CHAPTER 28 Cells of the Extracellular Matrix and Immune System
A remarkable variety of specialized cells populate the connective tissues of animals. These cells manufacture extracellular matrix, defend against infection, and maintain energy stores in the form of lipid (Fig. 28-1). Some of these cells arise in connective tissue and remain there. These indigenous cells are specialized: Fibroblasts make the collagen, elastic fibers, and proteoglycans of the extracellular matrix; chondrocytes secrete the matrix for cartilage; and osteoblasts manufacture the calcified matrix of bone. Fat cells store lipids, and mast cells secrete histamine and other mediators of inflammation. The remaining cells arise elsewhere, travel through blood and lymph, and enter connective tissue as needed, so they are known as immigrant cells. These visitors are part of the immune system, which defends against microorganisms. This chapter introduces all of these cells.
Indigenous Connective Tissue Cells
Primitive Mesenchymal Cells
Primitive mesenchymal cells are undifferentiated, multipotential stem cells (see Box 41-1) that proliferate and differentiate (Fig. 28-1) to give rise to all the indigenous cells of connective tissue (fibroblasts, fat cells, mast cells, chondrocytes, and osteoblasts). Small numbers of these inconspicuous precursor cells hide along the small blood vessels but cannot be identified by light microscopy. By electron microscopy (Fig. 28-2), mesenchymal cells resemble fibroblasts but with fewer organelles of the secretory pathway.
Fibroblasts
Fibroblasts are the connective tissue workhorses, synthesizing and secreting most of the macromolecules of the extracellular matrix (Fig. 28-2). Chapter 29 considers the synthesis of these matrix molecules in detail. Accordingly, mature fibroblasts have abundant rough endoplasmic reticulum and a large Golgi apparatus. They are generally spindle-shaped, with an oval, flattened nucleus, but can assume many other shapes depending on the mechanical forces in the surrounding matrix. The migratory patterns of the fibroblasts determine the patterns of collagen fibrils in tissues. In response to tissue damage, fibroblasts proliferate and migrate into the wound, where they synthesize new matrix to restore the integrity of the tissue (see Fig. 32-11).
Mast Cells
Mast cells are secretory cells that mediate “immediate hypersensitivity” reactions by secreting the contents of histamine-containing granules in response to insect bites or exposure to allergens, as in hay fever. Mast cells distribute along blood vessels in connective tissue (Fig. 28-3). The large, abundant granules contain, by mass, 30% heparin–basic protein complex, 10% histamine, and 35% basic proteins, including proteases. A variety of stimuli can induce secretion of the granule contents. The most specific stimulus operates through plasma membrane receptors for immunoglobulins of the immunoglobulin E (IgE) class. These receptors bind a random selection of soluble IgEs that the immune system makes in response to exposure to allergens. When the corresponding antigen binds to IgE on the surface of a mast cell, the receptors aggregate, triggering a cytoplasmic Ca2+ pulse (see Chapter 26) and fusion of granules with the plasma membrane (see Fig. 21-12). Mechanical trauma, radiant energy (heat, X-rays), and toxins or venoms are less specific stimuli but can also trigger secretion. Outside the cell, the carrier proteins release heparin and histamine. Histamine binds to cellular receptors, causing blood vessels to leak plasma, smooth muscle to contract, and itching sensations to occur. This results in the congestion and constriction of the respiratory tract in allergic reactions and swelling of the skin after an insect bite. Secreted fibrinolysin and heparin inhibit blood clotting. Stimulated mast cells also secrete tumor necrosis factor-a and eicosanoids, contributing to the activation of other inflammatory cells in chronic conditions including asthma and arthritis.
White Fat Cells
Fat cells (adipocytes) distributed in the connective tissue beneath the skin and in the abdominal mesentery of vertebrates store lipids as a readily accessible reserve of energy. These round cells vary in diameter depending on the size of their single, large, lipid droplet (Fig. 28-4) containing triglycerides, neutral lipids with a fatty acid esterified to all three carbons of glycerol (see Fig. 7-2 for the structures of glycerol and fatty acids). Intermediate filaments and endoplasmic reticulum separate the lipid droplet from the thin rim of cytoplasm. After a meal, fat cells take up fatty acids and glycerol from blood and synthesize triglycerides for storage. During fasting or when the body requires energy, enzymes called lipases hydrolyze fatty acids from triglycerides for release back into the blood for use by other organs. Hormones including epinephrine (see Fig. 27-3) regulate these metabolic reactions.
