good tissue contrast, spatial resolution, and temporal resolution, which make it a natural candidate for perfusion imaging. First-pass perfusion MRI with gadolinium-based contrast agents is an accepted tool in the evaluation of coronary artery disease (Fig. 14-5).14 When perfusion MRI is compared with current scintigraphic techniques, it performs well.15 Overall, numerous studies evaluating the performance of MR perfusion in detecting obstructive coronary artery disease produce sensitivity and specificity of 83% and 82%, respectively.16
Contraindications
Perfusion imaging is not performed if the patient cannot receive the stress agent. Table 14-1 lists contraindications to pharmacologic stress agents. General contraindications to MR examination and to gadolinium-based contrast agents, including nephrogenic systemic fibrosis are also reasons that preclude cardiac MR perfusion examinations.
Agents | Contraindications |
---|---|
Dobutamine |
Technique Description
Currently a T1-GRE, hybrid gradient-echo–echoplanar imaging (GRE-EPI), or balanced steady-state free precession (SSFP) sequence can be used for perfusion imaging. The T1-GRE sequence is limited by lower spatial and/or temporal resolution so the other two techniques are now favored. Both the hybrid GRE-EPI and balanced SSFP techniques have been validated recently.14 T1 weighting using a saturation recovery preparatory pulse has replaced inversion recovery as the current method of contrast preparation. Generally, short-axis views with at least three slice locations should be acquired—ideally each heartbeat, but every other one is acceptable. The in-plane resolution should be less than 3 mm with a temporal resolution of approximately 150 ms or less. Imaging duration should be long enough for the contrast to pass through the LV myocardium. As in many other areas, parallel imaging may be used to shorten the acquisition time. This is particularly useful in perfusion imaging to reduce motion artifacts and increase spatial or temporal resolution but it is limited by the tradeoff in SNR.
Contrast media used in perfusion imaging are typically gadolinium-based extracellular agents. The dosing range is between 0.025 to 0.15 mmol/kg body weight. MR-IMPACT, a multicenter, multivendor trial assessing the diagnostic performance of MRI perfusion with coronary angiography and SPECT, evaluated different contrast agent dosing regimens. Patients were randomized to receive 0.01, 0.025, 0.05, 0.075, or 0.10 mmol/kg per stress and rest injection, with the 0.10-mmol/kg dose resulting in the best performance of MRI perfusion.17 Nonetheless, variability still exists between imaging protocols and dosing regimens at different institutions, reflecting the lack of uniform agreement on the optimal imaging protocol. In routine clinical practice with qualitative visual analysis, the upper limit of dosing is preferable. In distinction, when performing semiquantitative analysis, a lower concentration dose regimen (e.g., 0.025 mmol/kg) is optimal for ensuring the relative linear relationship between gadolinium concentration and myocardial signal intensity.
Reporting
Qualitative interpretation remains the primary method of clinical reporting of perfusion MR imaging at this time. Full examination review, which requires interpretation of the delayed hyperenhancement (DHE) images in conjunction with stress perfusion images, is ideal in clinical practice.18
Delayed Hyperenhancement
Indications
LV function is an important factor in the long-term survival of patients with ischemic heart disease (IHD) for whom severe LV dysfunction is associated with a poor prognosis. However, LV function can improve after revascularization procedures, including both percutaneous transluminal coronary angioplasty (PTCA) and stent placement or coronary artery bypass grafting (CABG).19 Thus, the determination of tissue viability becomes paramount.
The concept of infarct-related hyperenhancement in MRI has long existed but has rapidly gained acceptance as a standard investigative tool with newer imaging techniques and their ability to detect and size infarcted tissue in vivo.20 The mechanism of delayed hyperenhancement in acute infarcts, although still incompletely understood, is thought to occur because of the increased volume of distribution of contrast agent resulting from myocardial interstitial edema and myocyte membrane disruption (Fig. 14-6).21 Similarly, in chronic infarcts, delayed hyperenhancement can be seen because the dense collagen of scar tissue may contain greater interstitial space than that between myocytes of viable myocardium, which also results in greater accumulation of contrast agent.
