Chapter 9 Cell Cooperation in the Antibody Response
• The primary development of B cells is antigen independent. Pre-B cells recombine genes for immunoglobulin heavy and light chains to generate their surface receptor for antigen.
• T-independent antigens activate B cells without requiring T-cell help. They can be divided into two groups. TI-1 antigens can act as polyclonal stimulators, while TI-2 antigens are polymers which activate by cross-linking the B-cell receptor.
• T-dependent antigens are taken up by B cells, processed and presented to TH cells. T cells and B cells usually recognize different parts of an antigen.
• B-cell activation requires signals from the B-cell receptor and costimulation. CD40 is the most important costimulatory molecule on B cells. Ligation of the B-cell–coreceptor complex can lower the threshold of antigen needed to trigger the B cell. Intracellular signaling pathways are analogous in B cells and T cells.
• Activated B cells proliferate and differentiate into antibody-forming cells. Cytokines from TH cells control the process of division, differentiation and class switching.
• Somatic hypermutation of immunoglobulin genes, followed by selection of high-affinity clones is the basis of affinity maturation. These processes occur in germinal centers.
• Class switching is effected by somatic recombination occurring within the heavy chain genes. Somatic hypermutation and class-switching by recombination are linked processes, which require selective targeting of DNA-modification and DNA-repair enzymes to the heavy chain gene locus.
Development of B cells
Primary B-cell development is antigen independent
Within the bone marrow, a sequence of immunoglobulin rearrangements and phenotypic changes takes place during B-cell ontogeny, analogous to that described for T cells in the thymus which leads to the production of the B cell’s antigen receptor (Fig. 9.w1). The molecular processes involved in immunoglobulin gene rearrangement have been described in Chapter 3, and this section relates these events to B-cell development.
• terminal deoxynucleotidyltransferase (TdT), an intranuclear enzyme uniquely expressed during Vh-gene rearrangement; and
• B220, which identifies a particular splice-variant of leukocyte common antigen (CD45R in humans), a tyrosine phosphatase that appears to be important in regulating B-cell receptor signaling.
Genes for immunoglobulin H and L chains are recombined to generate the surface receptor
Pre-B cells can be divided into large mitotically active pre-B cells and small non-dividing pre-B cells. Both large and small pre-B cells express Igμ heavy chains in the cytoplasm (cμ) and the pre-B cell–receptor complex on their surface (Fig. 9.w2). Large pre-B cells have successfully rearranged their Ig heavy chain genes. As these cells pass from the large pre-B cell group into the small pre-B cell group, they begin to rearrange their Ig light chain genes and upregulate RAG-1 and RAG-2. The final stage of B-cell development is the immature B-cell stage. Immature B cells have successfully rearranged their light chain genes and express IgM. Once again, RAG-1 and RAG-2 expression has been downregulated. As immature B cells develop further into mature B cells, they begin to express both IgM and IgD on their surface. These mature B cells are then free to exit the bone marrow and migrate into the periphery.
Other phenotypic markers such as CD25 (IL-2Rα chain), CD79a (Igα), CD79b (Igβ), and c-Kit can help to identify particular populations of pro-B, pre-B or immature B cells (see Fig. 9.w1).
Transcriptional controls in B-cell development
B-lymphopoiesis is regulated by multiple transcription factors including:
• E2A and EBF, which coordinately activate early B-cell genes;
• Pax5, which ensures development to B-cell lineages by restricting transcription of lineage inappropriate genes and activating expression of B-lineage signaling molecules;
• Sox4 and LEF1, which promote the survival and proliferation of pro-B cells;
• IRF4 and IRF8, which terminate pre-BCR signaling by IRF4 and promote differentiation to small pre-B cells; and
• Bcl-6 expression, which is required for germinal B-cell differentiation and generation of memory B-cells.
B cell activation
T-independent antigens do not require T cell help to stimulate B cells
A small number of antigens, however, can activate B cells without MHC class II-restricted T cell help and are referred to as T-independent (TI) antigens (Fig. 9.1).
• TI-1 antigens are predominantly bacterial cell wall components – the prototypical TI-1 antigen is lipopolysaccharide (LPS), a component of the cell wall of Gram-negative bacteria;
• TI-2 antigens are predominantly large polysaccharide molecules with repeating antigenic determinants (e.g. Ficoll, dextran, polymeric bacterial flagellin, and poliomyelitis virus).
