Carbon dioxide retention and capnography
The monitoring of CO2, the most abundant gas produced by the human body during anesthesia, has become a standard of practice strongly encouraged by the American Society of Anesthesiologists. CO2 is a byproduct of cellular metabolism, transported to the lungs by the systemic venous system and eliminated from the alveoli during ventilation (see Chapter 22).
CO2 retention
When Mapleson systems are used, inadequate fresh gas flow is the primary cause of an increase in CO2 because these systems do not contain unidirectional valves or absorbent canisters. Specifically, systems with inner tubes, such as the Bain system, can cause rebreathing if there is any dysfunction (kink) in that tube. The Mapelson D (Bain circuit) is the most efficient for controlled ventilation with regard to a relatively low flow of fresh gas, whereas the Mapleson A is most suitable for patients who are spontaneously breathing (see Chapter 193). Specific minimum fresh gas flow rates for the various Mapleson apparatuses are recommended for spontaneous ventilation as well as controlled ventilation (Box 9-1).
Capnograms
Capnograms rely on time or volume to assess CO2 concentrations. Time capnograms are further divided into slow and fast tracings. All have their advantages, but the time capnogram is the most commonly used system (fast speed for trends and slow speed for detailed waveform analysis). Volume capnograms are unique in that a breath-by-breath measurement of CO2 concentration can be made, dead space can be divided into components, and significant changes in the morphology of the expired waveform can be detected as they relate to ventilation and perfusion. Normal CO2 waveforms and several abnormal capnograms are shown in Figures 9-1 and 9-2. PaCO2 and PETCO2 trends of metabolism, circulation, ventilation, and various equipment failures are summarized in Table 9-1. Changes in PETCO2 and the capnogram may indicate one of a number of potential changes in the patient’s condition, requiring the clinician to act appropriately.
Table 9-1
Causes of Altered End-Tidal CO2 during Anesthesia*
Cause | PETCO2 | PETCO2-to-PaCO2 Gradient |
CO2 insufflation | Increased | Normal |
Increased CO2 production† | Increased | Normal |
Right-to-left shunt | Increased | Widened |
Increased physiologic or anatomic dead space, or both | Decreased | Widened |
Increased apparatus dead space | Increased | Normal |
Hyperventilation | Decreased | Normal |
Hypoventilation | Increased | Normal |
Leak in sampling line | Decreased | Widened |
Poor seal around tracheal tube | Decreased | Widened |
High sampling rate | Decreased | Widened |
Low sampling rate | Decreased | Widened |
Rebreathing due to malfunctioning breathing valve | Increased | Decreased |
Rebreathing with low fresh gas in the Mapleson system | Increased | Decreased |
Rebreathing with circle system (absorbent problem) | Increased | Normal |
*Normal pressure of end-tidal CO2 (PETCO2) is 38 mm Hg (5%). The PaCO2-to-PETCO2 gradient is normally <5 mm Hg.
†From hyperthermia, malignant hyperthermia, convulsions, pain, or bicarbonate administration.
Adapted, with permission, from Dorsch JA, Dorsch SE. Gas monitoring. In: Dorsch JA, Dorsch SE, eds. Understanding Anesthesia Equipment, 5th ed. Philadelphia: Lippincott, Williams & Wilkins; 2007:706-707.
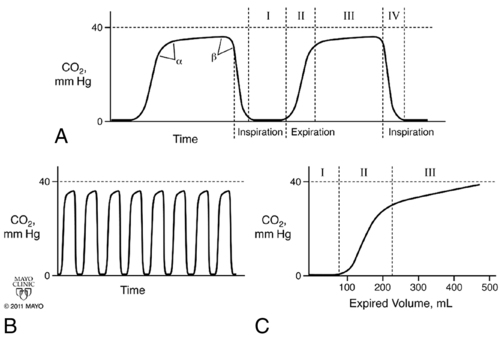
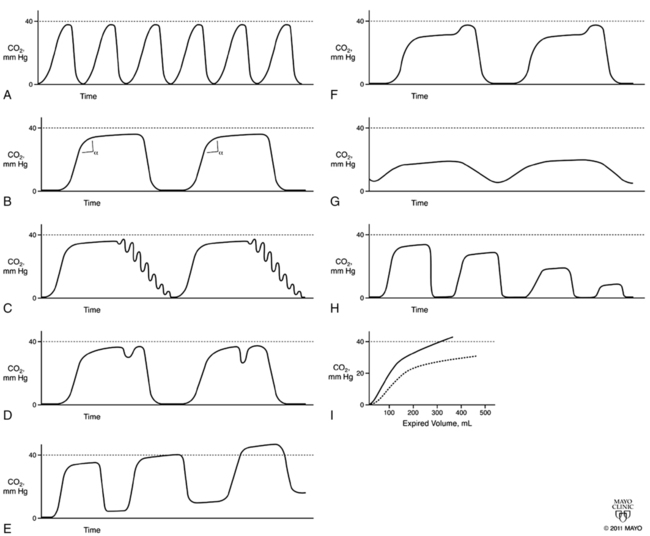