Chapter 32B Clinical Neurophysiology
Clinical Electromyography
Clinical electromyography is a distinct medical discipline that plays a pivotal role in the diagnosis of neuromuscular disorders (Katirji et al., 2002). The designations clinical electromyography, electrodiagnostic examination, and electroneuromyography are used interchangeably to encompass the electrophysiological study of nerve and muscle; the terms needle electromyography or needle electrode examination are reserved for the specific testing that involves needle electrode evaluation of muscle. Although many still refer to all such testing as simply electromyography (EMG), use of the word without a descriptor is discouraged because it can be confusing, often implying only the needle electrode part of the evaluation. For clarity, this chapter will refer to clinical EMG or needle EMG in the context of the discussion.
The clinical EMG examination is an important diagnostic tool that helps localize a neuromuscular problem at the motor neurons, nerve roots, peripheral nerves, neuromuscular junction, or muscle. It also helps establish the underlying process in these disorders and assess their management and prognosis. Electrodiagnostic testing provides the most valuable diagnostic information when the clinical assessment suggests a short list of differential diagnoses. The clinician should perform a detailed or focused neurological examination before referring the patient for a clinical EMG, which in turn serves as an independent procedure to provide an objective assessment of the peripheral nervous system (PNS) (Katirji, 2002). Patients with complex clinical pictures are best served by a neurological consultation prior to ordering electrodiagnostic testing.
The clinical EMG examination is composed of two main tests: nerve conduction studies (NCS) and needle EMG. These tests complement each other, and both often are necessary for a definite diagnosis. Additional electrodiagnostic procedures include assessment of F waves, H reflexes, and blink reflexes; repetitive nerve stimulation; and single-fiber EMG. A focused history and examination will help the electromyographer design the most appropriate electrodiagnostic study (Preston and Shapiro, 2005). The electromyographer must be proficient in using modern electrodiagnostic equipment and applying electrodiagnostic techniques, know the normal values for commonly and uncommonly examined nerve conduction studies and for motor unit action potentials (MUAPs) in different muscles, and be familiar with the specific and nonspecific electrodiagnostic findings in different neuromuscular disorders.
Nerve Conduction Studies
Motor Nerve Conduction Studies
The nerve usually is stimulated, whenever technically feasible, at two or more points along its course. Shorter nerves such as the axillary, femoral, and facial nerves are stimulated at only one point, because the more proximal portions of the nerves are inaccessible. Otherwise, the nerve typically is stimulated distally near the recording electrode and more proximally to evaluate one or more proximal segments. Motor NCS evaluate several measurements (Fig. 32B.1):
CMAP amplitude: The usual measure of amplitude is from baseline to negative peak and expressed in millivolts. When recorded with surface electrodes, CMAP amplitude is a semiquantitative measure of the number of axons conducting between the stimulating and recording points. CMAP amplitude also depends on the relative conduction speed of the axons, the integrity of the neuromuscular junctions, and the number of muscle fibers that are able to generate action potentials.
CMAP duration: This measurement usually is the duration of the negative phase of the evoked potential and expressed in milliseconds. It is a function of the conduction rates of the various axons forming the examined nerve and the distance between the stimulation and recording electrodes. The CMAP generated from proximal stimulation is slightly longer in duration and of lower amplitude than that obtained from distal stimulation, as a result of temporal dispersion and phase cancellation (see forthcoming section).
CMAP area: This usually is limited to the negative phase area under the waveform and shows linear correlation with the product of amplitude and duration. Measurement is in millivolts per millisecond and requires electronic integration using computerized equipment. The ability to measure CMAP area has practically replaced the need to measure its duration.
Latencies: Latency is the time interval between nerve stimulation (shock artifact) and the CMAP onset. Expression of latency is in milliseconds and reflects the conduction rate of the fastest-conducting axon. Whenever technically possible, the nerve is stimulated at two points: a distal point near the recording site and a more proximal point; the measures obtained are the distal latency and proximal latency, respectively. Both latencies depend mostly on the length of the nerve segment and, to a much lesser extent, on neuromuscular transmission time and propagation time along the muscle membrane.
Conduction velocity: This is a computed measurement of the speed of conduction expressed in meters per second. Measurement of conduction velocity allows comparison of the speed of conduction of the fastest fibers between different nerves and subjects, irrespective of the length of the nerve. The calculation requires measurement of the length of the nerve segment between distal and proximal stimulation sites. Measuring the surface distance along the course of the nerve estimates the nerve length; it should be more than 10 cm to improve the accuracy of surface measurement.
As with latencies, motor conduction velocity measures the speed of conduction of the fastest axon. In contrast with motor latency, however, motor nerve conduction velocity is a pure nerve conduction time, because neuromuscular transmission time and muscle fiber propagation time are common to both stimulation sites, and the latency difference between two points is the time required for the nerve impulse to travel from one stimulus point to the other.
Sensory Nerve Conduction Studies
SNAPs may be obtained by several methods: (1) stimulating and recording a pure sensory nerve (such as the sural and radial sensory nerves); (2) stimulating a mixed nerve while recording distally over a cutaneous branch (such as the antidromic median and ulnar sensory responses), or (3) stimulating a distal cutaneous branch while recording over a proximal mixed nerve (such as in orthodromic median and ulnar sensory studies). Similar to their motor counterparts, sensory NCS record several measurements (Fig. 32B.2):
SNAP amplitude: This semiquantitatively measures the number of sensory axons that conduct between the stimulation and recording sites. The calculation is from the baseline to negative peak or from negative peak to positive peak, and expressed in microvolts. SNAP duration and area may be measured but are not useful because of significant temporal dispersion and phase cancellation (see later discussion).
Latencies: Sensory distal latencies are measured (in milliseconds) from the stimulus artifact to the peak of the negative phase (peak latency) or from the stimulus artifact to the onset of the SNAP (onset latency). A large shock artifact, a noisy background, or a wavy baseline may obscure onset latency. Although peak latency does not reflect the fastest-conducting sensory fibers, it is easily defined and more precise than onset latency.
Conduction velocity: This requires stimulation at a single site only because the latency consists of just the nerve conduction time from the stimulus point to the recording electrode. As with motor velocity, the calculation may be done using both distal and proximal stimulations. Only onset latencies (not peak latencies) are useful to calculate velocities to assess the speed of the fastest-conducting fibers.
Segmental Stimulation in Short Increments
The inching (or actually “centimetering”) technique is particularly useful in assessing nerve conduction in patients with carpal tunnel syndrome or an ulnar neuropathy at the elbow or wrist (McIntosh et al., 1998). For example, stimulation of a normal median nerve in 1-cm increments across the wrist results in latency changes of approximately 0.16 to 0.21 msec/cm from midpalm to distal forearm (Fig. 32B.3). A sharply localized latency increase across a 1-cm segment indicates a focal abnormality of the median nerve (Fig. 32B.4). An abrupt change in waveform usually accompanies the latency increase across the site of compression.