T-independent antigens induce poor memory
Primary antibody responses to TI antigens in vitro are generally slightly weaker than those to TD antigens. They peak fractionally earlier and both generate mainly IgM. However, the secondary responses to TD and TI antigens differ greatly. The secondary response to TI antigens resembles the primary response, whereas the secondary response to TD antigens is far stronger and has a large IgG component (Fig. 9.2). It seems, therefore, that TI antigens do not usually induce the maturation of a response leading to class switching or to an increase in antibody affinity, as seen with TD antigens. This is most likely due to the lack of CD40 activation (see below). Memory induction to TI antigens is also relatively poor.
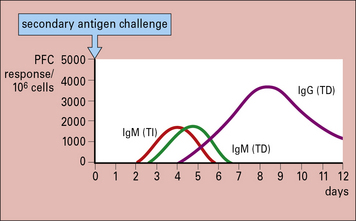
Fig. 9.2 Comparison of the secondary immune response to T-dependent and T-independent antigens in vitro
The secondary response to T-dependent antigens is stronger and induces a greater number of IgG-producing cells, as measured by plaque-forming cells (see Method box 9.1).
It is possible to convert TI antigens into T-dependent antigens by altering their structure. For example, pneumococcal polysaccharides are TI antigens and do not induce immunological memory or antibodies in infants. However, conjugation of pneumococcal polysaccharides to a carrier protein induces polysaccharide-specific antibody in infants, and memory similar to T-dependent antigens (see Method boxes 9.1 and 2.1).
Method box 9.1 Measuring antibody production – the PFC assay and ELISPOT
Antibody-forming cells (AFCs) are measured by means of a quantitative PFC assay (Fig. MB9.1.5), originally developed by Niels Jerne in the 1960s. B cells (e.g. spleen cells) are plated in agar with sheep red blood cells sensitized by binding the specific antigen to their surface. Antibody produced by any B cell will coat the red blood cells. Following the addition of complement, these coated cells may be lyzed, causing the appearance of a zone of lyzed cells (plaque) in the agar. Figure MB9.1.5 shows the appearance of such a plaque, with a B cell in the center, under the microscope. The plaques are then counted to give a quantitative measure of the number of PFCs.
Another way of detecting antibody-producing cells is by means of an enzyme-linked immunospot assay (ELISPOT). An ELISPOT assay starts out by coating a plastic well with antigen and adding a known quantity of B cells. The antigen coated onto the plastic will then capture any antibody in the vicinity of the activated B cell that is producing the antibody. After a period of time, the B cells are removed, and the specific antibody can be detected by adding an enzyme-labeled anti-immunoglobulin plus chromogen. Development of the label in this assay results in a spot surrounding the active B cell. Counting each spot and knowing the quantity of B cells originally added to the well allows one to enumerate the frequency of B cells producing the specific antibody. Method Box 2.1, Fig. MB2.1.3 shows the method of detecting antibodies and the appearance of the spots on the developed plates. In addition to analyzing specific antibody-secreting B cells, the ELISPOT assay has been adapted to measure the frequency of cytokine-secreting T cells and various other cell types (right panel). With the improvement in ELISPOT assay plate design and in ELISPOT detection equipment, antibody- or cytokine-secreting cells can now be detected at the single cell level.
Activation of B cells by T-dependent antigens
T cells and B cells recognize different parts of antigens
By manipulating the cell populations in these experiments, it was shown that:
These experiments were later reinforced by details of how:
This has led to the basic scheme for cell interactions in the antibody response set out in Figure 9.3. It is proposed that antigen entering the body is processed by cells that present the antigen in a highly immunogenic form to the TH and B cells. The T cells recognize determinants on the antigen that are distinct from those recognized by the B cells, which differentiate and divide into antibody-forming cells (AFCs). Therefore two processes are required to activate a B cell:
• antigen interacting with B cell immunoglobulin receptors – this involves ‘native’ antigen;
• stimulating signal(s) from TH cells that respond to processed antigen bound to MHC class II molecules.
B-cell activation and T-cell activation follow similar patterns
Cross-linking of surface Ig leads to activation of the src family kinases, which in B cells are Fyn, Lyn, and Blk. Syk is analogous to ZAP-70 in T cells, and binds to the phosphorylated ITAMs of Igα and Igβ (Fig. 9.4). This leads to activation of a kinase cascade and translocation of nuclear transcription factors analogous to the process that occurs in T cells.
B-cell activation is also markedly augmented by the ‘co-receptor complex’ comprising three proteins:
• CD21 (complement receptor-2, CR2);
• CD81 (target of anti-proliferative antibody, TAPA-1) (Fig. 9.5).