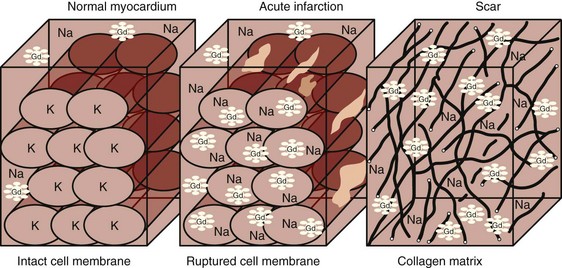
FIGURE 14-6 Potential mechanisms of delayed hyperenhancement in acute and chronic infarctions. See text for details.
(From Weinsaft J, Klem I, Judd RM. MRI for the assessment of myocardial viability. In Kim RJ [ed]. Cardiovascular MR Imaging. Philadelphia, WB Saunders, 2007, p 509.)
MRI thus represents a new tool to assess viability. Advantages to MRI include the lack of ionizing radiation and provision of simultaneous functional assessment. One particular advantage of DHE-MRI is the improvement in spatial resolution compared with existing techniques. The superior resolution of DHE-MRI provides a distinct advantage for imaging nontransmural infarction.22 Numerous studies have since compared DHE-MRI with current imaging modalities and confirmed this advantage. When Klein and colleagues23 compared PET and DHE-MRI in study patients, both performed well in the assessment of nonviable tissue but more infarcts were detected by DHE-MRI. Furthermore, when evaluating subendocardial infarcts (nontransmural), approximately one half (55%) were identified by DHE-MRI but classified as normal by PET. Similar differences have been shown in comparisons of DHE-MRI with SPECT imaging, where subendocardial infarcts (<50% wall thickness by histopathology) were identified in 92% (MRI) and 28% (SPECT) of patients, respectively.24
The superior spatial resolution of DHE-MRI is important to consider in conjunction with the ability to identify the viable component of myocardium adjacent to infarct (Fig. 14-7). When considering the transmural extent of infarction, DHE-MRI is ideally suited for quantifying the viable nonenhancing myocardium versus nonviable delayed enhancing scar. The percentage of wall thickness involvement is a direct measurement compared with the entire wall thickness at that level. In contrast, other techniques, such as dobutamine stress echocardiography, infer viability by response to exogenous drug administration. Nuclear techniques visualize viable tissue but do not directly see the nonviable tissue; they compare it with a remote normal zone. This degree of visualization may have implications for management and prognostic stratification.
In large areas of acute infarctions, one may observe microvascular obstruction (MO) with capillary occlusion and accumulation of debris. This phenomenon is thought to occur from damage and obstruction at the microcirculatory level, with reduced perfusion resulting in limited penetration of gadolinium contrast agent to the infarct core.25 The presence of MO can be identified on DHE-MRI as an area of no-reflow and has important implications for patients because they are at increased risk of recurrent chest pain, heart failure, and infarction (Fig. 14-8).26 The presence of MO was better demonstrated by DHE-MRI compared with contrast echo in one study, also confirming the importance of this finding as a prognostic factor in immediate postinfarct complications.27 Thus, there is extensive literature supporting the usefulness of DHE-MRI as a first-line test for imaging viability.