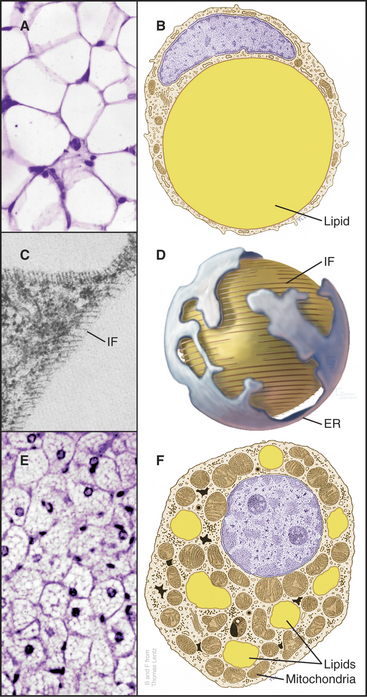
Figure 28-4 fat cells. A., Light micrograph of a section of white fat cells stained with hematoxylin and eosin. B, Drawing of a white adipose cell. C, Transmission electron micrograph of a thin section of the edge of a lipid droplet showing the circumferential sheath of vimentin intermediate filaments (IF [see Chapter 35). D, Interpretive drawing of a lipid droplet with its associated filaments and endoplasmic reticulum (ER). E, Light micrograph of a section of brown fat. F, Drawing of a brown fat cell.
(A, C, and E, Courtesy of D. W. Fawcett, Harvard Medical School, Boston, Massachusetts. B and F, Modified from T. Lentz, Yale Medical School, New Haven, Connecticut. D, Modified from Werner Franke, University of Heidelberg, Germany.)
Mutations in four different genes cause inherited lipodystrophies, human conditions with loss of fat tissue. Loss of function of an enzyme required to synthesize triglycerides or a nuclear receptor that stimulates differentiation of fat cells make sense. It is less clear why mutations in the gene for nuclear lamins A and C (see Fig. 14-9) or a protease that processes lamin A should cause loss of fat.
Brown Fat Cells
Brown fat cells derive their color from numerous mitochondria, which they use to generate heat in response to cold or (in lean rodents) to excess food intake. Cytochromes make mitochondria brown. Fat is stored in multiple, small droplets (Fig. 28-4F). Brown fat is less abundant than white fat, being concentrated in connective tissue between the scapulae in mammals. Newborn humans have more brown fat than do adults in order to generate heat during the adjustment to a new environment after birth. Hibernating animals use brown fat to raise their temperatures when emerging from hibernation.
Brown fat cells generate heat by short-circuiting the proton gradient that is usually used to generate adenosine triphosphate (ATP) in mitochondria (see Fig. 19-5). Sympathetic nerves acting through β-adrenergic receptors (see Fig. 27-3) and protein kinase A stimulate brown fat cells to express an “uncoupling protein” and to break down lipids to provide fatty acids for oxidation by mitochondria. Uncoupling protein inserts into the inner mitochondrial membrane and dissipates the proton electrochemical gradient across the inner membrane. Energy is lost as heat rather than being used to synthesize ATP. Thermogenesis may be an “energy buffer” that, when defective in animals, can contribute to obesity.
Origin and Development of Blood Cells
The blood of vertebrates contains a variety of cells, each with a specialized function (Fig. 28-5; Table 28-1). Red blood cells transport oxygen, platelets repair damage to blood vessels, and various types of white blood cells defend against infections. All blood cells derive ultimately from pluripotential stem cells (Fig. 28-5B; also see Box 41-1). After purification, these stem cells can restore the production of all blood cells in mice that have been irradiated to destroy their own blood cell precursors. Destruction of stem cells (e.g., by drugs such as chloramphenicol) leads to aplastic anemia, a condition in which few blood cells are produced, owing to a lack of precursors. These stem cells are also responsible for restoring blood cell production following human bone marrow transplantation.