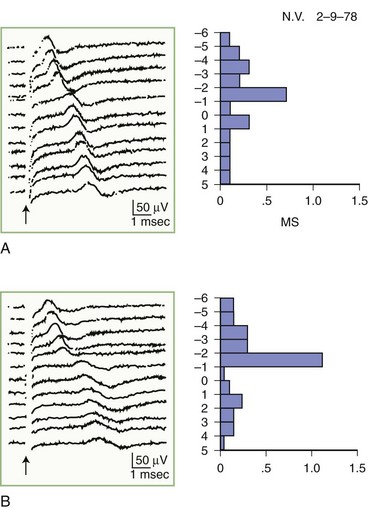
Fig. 32B.4 Sensory nerve action potentials in a patient with bilateral carpal tunnel syndrome (see also Fig. 35B.3 for settings). A sharply localized slowing was found from point −2 to point −1 in both hands, with a latency change measuring 0.7 msec on the left (A) and 1.1 msec on the right (B), compared with the other segments with normal latency changes of approximately 0.16 to 0.21 msec. Note also a distinct change in waveform of the sensory potential at the point of localized conduction delay.
(Reprinted with permission of the author and publisher from Kimura, J., 1979. The carpal tunnel syndrome: localization of conduction abnormalities within the distal segment of the median nerve. Brain 102, 619-635. By permission of Oxford University Press, Inc.)
Physiological Variability and Common Sources of Error
The major pitfalls in NCS usually involve technical errors in the stimulating or recording system (Kimura, 1997). Common errors include large stimulus artifact, increased electrode noise, submaximal stimulation, co-stimulation of adjacent nerve not under study, eliciting an unwanted potential from distant muscles, recording or reference electrode misplacement, and errors in measurement of nerve length and conduction time. Other errors are attributable to intertrial and physiological variability, which include the effects of temperature, age, the length of studied nerve, anomalous innervation, and temporal dispersion.
Temperature
Nerve impulse propagation slows by 2.4 m/sec, or approximately 5%, per degree centigrade from 38°C to 29°C of body temperature. Also, cooling results in a higher CMAP and SNAP amplitude and longer response duration, probably because of accelerated and slowed sodium channel inactivation (Rutkove et al., 1997). Therefore, a CMAP or SNAP with high amplitude and slow distal latency or conduction velocity should raise the suspicion of a cool limb. To reduce this type of variability, a plate thermistor measures skin temperature. This measurement correlates linearly with the subcutaneous and intramuscular temperatures. If the skin temperature falls below 33°C, warm the limbs by immersion in warm water or by application of warming packs or a hydrocollator. Adding 5% of the calculated conduction velocity for each degree below 33°C theoretically normalizes the result. The use of such conversion factors is based on evidence obtained in healthy persons, however, and may not be applicable in patients with abnormal nerves.
Anomalies
Martin-Gruber Anastomosis
In the Martin-Gruber anastomosis, anomalous fibers cross from the median to the ulnar nerve in the forearm. The communicating branches usually consist of motor axons supplying the ulnar innervated intrinsic hand muscles, particularly the first dorsal interosseous muscle, the hypothenar muscles (abductor digiti minimi), and the thenar muscles (adductor pollicis, deep head of flexor pollicis brevis), or a combination of these muscles. The Martin-Gruber anastomosis occurs in approximately 15% to 20% of the population and sometimes is bilateral. This anomaly manifests as a drop in the ulnar CMAP amplitude between distal and proximal stimulation sites (simulating the appearance of conduction block on ulnar NCS recording from the abductor digiti minimi or first dorsal interosseous). With distal stimulation (at the wrist), the CMAP reflects all ulnar motor fibers, whereas proximal stimulation activates only the uncrossed fibers, which are fewer in number. This anomaly can be confirmed by median nerve stimulation at the elbow, which evokes a small CMAP from the abductor digiti minimi or first dorsal interosseous, which is not present on median nerve stimulation at the wrist. When anomalous fibers innervate the thenar muscles, stimulation of the median nerve at the elbow activates the nerve and the crossing ulnar fibers, resulting in a large CMAP, often with an initial positivity caused by volume conduction of action potential from the ulnar thenar muscles to the median thenar muscles. By contrast, distal median nerve stimulation evokes a smaller thenar CMAP without the positive dip because the crossed fibers are not present at the wrist. In addition, the median nerve conduction velocity in the forearm is spuriously fast, particularly in the presence of carpal tunnel syndrome because the CMAP onset represents a different population of fibers at the wrist than at the elbow. Collision studies obtain an accurate conduction velocity by using action potentials of the crossed fibers (Sander et al., 1997).
Accessory Deep Peroneal Nerve
About 20% to 30% of subjects have an anomalous deep peroneal nerve. It is a branch of the superficial peroneal nerve and usually arises as a continuation of the muscular branch that innervates the peroneus longus and brevis muscles. It passes behind the lateral malleolus and terminates in the extensor digitorum brevis (EDB) on the dorsum of the foot. During peroneal motor NCS recording from the EDB, the peroneal CMAP amplitude is larger-stimulating proximally than distally because the anomalous fibers are not present at the ankle. Stimulation behind the lateral malleolus confirms this anomaly, which yields a small CMAP that approximately equals the difference between the CMAP amplitudes evoked with distal and proximal peroneal nerve stimulations. Complete innervation of the EDB by the accessory deep peroneal nerve is rare but should be suspected if there is preservation of function in the EDB muscle (i.e., extension of lateral toes) in a patient with severe deep peroneal neuropathy (Kayal and Katirji, 2009).
Temporal Dispersion and Phase Cancellation
The CMAP, evoked by supramaximal stimulation, represents the summation of all individual MUAPs directed to the muscle through the stimulated nerve. Typically, as the stimulus site moves proximally, the CMAP slightly drops in amplitude and area and increases in duration. The cause is temporal dispersion in which the velocity of impulses in slow-conducting fibers lags increasingly behind those of fast-conducting fibers as conduction distance increases. With dispersion, a slight positive and negative phase overlap, and cancellation of MUAP waveforms is seen (Fig. 32B.5). The result of temporal dispersion and phase cancellation is a reduction of CMAP amplitude and area and prolongation of its duration.
Physiological temporal dispersion affects the SNAP more than the CMAP (Fig. 32B.6). This difference relates to two factors. The first relates to the disparity between sensory fiber and motor fiber conduction velocities. The range of conduction velocities between the fastest and the slowest individual human myelinated sensory axons is almost twice that for the motor axons (25 m/sec and 12 m/sec, respectively). The second factor is the difference in duration of individual unit discharges between nerve and muscle. With short-duration biphasic sensory spikes, a slight latency difference could line up the positive peaks of the fast fibers with the negative peaks of the slow fibers and cancel both (Fig. 32B.7). In longer-duration MUAPs, the same latency shift would only partially superimpose peaks of opposite polarity, and phase cancellation would be less of a factor.