However, not all DHE is related to infarcted scar tissue. DHE-MRI imaging can be used to assess nonischemic origin cardiac disease as well. Patients with acute myocarditis can present with acute coronary syndrome but if a comprehensive cardiac work-up is performed, they show no evidence of coronary artery disease (CAD). The differential diagnosis of acute coronary syndrome includes acute myocarditis, which is often challenging to assess clinically and is a diagnosis of exclusion. In these patients, MRI can be helpful in the initial diagnosis and follow-up of the disease, with DHE-MRI reflecting inflammation in the acute phase. The presence of DHE may be the most common finding at diagnosis and can have a unique nonischemic distribution. Furthermore, the DHE on follow-up MRI may have prognostic implications with respect to long-term outcome in patients with myocarditis.28
DHE can also be seen in hypertrophic cardiomyopathy (CMO), with distinct nonischemic patterns involving the midwall, subepicardium, or right ventricular free wall. The presence and degree of enhancement may be related to risk factors for sudden death in this population.29
Similarly, cardiac involvement in sarcoidosis can be demonstrated by DHE-MRI with a predilection for the subepicardium of the anteroseptal and inferolateral walls (Fig. 14-9). In one small study, the degree of enhancement was inversely related to treatment with steroids, which suggests that DHE could be used as a surrogate marker for response to therapy.30 In a study of 81 patients by Patel and associates,31 a relatively high prevalence of delayed hyperenhancement was demonstrated, which was twice as sensitive for the detection of cardiac involvement compared with the current consensus criteria. More importantly, this study showed DHE-MRI to be the only independent predictor of adverse clinical events including cardiac death.
Cardiac involvement in amyloid remains a difficult diagnosis to make, even with endomyocardial biopsy, and carries a poor prognosis, with a decrease in median survival. Not only has DHE-MRI been shown to be able to image patients with characteristic subendocardial enhancement positively,32 but it also demonstrates prognostic value with decreased median survival and increased rate of death or heart transplantation.33
Finally, DHE-MRI has also been shown to be helpful in evaluating certain complications of IHD. False aneurysms may develop postinfarction when there is disruption of the complete wall thickness but integrity is maintained by overlying pericardium. Differentiating true from false aneurysms in the heart is crucial because the latter are at greater risk for rupture and surgical treatment is considered first-line therapy. Although classic imaging features exist for differentiating the two types of aneurysms, DHE may play an additional role in distinguishing these entities. In a small study of 22 patients, Konen and coworkers34 have demonstrated a pericardial DHE pattern, with false aneurysms being more commonly identified than true aneurysms.
Patients with intracardiac thrombi are at risk of distal embolization and are currently imaged by echocardiography. Correct identification is important for the initiation of medical therapy to prevent cerebrovascular events. Echocardiography, however, has mixed results in identifying thrombi and is subject to interobserver variability. In one study by Srichai and colleagues,35 MRI was more sensitive to the detection of thrombi (88%) compared with transthoracic (23%) and transesophageal echocardiography (40%). Typically, MRI imaging with cine sequences is used to identify thrombus but the routine addition of DHE may have incremental value, as suggested in a recent study by Weinsaft and associates.36 In their study of 784 patients with systolic dysfunction, DHE-MRI demonstrated a significant increase in the number of thrombi detected (55 patients) compared with cine MRI (37 patients) alone (Fig. 14-10).
Contraindications
No specific contraindications exist aside from those related to contrast administration.
Technique Description
Although many techniques have been described for imaging delayed hyperenhancement, currently a segmented k-space turbo field echo sequence with a trigger delay for diastole to minimize cardiac motion and an inversion pulse set to null normal myocardium is most commonly used (Fig. 14-11).37 The inversion pulse maximizes the contrast between abnormal myocardium with gadolinium accumulation (delayed hyperenhancement) and normal myocardium, which will appear dark.
Inversion time (TI) is determined by running a trial of pre-DHE images at varying TI times and selecting the most appropriate time for nulling normal myocardium (Fig. 14-12). Vendor-specialized software is available, such as Look-Locker (Philips Medical Systems, Best, The Netherlands) or TI Surf (Siemens Medical Solutions, Erlangen, Germany), which simplifies this process by acquiring a range of TI sample images in a single breath-hold for comparison.