Q. If an animal was depleted of complement C3 during the primary immune response, what effect do you think this would have on the development of the secondary antibody response?
A. Lack of C3 means that immune complexes containing C3b and C3d do not form. Therefore they cannot bind to follicular dendritic cells (FDCs) via CR2 or engage with B cells via the B cell co-receptor complex (see Fig. 9.5); consequently B cell activation and the development of the secondary immune response is greatly impaired. (These experiments have been done by depleting mice of C3 using cobra venom factor.)
Direct interaction of B cells and T cells involves costimulatory molecules
• the T cells become polarized, with the T-cell receptors concentrated on the B-cell side;
• the B cells also become polarized and express most of their MHC class II molecules and ICAM-1 in proximity to the T cells.
The initial interaction between a naive B cell and a cognate antigen via the BCR in the presence of cytokines or other growth stimuli induces activation and proliferation of the B cell. This then leads to processing of the T-dependent antigen and presentation to T cells. The interaction between B cells and T cells is a two-way process in which B cells present antigen to T cells and receive signals from the T cells for division and differentiation (Fig. 9.6).
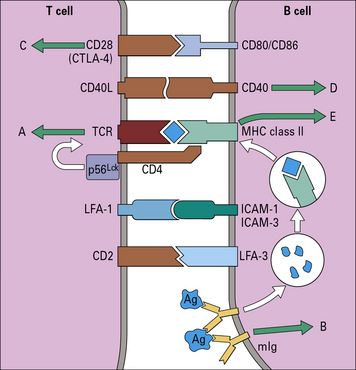
Fig. 9.6 Cell surface molecules involved in the interaction between B and TH cells
(Adapted with permission from DeFranco A, Nature 1991; 351: 603–5.)
The interaction between B and T cells is a two-way event as follows:
• CD40, a member of the TNF receptor family, delivers a strong activating signal to B cells, more potent even than signals transmitted via surface immunoglobulin;
• upon activation, T cells transiently express a ligand, termed CD40L (a member of the TNF family), which interacts with CD40;
• CD40–CD40L interaction helps to drive B cells into cell cycle;
• transduction of signals through CD40 induces upregulation of CD80/CD86 and therefore helps to provide further costimulatory signals to the responding T cells.
Cytokine secretion is important in B-cell proliferation and differentiation
Recent work has shown that CD4+ T cells in both mouse and man can be divided into different subsets, depending on their cytokine profile (see Fig. 11.4). During B-cell–T-cell interaction, T cells can secrete a number of cytokines that have a powerful effect on B cells (Fig. 9.7). IL-2, for example, is an inducer of proliferation for B cells as well as T cells.
• IL-4 acts on B cells to induce activation and differentiation. It also acts on T cells as a growth factor and promotes differentiation of TH2 cells, thus reinforcing the antibody response; excess IL-4 plays a part in allergic disease, causing production of IgE;
• IL-5 in humans is chiefly a growth and activation factor for eosinophils and is responsible for the eosinophilia of parasitic disease. In the mouse it also acts on B cells to induce growth and differentiation;
• IL-6 is produced by many cells including T cells, macrophages, B cells, fibroblasts and endothelial cells and acts on many cell types, but is particularly important in inducing B cells to differentiate into AFCs. IL-6 is considered to be an important growth factor for multiple myeloma, a malignancy of plasma cells;
• IL-10 acts as a growth and differentiation factor for B cells in addition to modulating cytokine production by TH1 cells;
• IL-13, which shares a receptor component and signaling pathways with IL-4, acts on B cells to produce IgE.
Cytokines can also influence antibody affinity. Antibody affinity to most TD antigens increases during an immune response, and a similar effect can be produced by certain immunization protocols. For example, high-affinity antibody subpopulations are potentiated after immunization with antigen and IFNγ (Fig. 9.8). A number of adjuvants are capable of enhancing levels of antibody, but few also potentiate affinity. As affinity markedly influences the biological effectiveness of antibodies, IFNγ may be an important adjuvant for use in vaccines.
Cytokine receptors guide B-cell growth and differentiation
Receptors for the many growth and differentiation factors required to drive the B cells through early stages of development are expressed at various stages of B-cell differentiation. Receptors for IL-7, IL-3 and low-molecular-weight B-cell growth factor are important in the initial stages of B-cell differentiation, whereas other receptors are more important in the later stages (Fig. 9.9).