Electrodiagnosis by Nerve Conduction Studies
Focal Nerve Lesions
The classification of peripheral nerve lesions follows. In neurapraxia (first-degree injury), distortion of myelin occurs near the nodes of Ranvier, producing segmental conduction block without wallerian degeneration. In axonotmesis (second-degree injury), the axon is interrupted, but all the supporting nerve structures remain intact. In neurotmesis, the nerve injury is severe, resulting in complete disruption of the nerve with all the supporting structures (see Chapter 52D). Often, the neurotmesis category is divisible as follows: third-degree injury, with disruption of the endoneurium and with intact perineurium and epineurium; fourth-degree injury, with disruption of all neural elements except the epineurium; and fifth-degree nerve injury, with complete nerve transection resulting in complete discontinuity of the nerve. Electrodiagnostic studies alone cannot accurately distinguish among the five degrees of nerve injuries, but they can separate the first (neurapraxia) from the other types (Wilbourn, 2002).
Demyelinative Mononeuropathy
When focal injury to myelin occurs, conduction along the affected nerve fibers may alter. This may result in conduction slowing or block along the nerve fibers. The cause of conduction block is interruption of action potential transmission across the nerve lesion; it is the electrophysiological correlate of neurapraxia and usually results from loss of more than one myelin segment (segmental or internodal demyelination). Bracketing two stimulation points, one distal and one proximal to the site of injury, best localizes a nerve lesion with conduction block. With such lesions, stimulation distal to the lesion elicits a normal CMAP, whereas proximal stimulation evokes a response with reduced amplitude or fails to evoke any response, defined as partial or complete conduction block, respectively (Fig. 32B.8, A). Several limitations exist to the diagnosis of demyelinative conduction block:
1. Phase cancellation between peaks of opposite polarity may reduce CMAP size because of abnormally increased temporal dispersion. Such excessive desynchronization often develops in acquired demyelinative neuropathies. If the distal and the proximal responses have dissimilar waveforms, the discrepancy in amplitude or area between the two may be the result of phase cancellation rather than conduction block. Therefore, for a diagnosis of partial conduction block, findings should include a significantly lower CMAP amplitude and smaller CMAP area with stimulation proximal to the injury site than with the CMAP distal to it, and without any significant prolongation of CMAP duration. More than 50% decay of both the CMAP amplitude and area across the lesion usually is the criterion for definite conduction block.
2. Distal demyelinating lesions causing conduction block of the nerve segment between the most distal stimulating point and the recording site manifest as unelicitable or low CMAP amplitudes at both distal and proximal stimulation sites. This finding mimics the NCS seen with axonal degeneration.
3. The prominent temporal dispersion normally seen in evaluating SNAPs precludes the use of these potentials to diagnose conduction block.
4. Conduction block also may follow axonal loss before the completion of wallerian degeneration.
Focal slowing of conduction usually is the result of widening of the nodes of Ranvier (paranodal demyelination). Slowing, often synchronized, affects all large myelinated fibers equally. This results in prolongation of distal latency if the focal lesion is distal (see Fig. 32B.8, B,a), or slowing in conduction velocity if the focal lesion is proximal (see Fig. 32B.8, B,b). CMAP amplitude, duration, and area, however, are normal and do not change when the nerve is stimulated proximal to the lesion. Desynchronized slowing (differential slowing) occurs when conduction velocity reduces at the lesion site along a variable number of the medium or small nerve fibers (average- or slower-conducting axons). Here, the CMAP disperses with prolonged duration on stimulations proximal to the lesion. The speed of conduction along the injury site (latency or conduction velocity) is normal because of sparing of at least some of the fastest-conducting axons (see Fig. 32B.8, C). When synchronized and desynchronized slowing coexists, slowing of distal latency or conduction velocity accompanies the dispersed CMAP with prolonged duration.
Axon-Loss Mononeuropathy
Soon after axonal transection (i.e., for the first 48 hours), the distal axon remains excitable. Therefore, stimulation distal to the lesion elicits a normal CMAP, whereas proximal stimulation elicits a response with reduced amplitude, producing a conduction block pattern (see Fig. 32B.8, D, middle panel). This pattern is axonal noncontinuity, early axon loss, or axon-discontinuity conduction block. Soon, however, the distal axons undergo wallerian degeneration, and the distal CMAP decreases to equal the proximal CMAP (see Fig. 32B.8, D, lower panel). With wallerian degeneration, the distal CMAP decreases in amplitude starting 1 or 2 days after nerve injury and reaches its nadir in 5 to 6 days. In contrast, the distal SNAP lags slightly behind and reaches its nadir in 10 or 11 days (Fig. 32B.9). The difference between the decline of the SNAP and CMAP amplitudes after axon loss probably relates to neuromuscular transmission failure, which affects only the CMAP amplitude. Supporting this hypothesis is the fact that MNAPs recorded directly from nerve trunks follow the time course of SNAPs.
The study is repeated after 10 or 11 days, when degenerating axons have lost excitability, to distinguish between conduction block due to demyelination and that due to axon loss. A reduction in amplitude of the evoked potential from stimulation above and below the lesion indicates axonal loss (see Fig. 32B.8, D). By contrast, if the distally evoked CMAP still has significantly higher amplitude than that of the proximally elicited response, this indicates partial segmental demyelination.
Generalized Polyneuropathies
Axonal Polyneuropathies
Axonal polyneuropathies produce length-dependent dying-back degeneration of axons. The major change on NCS is decrease of the CMAP and SNAP amplitudes, more marked in the lower extremities. By contrast, conduction velocities and distal latencies usually are normal (Fig. 32B.10, B). As with axon-loss mononeuropathies, selective loss of many fast-conducting fibers associated with more than a 50% reduction in CMAP amplitude can slow conduction velocity to 70% to 80% of normal value.
Demyelinating Polyneuropathies
The hallmark of demyelinating polyneuropathies is a widespread increase in conduction time caused by impaired saltatory conduction. Therefore, NCS findings are characterized by significant slowing of conduction velocities (less than 75% of the lower limit of normal) and distal latencies (>130% of the upper limit of normal). With distal stimulation, demyelination delays the distal latency, and there is usually moderate reduction of the CMAP amplitude because of abnormal temporal dispersion and phase cancellation. With proximal stimulation, the CMAP amplitude is lower, and the proximal conduction velocity markedly slows because the action potentials travel a longer distance, with increased probability for the nerve action potentials to pass through demyelinated segments (see Fig. 32B.10, C). The proximal CMAP amplitude decay is the result of more prominent temporal dispersion and phase cancellation as well as possible conduction block along some fibers.