Table 28-1 BLOOD CELLS (AS SEEN ON A STAINED SMEAR OF BLOOD)
Type | Concentration | Features |
---|---|---|
Platelets | 300,000/μL | Anucleate; 2–3 μm wide; purple granules |
Erythrocytes | ˜5 × 106/μL | 7-μm; diameter biconcave disks; no nucleus; pink cytoplasm |
Neutrophils | ˜60% of total WBCs | 10–12 μm wide; multilobed nucleus; many unstained granules; few azurophilic granules |
Eosinophils | ˜2% of total WBCs | Bilobed nucleus; numerous, large, refractile, pink-stained granules; ˜12 μm wide |
Basophils | ˜0.5% of total WBCs | Lobed nucleus; large, blue-stained granules; ˜10 μm wide |
Lymphocytes | ˜30% of total WBCs | Small, round, intensely stained nucleus; some small azurophilic granules; variable amount of clear blue cytoplasm, so they may be classified as either small (˜7–8 μm wide), medium, or large |
Monocytes | ˜5% of total WBCs | Up to 17 μm wide; large, indented nucleus and gray-blue cytoplasm with a few azurophilic granules |
WBCs, white blood cells.
Proliferation and differentiation of the progeny of pluripotential stem cells produce mature blood cells. At several stages in each line of cells, precursors undergo irreversible differentiation that commits them to a particular lineage. The first branch in the pathway of differentiation separates the precursors of lymphocytes from the precursors of the other blood cells called myeloid stem cells. Next, the myeloid stem cell differentiates into three different committed stem cells. One gives rise to red blood cells; another gives rise to megakaryocytes and platelets. Monocytes and the three types of granulocytes (neutrophils, eosinophils, and basophils) originate from a common committed stem cell in bone marrow and share a number of physiological features. Through differentiation, each also acquires unique functions. Platelets, red cells, granulocytes, and monocytes develop in bone marrow. Lymphocytes develop in bone marrow as well as in lymphoid tissues (thymus, spleen, and lymph nodes).
Minute quantities of specific glycoprotein growth factors control the balance between self-renewal and proliferation at each stage of development, starting with pluripotential stem cells. Feedback mechanisms control production of these growth factors. For example, the oxygen level in the kidney controls the synthesis of erythropoietin, the growth factor for the red blood cell series. (See Fig. 27-9 for the erythropoietin signaling pathway.) A dimeric transcription factor called HIF-1a/HIF-1b regulates the expression of erythropoietin. When oxygen is abundant, HIF-1a is hydroxylated on a proline residue, marking it for ubiquitination and destruction (see Fig. 23-8), turning down the expression of erythropoietin. When kidney cells lack oxygen (owing to low levels of red blood cells, poor blood circulation in the kidney, or high altitude) HIF-1a/HIF-1b accumulates, and erythropoietin is expressed and secreted to stimulate red blood cell production by bone marrow. The reciprocal relationship between oxygen and erythropoietin that is achieved by this feedback mechanism sets red blood cell production at a level required to deliver oxygen to the tissues. Many other cells use the HIF-1a/HIF-1b system to adjust gene expression to local oxygen levels.
Mutations altering the growth control (see Fig. 41-10) of a stem cell can give rise to monoclonal proliferative disorders, such as leukemia. In chronic myelogenous leukemia, a chromosomal rearrangement in a single white blood cell precursor creates a fusion between the genes for BCR (breakpoint cluster region) and ABL (a Src family cytoplasmic tyrosine kinase; see Fig. 25-3 and Box 27-1). The BCR-ABL protein is constitutively active and drives the proliferation of a clone of immature white blood cells that crowd out and inhibit the production of other blood cells, leading to anemia and platelet deficiency. Affected individuals are prone to infection because the immature white blood cells are ineffective phagocytes. Fortunately, a small-molecule inhibitor of the kinase activity of BCR-ABL suppresses this clone in many patients. Uncontrolled proliferation of a clone of red blood cell precursors causes a similar condition, characterized by excess red cells, called polycythe-mia vera.
Cells Confined to the Blood
Erythrocytes (Red Blood Cells)
Red blood cells (Fig. 28-5; also see Fig. 7-6) contain more than 300 mg/mL of hemoglobin to carry oxygen from the lungs to tissues and carbon dioxide from tissues to the lungs. These highly specialized cells discard their nuclei and organelles late in their development. A resilient, spectrin-actin membrane cytoskeleton (see Fig. 7-10) maintains the biconcave shape even after the cell is heavily distorted each time it passes through a small capillary. The elasticity of the membrane skeleton allows it to regain its shape. After circulating in blood for 120 days, erythrocytes abruptly become senescent, and phagocytes in the spleen, liver, and bone marrow remove them from the blood. The biochemical basis of this precise cellular aging and clearance process is still being investigated.