Newer techniques for DHE may obviate the need for a Look-Locker and the time required to determine the optimal inversion time. Whereas classic inversion recovery–turbo field echo (IR-TFE) sequences are magnitude based, with image quality and infarct depiction significantly dependent on IR time selection, the phase-sensitive inversion recovery sequence produces a spectrum of DHE-MRI images that maintain DHE to normal myocardium differentiation across a spectrum of IR times.38
Despite the success of the IR-TFE sequence for delayed hyperenhancement, there are limitations of two-dimensional imaging. Slice gap misregistration and time-consuming breath-holds may lead to loss of patient cooperation, fatigue, or inadequate image quality. To overcome some of these issues, three-dimensional techniques are now being used with potential benefits in contrast-to-noise ratio, SNR, and time savings.39
However, if patients are still unable to breath-hold, then navigator-facilitated methods can produce diagnostic images, which may be shorter in overall acquisition time. The patients can breathe freely and comfortably while the scanner acquires the imaging volume so that patients are not subjected to repetitive exhaustive breath-holding. However, many patients undergoing investigation for cardiac DHE are poor breath-holders and have irregular cardiac rhythms, which may render all motion compensation techniques useless. In this subset of patients, newer subsecond DHE sequences are superior to the segmented IR technique.40
Pitfalls and Solutions
Appropriate selection of inversion time is crucial to the image quality and subsequent scar visualization on DHE-MRI. The optimal time relies on a subjective assessment of optimal suppression; the MR technologist or radiologist must decide, based on pre-DHE images, which portion of myocardium is normal to determine the IR time that will produce suppressed (dark) viable myocardium. Optimal suppression leads to maximal differentiation of delayed enhancing infarct from the viable myocardium. Improper IR time selection will lead to poor differentiation of scar tissue from normal myocardium or accidental reversal of signal intensities, with a dark infarct and bright normal myocardium (Fig. 14-13). Careful assessment of Look-Locker or TI Surf scouts and correlation to cine imaging can help identify normal myocardium and assist in optimizing the differentiation of scar from normal myocardium.
Infarct sizing is important in assessing response to therapy, particularly in research trials. Improper timing of DHE-MRI outside of the 15- to 20-minute window can lead to improper assessment of scar tissue extent (up to 28% discrepancy). Imaging earlier may overestimate enhancement, whereas late imaging may result in underestimation.41 The simple recognition of infarct evolution over time on DHE-MRI is sufficient to maintain constant imaging within the optimal imaging range of 15 minutes.
Reporting
When considering ischemic heart disease, DHE-MRI images are usually interpreted in conjunction with cine imaging in three main combinations (Fig. 14-14).
Abdel-Aty H, Simonetti O, Friedrich MG. T2-weighted cardiovascular magnetic resonance imaging. J Magn Reson Imaging. 2007;26:452-459.
Kramer CM, Barkhausen J, Flamm SD, et al. Standardized cardiovascular magnetic resonance imaging (CMR) protocols. Society for Cardiovascular Magnetic Resonance: board of trustees task force on standardized protocols. J Cardiovasc Magn Reson. 2008;10:35.
Sakuma H. Magnetic resonance imaging for ischemic heart disease. J Magn Reson Imaging. 2007;26:3-13.
Vogel-Claussen J, Rochitte CE, Wu KC, et al. Delayed enhancement MR imaging: utility in myocardial assessment. RadioGraphics. 2006;26:795-810.
1 Hofman MBM, Wickline SA, Lorenz CH. Quantification of in-plane motion of the coronary arteries during the cardiac cycle: implications for acquisition window duration for MR flow quantification. J Magn Reson Imaging. 1998;8:568-576.
2 Fischer SE, Wickline SA, Lorenz CH. Novel real-time R-wave detection algorithm based on the vectorcardiogram for accurate gated magnetic resonance acquisitions. Magn Reson Med. 1999;42:361-370.
3 Palthow C, Ley S, Zaporozhan J, et al. Assessment of reproducibility and stability of different breath-hold manoeuvres by dynamic MRI: comparison between healthy adults and patients with pulmonary hypertension. Eur Radiol. 2006;16:173-179.
4 Ehman R, Felmlee JP. Adaptive technique for high-definition MR imaging of moving structures. Radiology. 1989;173:255-263.
5 Taylor AM, Jhooti P, Weismann F, et al. MR navigator-echo monitoring of temporal changes in diaphragm position: implications for MR coronary angiography. J Magn Reson Imaging. 1997;7:629-636.