In the most common form of Guillain-Barré syndrome, acute inflammatory demyelinating polyneuropathy, multifocal demyelination that fulfills the criteria for demyelination is evident in 35% to 50% of patients during the first 2 weeks of illness, compared with 85% by the third week (Al-Shekhlee et al., 2005; Albers et al., 1985). Two other suggestive nerve conduction findings in this disorder are abnormal upper extremity SNAPs with normal sural SNAPs, an unusual pattern in axonal length–dependent polyneuropathy, and diffuse absence of F waves with normal results on motor conduction studies, findings consistent with proximal peripheral nerve or spinal root involvement.
Needle Electromyographic Examination
Principles and Techniques
1. Inserting or slightly moving the needle causes insertional activity that results from needle injury of muscle fibers.
2. Moving the needle a small distance and pausing a few seconds assesses spontaneous activity in relaxed muscle. From a single cutaneous insertion, relocating the needle in four quadrants of the muscle completes the evaluations.
3. Minimal contraction assesses the morphology of several MUAPs measured on the oscilloscope or hard copy. The needle should be moved slightly (pulled back or moved deeper) if sharp MUAPs are not seen with minimal contraction.
4. Increasing the intensity of muscle contraction assesses the recruitment pattern of MUAPs. Maximal contraction normally fills the screen, producing the interference pattern.
Insertional and Spontaneous Activity
Normal Insertional and Spontaneous Activity
At rest, muscle is silent, with no spontaneous activity except in the motor end-plate region, the site of neuromuscular junctions, which usually are located along a line crossing the center of the muscle belly. Table 32B.1 lists normal and abnormal insertional and spontaneous activities (Katirji et al., 2002). Two types of normal end-plate spontaneous activity occur together or independently: end-plate noise and end-plate spikes (Fig. 32B.11).
Abnormal Insertional and Spontaneous Activity
Fibrillation Potentials (
See Videos 35B.3 and 35B.4, available at www.expertconsult.com.)
Fibrillation potentials are spontaneous action potentials of denervated muscle fibers. They result from reduction of the resting membrane potential of the denervated fiber to the level at which it can fire spontaneously. Fibrillation potentials, triggered by spontaneous oscillations in the muscle fiber membrane potential, typically fire in a regular pattern at a rate of 1 to 30 Hz. The sound they produce on the loudspeaker is crisp and clicking, reminiscent of rain on a tin roof or the tick-tock of a clock. Fibrillation potentials have two types of waveforms: brief spikes and positive waves. Brief spikes usually are triphasic with initial positivity (Fig. 32B.12, A). They range from 1 to 5 msec in duration and 20 to 200 µV in amplitude when recorded with a concentric needle electrode. Brief-spike fibrillation potentials may be confused with physiological end-plate spikes but are distinguishable by their regular firing pattern and triphasic configuration with an initial positivity. Occasionally, placement of the needle electrode near the end-plate zone of a denervated muscle results in brief spikes, morphologically resembling end-plate spikes with an initial negativity. Positive waves have an initial positivity and subsequent slow negativity with a characteristic sawtooth appearance (see Fig. 32B.12, B). Making recordings near the damaged part of the muscle fiber (incapable of generating an action potential) accounts for the absence of a negative spike. Although usually seen together, positive sharp waves tend to precede brief spikes after nerve section, possibly because insertion of a needle in already irritable muscle membrane triggers the response.
Myotonic Discharges (
See Video 35B.6, available at www.expertconsult.com.)
Myotonic discharge, a special type of abnormal insertional activity, appears either as a sustained run of sharp positive waves, each followed by a slow negative component of longer duration, or as a sustained run of negative spikes with a small initial positivity (see Fig. 32B.12, C). Myotonic discharges are recurring single-fiber potentials showing, as with fibrillation potentials, two types of waveforms depending on the spatial relationship between the recording surface of the needle electrode and the discharging muscle fibers. Needle insertion injuring muscle membranes usually initiates positive waves, whereas the negative spikes, resembling the brief spike form of fibrillation potentials, tend to occur at the beginning of slight volitional contraction. Both positive waves and negative spikes typically wax and wane in amplitude over the range of 10 µV to 1 mV, varying inversely to the rate of firing. Their frequency ranges from 20 to 150 Hz and gives rise to a characteristic noise over the loudspeaker, simulating an accelerating or decelerating motorcycle or chainsaw.
Myokymic Discharges (
See Video 35B.7, available at www.expertconsult.com.)
Myokymia results from complex bursts of grouped repetitive discharges in which motor units fire repetitively, usually with 2 to 10 spikes discharging at a mean of 30 to 40 Hz (see Fig. 32B.12, D). Each burst recurs at regular intervals of 1 to 5 seconds, giving the sound of marching soldiers on the loudspeaker. Clinically, myokymic discharges often give rise to sustained muscle contractions, which have an undulating appearance beneath the skin (“bag of worms”). The origin of myokymic discharges probably is ectopic, in motor nerve fibers, and amplified by increased axonal excitability, such as after hyperventilation-induced hypocapnia.
Complex Repetitive Discharges (
See Video 35B.8, available at www.expertconsult.com.)
A complex repetitive discharge results from the nearly synchronous firing of a group of muscle fibers. One fiber in the complex serves as a pacemaker, driving one or several other fibers ephaptically so that the individual spikes in the complex fire in the same order in which the discharge recurs. One of the late-activated fibers reexcites the principal pacemaker to repeat the cycle. The entire sequence recurs at slow or fast rates, usually in the range of 5 to 100 Hz. The discharge ranges from 50 µV to 1 mV in amplitude and up to 50 to 1000 msec in duration. The complex waveform contains several distinct spikes and remains uniform from one discharge to another (see Fig. 32B.12, E). These discharges typically begin abruptly, maintain a constant rate of firing for a short period, and cease as abruptly as they started when the chain reaction eventually blocks. They produce a noise on the loudspeaker that mimics the sound of a machine or a motorcycle.
Neuromyotonic Discharges (
See Video 35B.9, available at www.expertconsult.com.)
Neuromyotonic discharges are extremely rare discharges in which muscle fibers fire repetitively with a high intraburst frequency (40 to 350 Hz), either continuously or in recurring decrementing bursts, producing a pinging sound on the loudspeaker. The discharges are more prominent in distal than proximal muscles, probably implicating the terminal branches of motor axons as the site of generation (Maddison et al., 2006). Many cases of neuromyotonia are associated with the syndrome of continuous motor unit activity or acquired neuromyotonia, an autoimmune antibody-mediated peripheral nerve potassium channelopathy (Hart et al., 1997). Other conditions that may be associated with neuromyotonia include anticholinesterase poisoning, tetany, and chronic spinal muscular atrophies.
Voluntary Motor Unit Action Potentials
MUAP Morphology
Duration (
See Videos 35B.11 to 35B.13, available at www.expertconsult.com.)