In hereditary spherocytosis (and other hemolytic anemias), the membrane cytoskeleton loses its resili-ency as a result of mutations, causing deficiencies or molecular defects of spectrin or other component proteins. These defective cells are easily damaged and eventually become smaller and rounder than normal. Many different mutations of the globin genes decrease the stability or oxygen-carrying capacity of hemoglobin. In sickle-cell disease, hemoglobin S is prone to assemble into tubular polymers that distort the cell and clog up the circulation.
Platelets
Platelets, small anucleate cellular fragments derived from megakaryocytes, contribute to both blood clotting and the repair of minor defects in the sheet of endothelial cells that lines blood vessels. A long, coiled microtubule presses out against the plasma membrane, like a spring, to maintain the platelet’s disk shape (Fig. 28-6). The most prominent organelles are two types of membrane-bound granules. Dense granules contain adenosine diphosphate (ADP) and serotonin. Alpha granules contain stores of adhesive glycoproteins including fibrinogen, fibronectin (see Fig. 29-15) and thrombospondin as well as the potent protein hormone called platelet-derived growth factor. Platelet-derived growth factor has a role in wound healing (see Fig. 32-11) but also contributes to atherosclerosis by stimulating the abnormal proliferation of smooth muscle cells in the walls of damaged arteries.
Platelets repair defects in the endothelial cell lining of blood vessels that are produced by the mild trauma of daily existence. Platelets bind to von Willebrand factor and collagen in the basal lamina when it is exposed by damage to the endothelium. This triggers one of the best-understood examples of regulated cellular adhesion (for more detail, see Fig. 30-14). Activated platelets aggregate, extend actin-containing filopodia, and secrete the contents of their granules. The secreted ADP sets up a positive feedback loop, activating more platelets that form a cluster to fill the defect in the endothelium. Patients with defective platelets or reduced circulating platelets (a complication of bone marrow disease and cancer chemotherapy) bruise easily, owing to unrepaired damage in small blood vessels, and may even bleed spontaneously. Conversely, hyperactive platelets may initiate pathological clots in the blood vessels of the heart, causing heart attacks or thrombosis in the veins of the legs.
Cells Responsible for Innate and Adaptive Immunity
All multicellular animals use two forms of innate immunity to defend themselves against infection by microorganisms. Phagocytic cells similar to unicellular amoebas track down, ingest, and kill bacteria and fungi (see Fig. 22-3). The main mammalian phagocytes are macrophages and neutrophils. A second line of defense is secretion of cytokines and small antimicrobial proteins by white blood cells and epithelial cells of the skin and intestine. These cells are alerted to the presence of microorganism by “pattern recognition receptors,” the best known of which are Toll-like receptors (see Fig. 24-12). These receptors bind macromolecular products that are essential for the microorganisms, such as bacterial flagella and viral single-stranded RNA. Toll-like receptors regulate gene expression through transcription factors, including NF-kB. Secreted tumor necrosis factor and other cytokines attract cells of the innate immune system (see Fig. 26-11). Antimicrobial peptides such as defensins and cathelicidins not only kill pathogens by interacting with their membranes but also attract and activate cells of both the innate and the adaptive immune systems. These elements of the innate immune system are programmed genetically, so they respond without prior exposure to the pathogen. In spite of their generic nature, these innate responses work remarkably well, defending all metazoans against infection. In addition to phagocytes, mammals have special lymphocytes called natural killer cells that express a variety of receptors to detect infected cells.
Cellular Basis of Innate Immunity
The phagocytes in the blood and tissues originate in bone marrow from a common committed stem cell (Fig. 28-5) and acquire unique functions as they differentiate. All of these motile cells are attracted to sites of infection or inflammation by (in humans) a family of about 40 small proteins called chemokines. Tissue cells and leukocytes secrete chemokines at sites of infection or inflammation. Chemokines with many unrelated names (IL-8, RANTES, eotaxin, MCP-1, etc.) have similar structures and bind to a family of 14 different chemokine receptors expressed selectively by lymphocytes, monocytes, and granulocytes. These seven-helix receptors are coupled to trimeric G-proteins (see Fig. 24-3) that mediate chemotaxis (see Fig. 38-12) toward the source of the chemokine.