B cell differentiation and the antibody response
• the first pathway involves proliferation and differentiation into antibody forming cells (AFCs) in the lymph nodes or in the periarteriolar lymphoid sheath of the spleen. These AFCs function to rapidly clear antigen. However, the great majority of these cells die via apoptosis within 2 weeks. Therefore, it is unlikely that these AFCs are responsible for long-term antibody production;
• in the second pathway some members of the expanded B-cell population migrate into adjacent follicles to form germinal centers before differentiating into memory B cells.
B cell affinity maturation occurs in germinal centers
The germinal center is important in that it provides a microenvironment where B cells can undergo developmental events that ultimately result in an affinity-matured, long-lived memory B-cell compartment (Fig. 9.10). These developmental events come about due to complex interactions between B cells, CD4+ TH cells, and follicular dendritic cells. These events include:
The germinal center initially contains only dividing centroblasts. Shortly thereafter, it polarizes into a dark zone containing centroblasts and a light zone containing non-dividing (resting) centrocytes (Figs 9.11 & 9.12). Centroblasts proliferate rapidly in the dark zone and downregulate the expression of their surface immunoglobulin. Somatic hypermutation then occurs to diversify the rearranged variable region genes (see Chapter 3, Fig. 3.28). Somatic hypermutation allows a single B cell to give rise to variants with different affinities for the antigen.
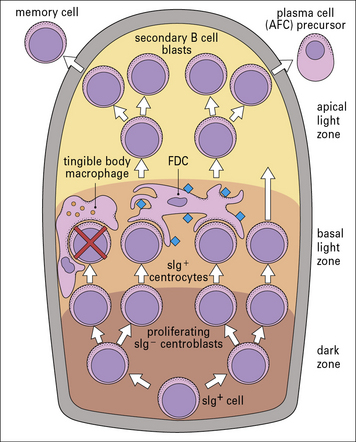
Fig. 9.11 Schematic organization of the germinal center
(Adapted from Roitt IM. Essential Immunology, 7th edn. Oxford: Blackwell Scientific Press, 1991.)
Self-reactive B cells generated by somatic mutation are deleted
The factors that regulate the decision to exit the germinal center are poorly understood.
In-vivo antibody responses show isotype switching, affinity maturation and memory
The earliest studies on antibody responses in vivo identified different phases in the response. Following primary antigenic challenge, there is an initial lag phase when no antibody can be detected. This is followed by phases in which the antibody titer increases logarithmically to a plateau and then declines. The decline occurs because the antibodies are either naturally catabolized or bind to the antigen and are cleared from the circulation (Fig. 9.13).
The responses following primary and secondary antigenic challenge are shown in Figure 9.14 and they differ in four major respects.
Affinity maturation depends on cell selection
The degree of affinity maturation is inversely related to the dose of antigen administered. High antigen doses produce poor maturation compared with low antigen doses (Fig. 9.15). It is thought that:
• in the presence of low antigen concentrations, only B cells with high-affinity receptors bind sufficient antigen and are triggered to divide and differentiate;
• in the presence of high antigen concentrations, there is sufficient antigen to bind and trigger both high- and low-affinity B cells.
B cell isotype switching and somatic hypermutation
Somatic hypermutation is a common event in antibody-forming cells during T-dependent responses and is important in the generation of high-affinity antibodies. Somatic hypermutation introduces point mutations at a very high rate into the variable regions of the rearranged heavy and light chain genes (see Fig. 3.28). This results in mutated immunoglobulin molecules on the surface of the B cell. Mutants that bind antigen with higher affinity than the original surface immunoglobulin provide the raw material for the selection processes mentioned above.
B cells recombine their heavy chain genes to switch immunoglobulin isotype
B cells produce antibodies of five major classes – IgM, IgD, IgG, IgA, and IgE. In humans, there are also four subclasses of IgG and two of IgA (see Chapter 3). Each terminally differentiated plasma cell is derived from a specific B cell and produces antibodies of just one class or subclass (isotype).
The arrangement of the constant genes in mouse and humans is shown in Figures 9.16 and 9.17. Upstream of the μ genes is a switch sequence (S), which is repeated upstream to each of the other constant region genes except δ. These sequences are important in the recombination events that occur during class switching, as explained below.