Long-duration MUAPs often are of high amplitude and are the best indicators of reinnervation, as seen with LMN disorders, peripheral neuropathies, and radiculopathies. They occur with increased number or density of muscle fibers or a loss of synchrony of fiber firing within a motor unit. Short-duration MUAPs often are of low amplitude. They occur in disorders associated with loss of muscle fibers, as seen with necrotizing myopathies (Fig. 32B.13).
MUAP Stability
(See Video 32B.16, available at www.expertconsult.com.)
Motor units normally discharge semirhythmically, with successive MUAPs showing nearly identical configuration because all muscle fibers of the motor unit fire during every discharge. The morphology of a repetitively firing unit may fluctuate if individual muscle fibers intermittently block within the unit. Moment-to-moment MUAP variability indicates deficient neuromuscular transmission as recurring discharges deplete the store of immediately available acetylcholine (Fig. 32B.14). This instability occurs in neuromuscular junction disorders such as myasthenia gravis, the Lambert-Eaton myasthenic syndrome, and botulism, as well as in neurogenic disorders associated with recent reinnervation, such as motor neuron disease, subacute radiculopathy, and polyneuropathy, and during the early stages of reinnervation in acute peripheral nerve injuries.
MUAP Firing Patterns
(See Videos 32B.17 and 32B.18, available at www.expertconsult.com.)
During constant contraction in a healthy person, initially only one or two motor units activate semirhythmically. The motor units activated early are primarily those with small type I muscle fibers. Large type II units participate later during strong voluntary contraction. Greater muscle force brings about not only recruitment of previously inactive units but also more rapid firing of already active units, with both mechanisms operating simultaneously (Erim et al., 1996).
Electrodiagnosis by Needle Electromyography
Lower Motor Neuron Disorders
MUAPs are normal in morphology in the acute phase of denervation, but signs of reinnervation become apparent as early as 1 month later. Reinnervation causes first an increased number of MUAP turns and phases and later increased MUAP amplitude and duration. Amplitude generally reflects fiber density, whereas duration reflects motor unit territory. The expected MUAP from LMN lesions is a long-duration, high-amplitude, and polyphasic unit (Fig. 32B.15; see also Fig. 32B.13). The exception is in early reinnervation in which motor units acquire few muscle fibers, resulting in brief, small, polyphasic MUAPs (“nascent” MUAPs), mimicking a myopathic process.
Radiculopathies
Needle EMG is the most sensitive and specific electrodiagnostic test for identifying cervical and lumbosacral radiculopathies, particularly those associated with axon loss. Needle EMG is useful for accurate localization of the level of the root lesion. Finding signs of denervation (fibrillation potentials, decreased recruitment, and long-duration, high-amplitude polyphasic MUAPs) in a segmental myotomal distribution (i.e., in muscles innervated by the same roots via more than one peripheral nerve), with or without denervation of the paraspinal muscles, localizes the LMN lesion to the root level (Wilbourn and Aminoff, 1998). In radiculopathies associated with axonal loss of proximal sensory fibers, the distal sensory axons do not degenerate, because the unipolar neurons of dorsal root ganglia and their distal axons usually escape injury. Hence, a normal SNAP of the corresponding dermatome ensures that the root lesion is within the spinal canal (i.e., proximal to the dorsal root ganglia). For example, in an L5 radiculopathy, the tibialis anterior (peroneal nerve) and tibialis posterior (tibial nerve) muscles often are abnormal on needle EMG, as may be those from the lumbar paraspinal muscles, but the superficial peroneal SNAP usually is normal.
Plexopathies
Plexopathies are lesions that involve extraspinal peripheral nerves. The diagnosis of brachial or lumbosacral plexopathies requires a solid knowledge of peripheral nerve anatomy. Brachial plexus anatomy is particularly complex, so that multiple NCS and muscle needle EMGs are needed for evaluation. An important task of the electrodiagnostic evaluation is to differentiate between lesions affecting the brachial plexus (postganglionic lesions) and those involving the roots (preganglionic lesions). This distinction is particularly important in brachial plexus traction injuries, which may mimic root avulsions (Ferrante and Wilbourn, 2002). In avulsions, the dorsal root ganglia remain intact, and their peripheral axons do not undergo wallerian degeneration. Therefore, root avulsions spare SNAPs, whereas SNAPs are low in amplitude or absent in brachial plexopathies when studied after the completion of wallerian degeneration (more than 10 days from injury).
Mononeuropathies
The principle of localizing an axon-loss mononeuropathy by needle EMG is similar to manual muscle strength testing on clinical examination. Typically, the needle EMG reveals neurogenic changes (fibrillation potentials, reduced MUAP recruitment, and chronic neurogenic MUAP morphology changes) that are limited to muscles innervated by the involved nerve and located distal to the site of the lesion. Localization of axon-loss peripheral nerve lesions by needle EMG is suboptimal, however, because some nerves have very long segments from which no motor branches arise, such as the median and ulnar nerves in the arm or the common peroneal nerve in the thigh. In addition, needle EMG may falsely localize a partial nerve lesion more distally along the affected nerve because of fascicular involvement of nerve fibers or effective reinnervation of proximally situated muscles (Wilbourn, 2002). An example is sparing of ulnar muscles in the forearm (flexor carpi ulnaris and ulnar part of flexor digitorum profundus) following an axon-loss ulnar nerve lesion at the elbow.
Needle EMG is particularly useful in assessing the progress of reinnervation occurring spontaneously or after nerve repair. MUAP recruitment and morphology help assess the process of muscle fiber reinnervation that occurs with proximodistal regeneration of nerve fibers from the site of the injury or collateral sprouting. Early proximodistal regeneration of nerve fibers in severe axon-loss lesions often manifests as brief, small, polyphasic (nascent) MUAPs. Collateral sprouting causes an increased number of MUAP turns and phases, followed by an increased duration and amplitude of MUAPs (Katirji, 2006).
Anterior Horn Cell Disorders
One disadvantage of needle EMG in motor neuron disease is that it evaluates only LMN degeneration; UMN degeneration requires clinical assessment. Therefore, clinical evaluation is the basis for diagnosing amyotrophic lateral sclerosis (ALS), with the electrodiagnostic studies playing a supporting role. The reasons to perform such studies in patients with suspected ALS are to (1) confirm LMN dysfunction in clinically affected regions, (2) detect evidence of LMN dysfunction in clinically uninvolved regions, and (3) exclude other pathophysiological processes such as multifocal motor neuropathy or chronic myopathy (Chad, 2002).
Although LMN degeneration in ALS may ultimately affect the entire neuraxis (brainstem and cervical, thoracic, and lumbosacral segments of spinal cord), participation in clinical trials requires early diagnosis. Lambert’s initial criteria of fibrillation and fasciculation potentials detected in muscles of the legs and arms or in the limbs and the head were stringent. These criteria evolved into active and chronic denervation detected in at least three extremities or two extremities and cranial muscles (with the head and neck considered an extremity). The revised El Escorial criteria recommended that needle EMG signs of LMN degeneration be present in at least two of the four central nervous system regions (i.e., the brainstem, cervical, thoracic, and lumbosacral regions) (Brooks et al., 2000). Though rigid requirement of signs of chronic denervation and reinnervation as well as active denervation in the form of fibrillation potentials had been useful, recent consensus recommends including fasciculation potentials when seen in a muscle with chronic neurogenic changes as evidence equivalent in importance to the presence of fibrillation potentials (de Carvalho et al., 2008).