Neutrophils
Neutrophils, also known as polymorphonuclear leukocytes or “polys,” are the main phagocytes circulating in blood on their way to connective tissues. They are distinguished by a multilobed nucleus and two types of granules (Fig. 28-7). The more abundant specific granules contain lysozyme (an enzyme that digests bacterial cell walls) and alkaline phosphatase. These granules do not stain with either the basic or acidic dyes used for blood smears, so these cells are called neutrophils. Azurophilic granules are true lysosomes containing hydrolytic enzymes bound to acidic proteoglycans (see Fig. 21-17). Neutrophils have copious glycogen but few mitochondria, so they rely on glycolysis for ATP synthesis in poorly oxygenated wounds. They are among the most motile cells in the body.
Human bone marrow produces about 80 g of neutrophils each day. In response to infection or injury, a circulating factor releases neutrophils from the bone marrow into the blood. Neutrophils spend about 10 hours in blood, alternating between a circulating pool and a so-called marginated pool adherent to endothelial cells, chiefly in the lung. Exercise and epinephrine release marginated neutrophils into the circulating pool; smoking increases the marginated pool. Neutrophils leave the blood by receptor-mediated attachment to endothelial cells and then crawl between endothelial cells into the connective tissue (see Fig. 30-13), where they perish after a day or two of phagocytosis.
Neutrophils are humans’ first line of defense against bacterial infection, as they are highly specialized for finding and destroying bacteria. Provided that the concentration of neutrophils is high enough (about 10 million cells per milliliter), these motile phagocytes can find and destroy bacteria faster than the invaders can reproduce. Bacterial products, especially N-formylated peptides, attract neutrophils by binding plasma membrane receptors and stimulating locomotion, similar to chemotaxis by other cells (see Fig. 38-12). Neutrophils bind and ingest bacteria by phagocytosis (see Fig. 22-3). Both types of granules fuse with phagosomes, delivering antibacterial proteins and proteolytic enzymes that kill the ingested bacteria. Some granules fuse with the plasma membrane, releasing antibacterial proteins outside the cell. Phagosome membranes produce millimolar concentrations of superoxide (O2−) radicals and other reactive oxygen species that help to disperse the granule enzymes and contribute to killing bacteria. These toxic oxygen species may also cause collateral damage to the neutrophil. Genetic defects in the enzymes that produce superoxide cause chronic granulomatous disease, a serious human disease, because neutrophils cannot kill ingested bacteria and fungi.
Monocytes and Macrophages
Monocytes in the blood are precursors of tissue macrophages. Monocytes are large cells with an indented nucleus and a small number of azurophilic granules (Figs. 28-5 and 28-7D). After circulating in the blood for about three days, monocytes enter tissues and differentiate into macrophages under the influence of local growth factors, including lymphokines secreted by lymphocytes (Fig. 28-8). They enlarge and amplify their machinery for locomotion, phagocytosis, and killing microorganisms and tumor cells.
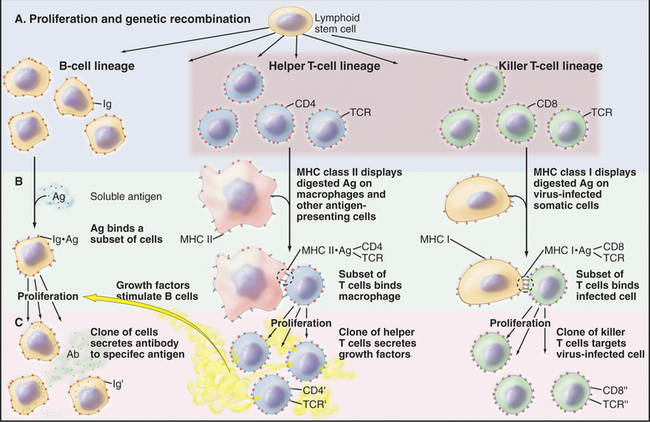
Figure 28-8 the immune response by three classes of lymphocytes through three parallel steps. A, Genetic recombination produces populations of cells with a wide variety of antigen specificities provided by cell surface immunoglobulins (Ig) or T-cell receptors (TCR). B, The binding of specific antigens (Ag) to surface immunoglobulins or T-cell receptors selects a subset of the cells. C, Proliferation of clones of the selected cells yields many cells specialized to produce antibody (Ab) to soluble antigens, secretion of growth factors by helper T cells in response to ingested and degraded antigens, or killing of virus-infected cells identifiable by the viral peptides on their surface. The helper and killer T cells use a common set of T-cell receptors and are guided to the appropriate target cells by the CD4 and CD8 accessory molecules. See Figures 27-8 and 46-8 for details on T-lymphocyte activation and selection, respectively.