6 Abdel-Aty H, Zagrosek A, Schulz-Menger J, et al. Delayed enhancement and T2-weighted cardiovascular magnetic resonance imaging differentiate acute from chronic myocardial infarction. Circulation. 2004;109:2411-2416.
7 Abdel-Aty H, Boye P, Zagrosek A, et al. Diagnostic performance of cardiovascular magnetic resonance imaging in patients with suspected acute myocarditis: comparison of different approaches. J Am Coll Cardiol. 2005;45:1815-1822.
8 Sharkey SW, Lesser JR, Zenovich AG, et al. Acute and reversible cardiomyopathy provoked by stress in women from the United Sates. Circulation. 2005;111:472-479.
9 Anderson LJ, Holden S, Davis B, et al. Cardiovascular T2-star (T2*) magnetic resonance for the early diagnosis of myocardial iron overload. Eur Heart J. 2001;22:2171-2179.
10 Reference deleted in proofs.
11 Abdel-Aty H, Simonetti O, Friedrich M. T2-weighted cardiovascular magnetic resonance imaging. J Magn Reson Imaging. 2007;26:452-459.
12 Maddahi J, Van Train K, Prigent F, et al. Quantitative single photon emission computed thallium-201 tomography for detection and localization of coronary artery disease: optimization and prospective validation of a new technique. J Am Coll Cardiol. 1989;14:1689-1699.
13 Muzik O, Duvernoy C, Beanlands RS, et al. Assessment of diagnostic performance of quantitative flow measurements in normal subjects and patients with angiographically documented coronary artery disease by means of nitrogen-13 ammonia and positron emission tomography. J Am Coll Cardiol. 1998;31:534-540.
14 Giang TH, Nanz D, Coulden R, et al. Detection of coronary artery disease by magnetic resonance myocardial perfusion imaging with various contrast medium doses: first European multi-centre experience. Eur Heart J. 2004;25:1657-1665.
15 Schwitter J, Nanz D, Kneifel S, et al. Assessment of myocardial perfusion in coronary artery disease by magnetic resonance: a comparison with positron emission tomography and coronary angiography. Circulation. 2001;103:2230-2235.
16 Kim HWJ, Klem I, Kim RJ. Detection of myocardial ischemia by stress perfusion cardiovascular magnetic resonance. In: Kim RJ, editor. Cardiovascular MR Imaging. Philadelphia: WB Saunders; 2007:527-540.
17 Schwitter J, Wacker CM, van Rossum AC, et al. MR-IMPACT: comparison of perfusion-cardiac magnetic resonance with single-photon emission computed tomography for the detection of coronary artery disease in a multicentre, multivendor, randomized trial. Eur Heart J. 2008;29:480-489.
18 Klem I, Heitner JF, Shah DJ, et al. Improved detection of coronary artery disease by stress perfusion cardiovascular magnetic resonance with the use of delayed enhancement infarction imaging. J Am Coll Cardiol. 2006;47:1630-1638.
19 Elefteriades JA, Tolis G, Levi E, et al. Coronary artery bypass grafting in severe left ventricular dysfunction: excellent survival with improved ejection fraction and functional state. J Am Coll Cardiol. 1993;22:1411-1417.
20 Kim RJ, Fieno JS, Parrish TB, et al. Relationship of MRI delayed contrast enhancement to irreversible injury, infarct age, and contractile function. Circulation. 1999;100:1992-2002.
21 Saeed M, Wendland MF, Masui T, Higgins CB. Reperfused myocardial infarctions on T1- and susceptibility-enhanced MRI: evidence for loss of compartmentalization of contrast media. Magn Reson Med. 1994;31:31-39.
22 Kim RJ, Wu E, Rafael A, et al. The use of contrast-enhanced magnetic resonance imaging to identify reversible myocardial dysfunction. N Engl J Med. 2000;343:1445-1453.