Upper Motor Neuron Lesions
In patients with UMN lesions, electrodiagnostic studies show normal insertional activity, no spontaneous activity at rest, and normal MUAP morphology. The only abnormality is a reduced interference pattern and a poor activation with a slow rate of motor unit discharge (see Fig. 32B.15). Recruitment measured by either recruitment frequency or ratio is normal. Nonphysiological weakness or poor effort produces a similar pattern, except that motor unit firing may be irregular.
Myopathic Disorders
Insertional activity usually is normal except in the late stage of muscular dystrophies, when it is reduced secondary to atrophy and fibrosis. Fibrillation potentials usually are absent, except in necrotizing myopathies such as inflammatory myopathies and muscular dystrophies (see Fibrillation Potentials). Random loss of fibers from the motor unit leads to a reduction of MUAP amplitude and duration (see Fig. 32B.13). Regeneration of muscle fibers sometimes gives rise to long-duration spikes and satellite potentials. Early recruitment is the rule because of the need for more motor units to maintain a given force in compensation for the small size of individual units (see Fig. 32B.15).
A disadvantage of the electrodiagnostic study of myopathies is that the needle EMG findings are not always specific enough to make a final diagnosis (Table 32B.2). Exceptions include conditions associated with (1) myotonia, such as the myotonic dystrophies, myotonia congenita, paramyotonia congenita, hyperkalemic periodic paralysis, acid maltase deficiency, and some toxic myopathies (such as from colchicine), and (2) fibrillation potentials, which occur in necrotizing myopathies such as inflammatory myopathies and progressive muscular dystrophies (such as Becker and Duchenne muscular dystrophies). Another disadvantage of the needle EMG is that findings either are normal or include subtle abnormalities in some myopathies, such as the metabolic and endocrine myopathies (Lacomis, 2002). Therefore, normal findings on the needle EMG do not exclude a myopathy.
In polymyositis and dermatomyositis, it is essential to recognize the changing pattern on the needle EMG at diagnosis, after treatment, and during relapse. Fibrillation potentials appear first at diagnosis or relapse and disappear early during remission. Abnormal MUAP morphology becomes evident later and takes longer to resolve. The presence of fibrillation potentials also is helpful in distinguishing exacerbation of myositis from a corticosteroid-induced myopathy (Wilbourn, 1993).
Specialized Electrodiagnostic Studies
F Wave
A supramaximal stimulus applied at any point along the course of a motor nerve elicits a small, late, motor response (F wave) after the CMAP (M response). The F wave derives its name from foot—the first recording was from the intrinsic foot muscles. The nerve action potential initiated during a motor nerve conduction study travels in two directions: distally (orthodromically) to depolarize the muscle and generate a CMAP, and proximally (antidromically), toward the spinal cord, to trigger an F wave. The long-latency F wave is a very small CMAP that results from backfiring of antidromically activated anterior horn cells, averaging 5% to 10% of the motor neuron pool. The F wave’s afferent and efferent loops are the motor neuron, with no intervening synapse (Fisher, 2002). The F wave varies in latency, morphology, and amplitude with each stimulus because a different population of anterior horn cells backfires. Therefore, an adequate study requires that about 10 F waves be clearly identified (Fig. 32B.16, A). Moving the stimulator proximally decreases the F wave latency because the action potential travels a shorter distance.
The F-wave minimal latency, measured from the stimulus artifact to the beginning of the evoked potential, is the most reliable and useful measurement and represents conduction of the largest and fastest motor fibers. The minimal F-wave latency depends on the length of the nerve studied (see Fig. 32B.16, B). The most sensitive criterion of abnormality in a unilateral disorder affecting a single nerve is a minimum latency difference between the two sides or between two nerves in the same limb. Absolute latencies are useful only for sequential reassessment of the same nerve.
Prolonged F-wave minimal latencies occur in most polyneuropathies, particularly the demyelinating type. In the early phases of Guillain-Barré syndrome, findings on routine motor nerve studies may be normal except for prolonged or absent F responses, which imply proximal demyelination (Al-Shekhlee et al., 2005; Gordon and Wilbourn, 2001). F-wave latencies in radiculopathies have limited use. They may be normal despite partial motor axonal loss, and most muscles have multiple root innervations (Wilbourn and Aminoff, 1998).
A Wave
The A wave (axonal wave) is a potential seen occasionally during recording of F waves at supramaximal stimulation. The A wave follows the CMAP and often precedes, but occasionally follows, the F wave. The A wave may be seen in asymptomatic persons during studies of the tibial nerve. It may be mistaken for an F wave, but its constant latency and morphology differentiate it from the highly variable morphology and latency of the F wave (see Fig. 32B.16, B). A waves sometimes are seen in axon-loss polyneuropathies, motor neuron disease, and radiculopathies, whereas multiple or complex A waves often are associated with acquired or inherited demyelinating polyneuropathies. The exact pathway of the A wave is unknown; the constant morphology and latency of the A wave are best explained by the fixed point of a collateral reinnervating sprout or an ephapse between two axons.
H Reflex
The H reflex, named after Hoffmann for his original description, is an electrical counterpart of the stretch reflex elicited by a mechanical tap to the tendon. The group 1A sensory fibers and alpha motor neurons form the respective afferent and efferent arcs of this predominantly monosynaptic reflex. The H reflex and the F wave can be distinguished by increasing stimulus intensity. The H reflex is best elicited by a long-duration stimulus, submaximal to produce an M response (Fig. 32B.17), whereas the F wave requires supramaximal stimulus intensity. In contrast with the F wave, which can be elicited from any limb muscle, the H reflex from stimulating the tibial nerve while recording the soleus muscle (S1 arc reflex) is the most reproducible and commonly used in clinical practice. Absent H reflexes are very common although not specific in the early phases of Guillain-Barré syndrome (Al-Shekhlee et al., 2005; Gordon and Wilbourn, 2001) and in peripheral polyneuropathy. An asymmetrically absent or side-to-side latency difference greater than 1.5 msec or amplitude difference of more than 50% is common in S1 radiculopathy (Nishida et al., 1996).
Repetitive Nerve Stimulation
Principles
A quantum is the amount of acetylcholine in a single vesicle, which is approximately 5000 to 10,000 acetylcholine molecules. Each quantum (vesicle) released results in a 1-mV change of postsynaptic membrane potential. This occurs spontaneously during rest and forms the basis of the miniature end-plate potential.