Macrophages are professional phagocytes, which generally follow neutrophils to wounds or infections to clean up debris, including cellular debris and foreign material. Plasma membrane receptors for antibodies allow macrophages to recognize foreign matter marked with antibodies and to facilitate its ingestion. Primary lysosomes fuse with phagosomes to degrade the contents. Eventually, the cytoplasm fills with residual bodies containing the remains of ingested material (see Fig. 23-3). These “professional phagocytes” may divide and survive for months in tissues, where they ingest foreign material, participate in immune responses, and secrete growth factors that influence other cells. When confronted with large foreign bodies, macrophages can fuse together to form giant cells. No challenge is too great. Giant multinucleated microphages will even try to ingest a Petri dish if it is coated with antibody. Local growth factors in bone stimulate monocytes to fuse and differentiate into multinucleated osteoclasts that degrade bone matrix during bone remodeling (see Fig. 32-6).
Macrophages participate in the immune response by degrading ingested protein antigens and presenting fragments on their surface bound to MHC class II proteins (Fig. 28-8). This complex activates helper T lymphocytes carrying the appropriate T-cell receptors (see Fig. 27-8). Activated T cells proliferate and secrete growth factors that stimulate B lymphocytes to produce antibody. Macrophages also secrete a variety of factors involved with host defense and inflammation. Interleukin-1, transforming growth factor-a, transforming growth factor-b, and platelet-derived growth factor stimulate the proliferation and differentiation of the cells required to heal wounds (see Fig. 32-11). Chemokines attract cells of the immune system to sites of inflammation.
Eosinophils
Eosinophils are identified in blood smears as cells with a bilobed nucleus and large specific granules that stain brightly with eosin (Fig. 28-7B). Specific granules contain a cationic protein, a ribonuclease and peroxidase, in addition to a crystalloid of a basic protein. Like neutrophils, eosinophils pass briefly through the blood on their way to connective tissue, where they survive for about two weeks. Chemotactic factors generated by the complement system, basophils, some tumors, parasites, and bacteria all attract eosinophils. Many of the same factors attract other leukocytes, but particular chemokines are specialized for eosinophils. Eosinophils accumulate in blood and tissues in response to parasitic infections. Eosinophils bind parasites and lyse them, like killer lymphocytes (see later discussion), by secreting a cationic protein that forms pores in their membrane. Production of superoxide, hydrogen peroxide, and antimicrobial peptides also contributes to killing. Activated eosinophils contribute to inflammation in some allergic disorders such as asthma.
Basophils
Basophils are the least abundant and least understood granulocytes. They look much like neutrophils but have a bilobed nucleus and large, basophilic, specific granules containing heparin, serotonin, and all of the blood histamine (Fig. 28-7C). Basophils are weak phagocytes. Like mast cells, they have cell surface receptors that bind IgE and release the vasoactive agents stored in their granules when antigens bind to these bound immunoglobulins.
Cellular Basis of Adaptive Immunity
In response to infection, lymphocytes of the immune systems of vertebrates produce two kinds of adaptive responses: humoral (in the body fluids) and cellular. B lymphocytes produce the humoral response by secreting antibodies (immunoglobulins), soluble proteins that diffuse in the blood and tissue fluids. Two types of T lymphocytes mediate the cellular arm of the adaptive immune response. Cytotoxic T lymphocytes (killer T cells) destroy cells infected with viruses, whereas helper T cells regulate other lymphocytes. These responses protect against infection but fail in acquired immunodeficiency syndrome (AIDS) when the human immunodeficiency virus (HIV) kills helper T cells. A blood smear reveals lymphocytes of various sizes and shapes (Fig. 28-5) but not their remarkable heterogeneity at the molecular level (Fig. 28-8).