23 Klein C, Nekolla SG, Bengel FM, et al. Assessment of myocardial viability with contrast-enhanced magnetic resonance imaging: comparison with positron emission tomography. Circulation. 2002;105:162-167.
24 Wagner A, Mahrholdt H, Holly TA, et al. Contrast-enhanced MRI and routine single photon emission computed tomography (SPECT) perfusion imaging for detection of the subendocardial myocardial infarcts: an imaging study. Lancet. 2003;361:374-379.
25 Rochitte CE, Lima JA, Bluemke DA, et al. Magnitude and time course of microvascular obstruction and tissue injury after acute myocardial infarction. Circulation. 1998;98:1006-1014.
26 Wu KC, Zerhouni EA, Judd RM, et al. Prognostic significance of microvascular obstruction by magnetic resonance imaging in patients with acute myocardial infarction. Circulation. 1998;97:765-772.
27 Wu KC, Kim RJ, Bluemke DA, et al. Quantification and time course of microvascular obstruction by contrast-enhanced echocardiography and magnetic resonance imaging following acute myocardial infarction and reperfusion. J Am Coll Cardiol. 1998;32:1756-1764.
28 Wagner A, Schultz-Menger J, Deitz R, Friedrich MG. Long-term follow-up of patients with acute myocarditis by magnetic resonance imaging. MAGMA. 2003;16:17-20.
29 Moon JCC, McKenna WJ, McCrohon JA, et al. Toward clinical risk assessment in hypertrophic cardiomyopathy with gadolinium cardiovascular magnetic resonance imaging. J Am Coll Cardiol. 2003;41:1561-1567.
30 Vignaux O, Dhote D, Duboc D, et al. Cardiac magnetic resonance imaging findings in sarcoidosis. J Cardiovasc Magn Reson. 2003;5:15-16.
31 Patel M, Cawley P, Heitner JE, et al. Detection of myocardial damage in patients with sarcoidosis. Circulation. 2009;120:1969-1977.
32 Kwong RY, Falk RH. Cardiovascular magnetic resonance in cardiac amyloidosis. Circulation. 2005;111:122-124.
33 White J, Patel M, Shah DJ, et al. Prognostic utility of delayed enhancement cardiac magnetic resonance imaging in patients with systemic amyloidosis and suspected cardiac involvement. Circulation. 2006;114:679.
34 Konen E, Merchant N, Gutierrez C, et al. True versus false aneurysm: differentiation with MR imaging—initial experience. Radiol. 2005;236:65-70.
35 Srichai MB, Junor C, Rodriguez LL, et al. Clinical, imaging, and pathologic characteristics of left ventricular thrombus: a comparison of contrast enhanced magnetic resonance imaging, transthoracic echocardiography and transesophageal echocardiography with surgical or pathologic validation. Am Heart J. 2006;152:75-84.
36 Weinsaft JW, Kim HW, Shah DJ, et al. Detection of left ventricular thrombus by delayed enhancement cardiovascular magnetic resonance. J Am Coll Cardiol. 2008;52:148-157.
37 Simonetti OP, Kim RJ, Fieno DS, et al. An improved MR imaging technique for the visualization of myocardial infarction. Radiology. 2001;218:215-223.
38 Huber AM, Schoenberg S, Hayes C, et al. Phase-sensitive inversion-recovery MR imaging in the detection of myocardial infarction. Radiology. 2005;237:854-860.
39 Tatli S, Zou K, Fruitman M, et al. Three-dimensional magnetic resonance imaging for myocardial-delayed hyperenhancement: a comparison with the two- dimensional technique. J Magn Reson Imaging. 2004;20:378-382.
40 Burkhard S, Rehwald WG, Albert TSE, et al. Respiratory motion and cardiac arrhythmia effects on diagnostics accuracy of myocardial delayed-enhancement MR imaging in canines. Radiology. 2008;247:106-114.
41 Oshinski JN, Yang Z, Jones JR, et al. Imaging time after Gd-DTPA injection is critical in using delayed enhancement to determine infarct size accurately with magnetic resonance imaging. Circulation. 2001;104:2838-2842.