The number of quanta released after a nerve action potential depends on the number of quanta in the immediately available (i.e., primary) store and the probability of release: m = p × n, where m = the number of quanta released during each stimulation, p = the probability of release (effectively proportional to the concentration of calcium and typically about 0.2, or 20%), and n = the number of quanta in the immediately available store. In normal conditions, a single nerve action potential triggers the release of 50 to 300 vesicles (quanta), with an average equivalent to about 60 quanta (60 vesicles). In addition to the immediately available store of acetylcholine located beneath the presynaptic nerve terminal membrane, a secondary (or mobilization) store starts to replenish the immediately available store after 1 to 2 seconds of repetitive nerve action potentials. A large tertiary (or reserve) store also is available in the axon and cell body.
The end-plate potential is the potential generated at the postsynaptic membrane after a nerve action potential. Because each vesicle released causes a 1-mV change in the postsynaptic membrane potential, this results in an approximately 60-mV change in the amplitude of the membrane potential.
In normal conditions, the number of quanta (vesicles) released at the neuromuscular junction by the presynaptic terminal far exceeds the postsynaptic membrane potential change necessary to reach the threshold needed to generate a postsynaptic muscle action potential. This is the basis of the safety factor, which results in an end-plate potential that is always above threshold and able to generate a muscle fiber action potential. In addition to quantal release, other factors that contribute to the safety factor and the end-plate potential include acetylcholine receptor conduction properties, acetylcholine receptor density, and acetylcholinesterase activity (Boonyapisit et al., 1999).
Voltage-gated calcium channels open after depolarization of the presynaptic terminal, leading to calcium influx. Through a calcium-dependent intracellular cascade, vesicles dock into active release zones, releasing acetylcholine molecules. Calcium then diffuses slowly out of the presynaptic terminal in 100 to 200 msec. The rate at which motor nerves are repetitively stimulated dictates whether or not calcium accumulation plays a role in enhancing the release of acetylcholine. At slow rate of RNS (i.e., a stimulus every 200 msec or more; or a stimulation rate less than 5 Hz), the calcium role in acetylcholine release is not increased, and subsequent nerve action potentials reach the nerve terminal long after calcium has dispersed. By contrast, with rapid RNS (i.e., a stimulus every 100 msec or less; a stimulation rate greater than 10 Hz), calcium influx is greatly increased, and the probability of release of acetylcholine quanta increases.
Slow Repetitive Nerve Stimulation
In normal conditions, slow RNS does not cause a CMAP decrement. Although the second through fifth end-plate potentials fall in amplitude, they remain above threshold (because of the normal safety factor) and ensure muscle fiber action potential generation after each stimulation. In addition, the secondary store begins to replace the depleted quanta after the first few seconds, with a subsequent rise in the end-plate potential. Therefore, all muscle fibers generate muscle fiber action potentials, and the CMAP does not change in size. In postsynaptic neuromuscular junction disorders (such as myasthenia gravis), the safety factor is reduced because fewer acetylcholine receptors are available. Therefore, the baseline end-plate potential reduces but usually is still above threshold. Slow RNS results in a decrease in end-plate potential amplitudes at many neuromuscular junctions. As end-plate potentials decline below the threshold, the number of muscle fiber action potentials produced declines, leading to a CMAP decrement (Fig. 32B.18). In presynaptic neuromuscular junction disorders (such as Lambert-Eaton myasthenic syndrome), the baseline end-plate potential is low, with many end-plates not reaching threshold. Therefore, many muscle fibers do not fire, resulting in low baseline CMAP amplitude (Table 32B.3). With slow RNS, further CMAP decrement occurs, caused by the further decline of acetylcholine release with the subsequent stimuli, resulting in further loss of many end-plate potentials and muscle fiber action potentials (Katirji and Kaminski, 2002).
1. Obtain slow RNS at rest and after exercise. If a reproducible CMAP decrement (less than 10%) appears at rest, slow RNS should be repeated after the patient exercises for 10 seconds to demonstrate repair of the decrement (posttetanic facilitation). If no or equivocal (less than 10%) decrement occurs at rest, the patient should perform maximal voluntary exercise for 1 minute. Then, repeat slow RNS every 30 seconds afterward and for 3 to 5 minutes after exercise. Because the amount of acetylcholine released with each stimulus is at its minimum 2 to 5 minutes after exercise, slow RNS after exercise increases the chance of detecting a defect of neuromuscular transmission at the neuromuscular junction by demonstrating a worsening CMAP decrement (postexercise exhaustion).
2. Record from clinically weakened muscles. Most commonly used and technically feasible nerves for slow RNS are the median, ulnar, and spinal accessory nerves. The diagnostic sensitivity is clearly higher for slow RNS recording in proximal muscles than in distal muscles. Facial nerve repetitive stimulation is indicated in patients with oculobulbar weakness (Zinman et al., 2006), but this study is technically difficult and sometimes associated with a large stimulation artifact that renders waveform interpretation subject to error.
3. Warm the extremity studied (skin temperature should be above 32°C). This precaution decreases false-negative results, because cooling improves neuromuscular transmission and may mask the decrement.
4. Discontinue cholinesterase inhibitors for 12 to 24 hours (if clinically possible). This measure also decreases the false-negative rate with slow RNS.
Rapid Repetitive Nerve Stimulation
In a presynaptic disorder such as Lambert-Eaton myasthenic syndrome, very few vesicles release, and many muscle fibers do not reach threshold, resulting in low baseline CMAP amplitude. With rapid RNS, the calcium concentrations in the nerve terminal increases high enough to enhance release of a sufficient number of synaptic vesicles to result in a larger end-plate potential that crosses threshold and is capable of action potential generation. This leads to many muscle fibers firing and results in a CMAP increment (see Table 32B.3). The increment often is higher than 200% in Lambert-Eaton myasthenic syndrome (Fig. 32B.19), with 10-second postexercise facilitation achieving the highest diagnostic sensitivity (Hatanaka and Oh, 2008). Patients with botulism have a less pronounced increment, ranging from 40% to 200%, due to the more severe neuromuscular blockade (Witoonpanich R et al., 2009). In a postsynaptic disorder such as myasthenia gravis, rapid RNS causes no change of CMAP, because the depleted stores are compensated by calcium influx. In severe postsynaptic blockade (such as during myasthenic crisis), the increased quantal release cannot compensate for the marked neuromuscular block, resulting in a drop in end-plate potential amplitude. Therefore, fewer end-plates reach threshold, and fewer muscle fiber action potentials are generated, resulting in CMAP decrement.