The huge repertoire of antigen-binding sites present in the collection of antibodies that circulate in a single individual arises through rearrangement and somatic mutations of immunoglobulin genes (Fig. 28-9). This remarkable process was exploited during evolution specifically for the use of the immune system. Each mammalian immunoglobulin is composed of four polypeptide chains—two identical heavy chains and two identical light chains—each encoded by different genes (see Fig. 3-13). Light chains and heavy chains both contribute to the antigen-binding site. In vertebrate genomes, immunoglobulin genes exist in segments aligned along a chromosome. Several of these gene segments must be combined in the proper order to make a full-length functional antibody gene. Some of these gene segments encode the framework of the antibody protein, which is essentially identical within each class of antibodies. Other gene segments, present in many variations, encode the part of the polypeptide chain that forms the antigen-binding site.
Infection by a pathogen results in the production of antibodies that bind to the pathogen but not to any of the individual’s own molecules. This response comes from activation and proliferation of preexisting B cells that have the capacity to make antibodies to molecules of the pathogen. Activation requires a chance encounter of particular B cells with the pathogen and stimulates the cell to mature into a factory for secreting antibodies. Alternate splicing of messenger RNA (mRNA [see Fig. 16-6]) selects domains required to direct the same antibody to either the plasma membrane or the secretory pathway.
Cells that are activated by antigen binding to a surface immunoglobulin divide to increase their numbers. This process, called clonal expansion, amplifies the production of antibody specific for the antigen. The mature cellular product of the B-cell response is a plasma cell, which is highly specialized to secrete one specific antibody. Other B cells that are activated by the antigen become memory cells. These long-lived cells display the specific antibody on their surface and stand poised to mount an amplified response on subsequent exposure to the same antigen. This immunologic memory explains why exposure to a particular pathogen or vaccination against a pathogen results in protection, in the form of antibodies, for many years.
Specialized B lymphocytes and plasma cells secrete different antibody isoforms or isotypes. Formation of immunoglobulins with the various isotypes requires further recombination events to join the variable region with the antigen-binding site to the isotype constant domain. IgG isotypes, produced in lymph nodes and spleen, circulate in blood and tissue fluids. IgA isotypes, produced by lymphoid nodules in the respiratory and gastrointestinal tracts and by mammary glands, are first taken up and then secreted by epithelial cells of these organs (transcytosis [see Fig. 22-6]). IgE isotypes bind to receptors on the surface of mast cells and basophils (see earlier discussion).
T lymphocytes provide cellular responses to pathogens. Cytotoxic T cells execute tumor cells and virus-infected cells. Helper T cells stimulate antibody production by B cells. The specificity of these responses is provided by variable cell surface receptors called T-cell receptors (see Fig. 27-8). A set of segmented genes analogous to immunoglobulin genes encode T-cell receptors. In contrast to antibodies, T-cell receptors do not bind free antigens but rather recognize peptide antigens displayed on the surface of target cells complexed to proteins called major histocompatibility complex (MHC) antigens (see Fig. 27-8). These highly variable MHC proteins are responsible for the rejection of tissue grafts from nonidentical individuals.
The two types of MHC proteins—class I and class II—acquire their antigenic peptides differently. All somatic cells produce class I MHC proteins. In cells that have been infected by a virus, cytoplasmic immunoproteasomes degrade some viral proteins to peptides (see Chapter 23), which ABC transporters (TAP1, 2) move from the cytoplasm (see Fig. 8-9) into the endoplasmic reticulum. In the lumen of the ER, peptides insert into the binding site of compatible class I molecules and the complex moves to the plasma membrane. In contrast, macrophages and other antigen-presenting cells, such as dendritic cells, ingest foreign matter and degrade it in endosomes and lysosomes. Peptide fragments bind to class II proteins in endosomes and thence move to the cell surface of these antigen-presenting cells.
T lymphocytes patrol the body, inspecting the surfaces of other cells. A chance encounter with a cell displaying a peptide-MHC complex complementary to its T-cell receptor stimulates the T cell (see Fig. 27-8). The response is proliferation and expansion of a clone of identical T cells. Accessory membrane proteins CD4 and CD8 on the T-cell surface cooperate with T-cell receptors to direct the two types of T cells to target cells with the appropriate MHC proteins. T-cell receptors provide antigen specificity. Immature T-cells express both CD4 and CD8 but lose one of them as they mature into cytotoxic (CD8+) or helper (CD4+) T cells.