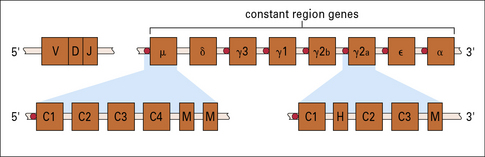
Fig. 9.16 Constant-region genes of mouse
The constant-region genes of the mouse are arranged 8.5 kb downstream from the recombined VDJ segment. Each C gene (except Cδ) has one or more switching sequences at its start (red circles), which correspond to a sequence at the 5′ end of the μ gene. This allows any of the C genes to be expressed together with the VDJ segment. δ genes appear to use the same switching sequences as μ, but the μ gene transcript is lost in RNA processing to produce IgD (see Fig. 9.19). The C genes (expanded below for μ and γ2a) contain introns separating the exons for each domain (C1, C2, etc.). The γ genes also have a separate exon coding for the hinge (H), and all the genes have one or more exons coding for membrane-bound immunoglobulin (M).
Class switching occurs during maturation and proliferation
Most class switching occurs during proliferation. However, it can also take place before encounter with exogenous antigen during early clonal expansion and maturation of the B cells (Fig. 9.18). This is known because some of the progeny of immature B cells synthesize antibodies of other immunoglobulin classes, including IgG and IgA.
Class switching may be achieved by differential splicing of mRNA
It is suggested that much larger stretches of DNA are sometimes also transcribed together, with differential splicing giving other immunoglobulin classes sharing Vh regions (Fig. 9.19). This has been observed in cells simultaneously producing IgM and IgE.
Class switching is mostly achieved by gene recombination
The principal mechanism of class switching is by recombination. B cells switch from IgM to the other immunoglobulin classes or subclasses by an intrachromosomal deletion process which involves the excision of intervening genetic material between highly repetitive switch regions 5′ to each chain (Fig. 9.20).
Membrane and secreted immunoglobulins are produced by differential splicing of RNA transcripts of heavy chain genes
Membrane immunoglobulins are therefore slightly larger than their secreted counterparts. Their additional amino acids traverse the cell membrane and anchor the molecule in the lipid bilayer. In membrane IgM, for example, a section of hydrophobic (lipophilic) amino acids is sandwiched between hydrophilic amino acids, which lie on either side of the membrane (Fig. 9.w3).
• exist only as the basic four-chain unit;
• do not polymerize further; and
• are associated with molecules involved in signal transduction (Igα and Igβ).
Production of the two forms of immunoglobulin (membrane and secreted) occurs by differential transcription of the germline C region (Fig. 9.w4). It is thought that the poly A sequence is important in determining which RNA transcript is produced, but exactly how this is controlled is uncertain.
Immunoglobulin class expression is influenced by cytokines and type of antigenic stimulus
• in mice, T cells in mucosal sites have been shown to stimulate IgA production;
• IL-4 preferentially switches B cells that have been either polyclonally activated (by lipopolysaccharide) or specifically activated by antigen to the IgG1 or IgE isotype, with concomitant suppression of other isotypes, and in a similar system IL-5 induces a 5–10-fold increase in IgA production with no change in other isotypes;
• IFNγ enhances IgG2a responses in mice, but suppresses all other isotypes (Fig. 9.21).
Critical thinking: Development of the antibody response (see p. 436 for explanations)
A project is underway to develop a vaccine against mouse hepatitis virus, a pathogen of mice, which may become a serious problem in colonies of mice. The vaccine consists of capsid protein of the virus, which is injected subcutaneously as a depot in alum on day 0. At days 5 and 14, the group of six mice is bled and the serum is tested for the presence of antibodies against the viral capsid protein. Separate assays are done for each of the immunoglobulin classes, IgM, IgG and IgA. The amounts, expressed in μg/mL of antibody, are shown in Figure 9.22.
1. Why do the titres of IgG antibodies increase more rapidly between days 5 and 14 than do IgM antibodies, in all animals?
2. Propose an explanation for the high titres of IgG antibodies in the two animals indicated at day 5. Can this explanation also account for the relatively high levels of IgA antibodies also seen in these mice?
3. Why do you suppose there are no IgA-producing clones, despite the good IgA response?
4. You want a high-affinity antibody for use in an assay. Which of the clones you have produced are likely to be of higher affinity?
Hardy R.R., Hayakawa K. B cell development pathways. Annu Rev Immunol. 2001;19:595–621.
Peled J.U., Kuang F.L., Iglesias-Ussel M.D., et al. The biochemistry of somatic hypermutation. Ann Rev Immunol. 2008;26:481–511.
Smith K.G., Fearon D.T. Receptor modulators of B cell receptor signaling – CD19/CD22. Curr Top Microbiol Immunol. 2000;245:195–212.
Stavenezer J., Guikema J.E.J., Schrader C.E. Mechanisms and regulation of class switch recombination. Ann Rev Immunol. 2008;26:261–292.