Single-Fiber Electromyography
The technical requirements for performing single-fiber EMG are as follows. First, a concentric single fiber needle electrode allows the recording of single muscle fiber action potentials. The small side port on the cannula of the needle serves as the pickup area. A single fiber needle electrode records from a circle of 300-mm radius, as compared with the l-mm radius of a conventional concentric EMG needle. Recent studies have shown no difference in sensitivity or specificity between the reusable single fiber and disposable concentric-needle electrodes (Sarrigiannis et al., 2006; Stålberg and Sanders, 2009). Second, the amplifier must have an impedance of 100 megohms or greater to counter the high electrical impedance of the small leadoff surface, the gain is set higher for single-fiber EMG recordings than for conventional EMG, the sweep speed is faster, and the filter should have a 500-Hz low frequency to attenuate signals from distant fibers. Third, an amplitude threshold trigger allows recording from a single muscle fiber, and a delay line permits viewing of the entire waveform even though the single-fiber potential triggers the sweep. Fourth, computerized equipment assists in data acquisition, analysis, and calculation.
Jitter
Jitter is the variability of the time interval between two muscle fiber action potentials (a muscle pair) innervated by the same motor unit. It is the variability of the interpotential intervals between repetitively firing paired single fiber potentials (Stålberg and Trontelj, 1997) (Fig. 32B.20). Neuromuscular jitter can be determined by using a commercially available computer program. The program calculates the mean value of consecutive interval differences over a number of 50 to 100 discharges, as follows:
Albers J.W., Donofrio P.D., McGonagle T.K. Sequential electrodiagnostic abnormalities in acute inflammatory demyelinating polyradiculoneuropathy. Muscle Nerve. 1985;8:528-539.
Al-Shekhlee A., Hachwi R.N., Preston D.C., et al. New criteria for early electrodiagnosis of acute inflammatory demyelinating polyneuropathy. Muscle Nerve. 2005;32:66-73.
Boonyapisit K., Kaminski H.J., Ruff R.L. The molecular basis of neuromuscular transmission disorders. Am J Med. 1999;106:97-113.
Brooks B.R., Miller R.G., Swash M., et alWorld Federation of Neurology Group on Motor Neuron Diseases. El Escorial revisited: revised criteria for the diagnosis of amyotrophic lateral sclerosis. Amyotroph Lateral Scler Other Motor Neuron Disord. 2000;1:293-299.
Chad D.A. Electrodiagnostic approach to the patient with suspected motor neuron disease. Neurol Clin. 2002;20:527-555.
de Carvalho M., Dengler R., Eisen A., et al. Electrodiagnostic criteria for diagnosis of ALS. Clin Neurophysiol. 2008;119:497-503.
Erim Z., de Luca C.J., Mineo K., et al. Rank-ordered regulation of motor units. Muscle Nerve. 1996;19:563-573.
Ferrante M.A., Wilbourn A.J. Electrodiagnostic approach to the patient with suspected brachial plexopathy. Neurol Clin. 2002;20:423-450.
Fisher M.A. H reflex and F waves. Fundamentals, normal and abnormal patterns. Neurol Clin. 2002;20:339-360.
Gordon P.H., Wilbourn A.J. Early electrodiagnostic findings in Guillain-Barré syndrome. Arch Neurol. 2001;58:913-917.
Hart I.K., Waters C., Vincent A., et al. Autoantibodies detected to expressed K+ channels are implicated in neuromyotonia. Ann Neurol. 1997;41:238-246.
Hatanaka Y., Oh S.J. Ten-second exercise is superior to 30-second exercise for post-exercise facilitation in diagnosing Lambert-Eaton myasthenic syndrome. Muscle Nerve. 2008;37:572-575.
Katirji B. The clinical electromyography examination. An overview. Neurol Clin. 2002;20:291-303.
Katirji B. Electrodiagnostic studies in the evaluation of peripheral nerve injuries. In: Evans R.W., editor. Neurology and Trauma. second ed. Oxford: Oxford University Press; 2006:386-401.
Katirji B., Kaminski H.J. Electrodiagnostic approach to the patient with suspected neuromuscular junction disorder. Neurol Clin. 2002;20:557-586.
Katirji B., Kaminski H.J., Preston D.C., et al. Neuromuscular Disorders in Clinical Practice. Boston: Butterworth Heinemann, 2002.
Kayal R., Katirji B. Atypical deep peroneal neuropathy in the setting of an accessory deep peroneal nerve. Muscle Nerve. 2009;40:313-315.
Kimura J. Facts, fallacies, and facies of nerve conduction studies: twenty-first annual Edward H. Lambert lecture. Muscle Nerve. 1997;20:777-787.
Lacomis D. Electrodiagnostic approach to the patient with suspected myopathy. Neurol Clin. 2002;20:587-603.
Maddison P., Mills K.R., Newsom-Davis J. Clinical electrophysiological characterization of the acquired neuromyotonia phenotype of autoimmune peripheral nerve hyperexcitability. Muscle Nerve. 2006;33:801-808.
McIntosh K.A., Preston D.C., Logigian E.L. Short segment incremental studies to localize ulnar entrapments at the wrist. Neurology. 1998;50:303-306.
Nishida T., Kompoliti A., Janssen I., et al. H reflex in S-1 radiculopathy: latency versus amplitude controversy revisited. Muscle Nerve. 1996;19:915-917.
Preston D.C., Shapiro B.E. Electromyography and Neuromuscular Disorders. Clinical-Electrophysiologic Correlations, second ed. Philadelphia: Elsevier/Butterworth Heinemann; 2005.
Rutkove S.B., Kothari M.J., Shefner J.M. Nerve, muscle, and neuromuscular junction electrophysiology at high temperature. Muscle Nerve. 1997;20:431-436.
Sander H.W., Quinto C., Chokroverty S. Median-ulnar anastomosis to thenar, hypothenar, and first dorsal interosseous muscles: collision technique confirmation. Muscle Nerve. 1997;20:1460-1462.
Sarrigiannis P.G., Kennett R.P., Read S., et al. Single-fiber EMG with a concentric needle electrode: validation in myasthenia gravis. Muscle Nerve. 2006;33:61-65.
Stålberg E.V., Sanders D.B. Jitter recordings with concentric needle electrodes. Muscle Nerve. 2009;40:331-339.
Stålberg E., Trontelj J.V. The study of normal and abnormal neuromuscular transmission with single fibre electromyography. J Neurosci Methods. 1997;74:145-154.
Wilbourn A.J. The electrodiagnostic examination with myopathies. J Clin Neurophysiol. 1993;10:132-148.
Wilbourn A.J. Nerve conduction studies. Types, components, abnormalities and value in localization. Neurol Clin. 2002;20:305-338.
Wilbourn A.J., Aminoff M.J. The electrodiagnostic examination in patients with radiculopathies. Muscle Nerve. 1998;21:1612-1631.
Witoonpanich R., Vichayanrat E., Tantisiriwit K., et al. Electrodiagnosis of botulism and clinico-electrophysiological correlation. Clin Neurophysiol. 2009;120:1135-1138.
Zinman L.H., O’Connor P.W., Dadson K.E., et al. Sensitivity of repetitive facial-nerve stimulation in patients with myasthenia gravis. Muscle Nerve. 2006;33:694-696.