CD8-positive cytotoxic T cells are specialized to kill cells infected with viruses. The presence of virus inside is revealed by MHC class I proteins displaying vital peptides on the surface of the infected cell. CD8 binds to a constant region of MHC class I proteins carrying viral peptides. During its intimate encounters with the target cell, a cytotoxic T cell uses three weapons to kill the target: First, T cells carry a ligand for the Fas receptor on the target, which stimulates apoptosis of the target cell (see Fig. 46-17). Second, activated T cells secrete perforin, a protein that inserts into the plasma membrane of the target cell, forming large (10 nm) pores that leak cytoplasmic contents and ultimately lyse the cell. Third, T cells secrete toxic enzymes that enter target cells through the plasma membrane pores.
ACKNOWLEDGMENTS
Thanks go to John Hartwig and Sam Silverstein for their suggestions on revisions to this chapter.
Beutler B. Inferences, questions and possibilities in Toll-like receptor signalling. Nature. 2004;430:257-263.
Boes M, Ploegh HL. Translating cell biology in vitro into immunity in vivo. Nature. 2004;430:264-270.
Buckley RH. Primary immunodeficiency diseases due to defects in lymphocytes. New Engl J Med. 2000;343:1313-1324.
Call ME, Wucherpfennig KW. The T cell receptor: Critical role of the membrane environment in receptor assembly and function. Annu Rev Immunol. 2005;23:101-125.
Cannon B, Nedergaard J. Brown adipose tissue: Function and physiological significance. Physiol Rev. 2004;84:277-359.
Delves PJ, Roitt IM. The immune system. New Engl J Med. 2000;343:37-49. 108–117
Galli SJ, Kalesnikoff J, Grimbaldeston MA, et al. Mast cells as “tunable” effector and immunoregulatory cells. Annu Rev Immunol. 2005;23:749-786.
Garg A. Acquired and inherited lipodystrophies. New Engl J Med. 2004;350:1120-1134.
Grinnell F. Fibroblast biology in three-dimensional collagen matrices. Trends Cell Biol. 2003;13:264-269.
Hargreaves DC, Medzhitov R. Innate sensors of microbial infection. J Clin Immunol. 2005;25:503-510.
Hartwig J, Italiano JJr. The birth of the platelet. J Thromb Haemost. 2003;1:1580-1586.
Janeway CAJr., Medzhitov R. Innate immune recognition. Annu Rev Immunol. 2002;20:197-216.
Kaufmann SHE. Cell-mediated immunity: Dealing a blow to pathogens. Curr Biol. 1999;9:R97-R99.
Kaushansky K. Thrombopoietin. N Engl J Med. 1998;339:746-754.
Klein J, Sato A. The HLA system. New Engl J Med. 2000;343:702-709. 782–786
Lanzavecchia A, Sallusto F. Dynamics of T lymphocyte responses: Intermediates, effectors and memory cells. Science. 2000;290:92-97.
Lekstrom-Himes JA, Gallin JI. Immunodeficiency diseases caused by defects in phagocytes. New Engl J Med. 2000;343:1703-1714.
Marx J. How cells endure low oxygen. Science. 2004;303:1454-1456.
Rot A, von Adrian UH. Chemokines in innate and adaptive host defense: Basic chemokinese grammar for immune cells. Annu Rev Immunol. 2004;22:891-928.
Segal AW. How neutrophils kill microbes. Annu Rev Immunol. 2005;23:197-223.
Takeda K, Kaisho T, Akira S. Toll-like receptors. Annu Rev Immunol. 2003;21:335-376.
Trombetta ES, Mellman I. Cell biology of antigen processing in vitro and in vivo. Annu Rev Immunol. 2005;23:975-1028.
van Andrian UH, Mackay CR. T-cell function and migration. New Engl J Med. 2000;343:1020-1034.
Verfaillie CM. Adult stem cells: Assessing the case for pluripotency. Trends Cell Biol. 2002;12:502-508.
Yang D, Biragyn A, Hoover DM, et al. Multiple roles of antimicrobial defensins, cathelicidins, and eosinophil-derived neurotoxin in host defense. Annu Rev Immunol. 2004;22:181-215.
Yokoyama WM, Kim S, French AR. The dynamic life of natural killer cells. Annu Rev Immunol. 2004;22:405-429.