72 Cancer Stem Cell Biology and Its Role in Radiotherapy
Radiotherapy is one of the most frequently employed and most effective forms of cancer treatment. Advances in medical physics are leading to an ever-increasing ability to deliver conformal radiotherapy, thus allowing dose escalation to tumors while minimizing toxicity to surrounding normal tissues. Radiation therapy can be employed alone or in combination with surgery and chemotherapy, and plays a critical role in achieving local control and cure for many patients. However, even with modern treatment approaches, local control cannot always be attained. In these cases, toxicity to normal structures in the vicinity of a tumor limit the total dose that can be delivered, and thus force radiation oncologists to limit treatment to doses that are insufficient to eliminate every clonogenic cancer cell. In such cases, combined chemoradiotherapy can be a potentially effective therapeutic strategy. Unfortunately, the generally relatively limited levels of tumor cell killing achievable with cytotoxic chemotherapeutics (compared with ionizing radiation)1–3 and the tendency to cause overlapping toxicities with radiation continue to pose significant restrictions on the applications of this approach. It would therefore be ideal to identify new molecular agents that can radiosensitize tumor cells and thus increase the probability of local control or even decrease the dose of radiation necessary to sterilize a given site. Recent insights into tumor biology, gained by applying the techniques and principles of normal stem cell biology to the study of cancers, suggest that in many tumors only a subset of cancer cells has the potential to divide indefinitely and to lead to tumor recurrence after treatment. It is this subset of cells, often called cancer stem cells, that needs to be targeted and radiosensitized for clinical outcomes to be further improved. The cancer stem cell hypothesis implies that identification of survival, self-renewal, and treatment resistance mechanisms in these cells may ultimately lead to improved outcomes for cancer patients.
Concepts and Principles of Normal Stem Cell Biology
The most-studied and best-understood stem cells are hematopoietic stem cells (HSCs), which serve as a model for understanding and studying all other normal-tissue stem cells. HSCs can give rise to the entire lymphohematopoietic system, including red blood cells, lymphocytes, granulocytes, monocytes, macrophages, platelets, and so on (Fig. 72-1).4 Radiation biologists played critical roles in the development of stem cell concepts and principles. James Till and Ernest McCulloch provided the first evidence for the existence of HSC in their efforts to study the ability of bone marrow transplantation to rescue lethally irradiated mice.5–7 They developed a method, called the spleen colony–forming assay, which allowed detection and quantification of stem cells based on the ability of transplanted bone marrow cells to form colonies in the spleens of previously irradiated recipients. Importantly, these colonies could be harvested and retransplanted into secondary recipients, where some gave rise to multilineage colonies, thus providing evidence for both self-renewal and differentiation potential. To this day, adaptation of the clonal in vivo assays pioneered by Till and McCulloch to other tissues and organs is the foundation of all stem cell analyses, because these assays best measure the core stem cells’ properties.
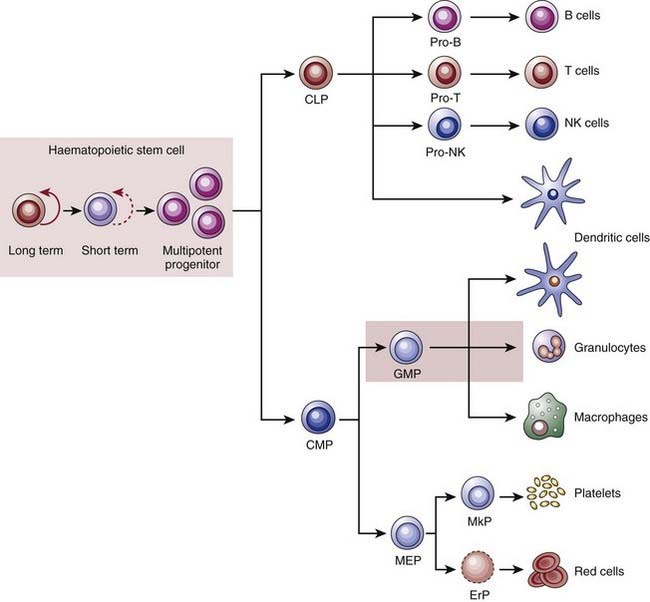
(From Reya T, Morrison SJ, Clarke MF, et al: Stem cells, cancer, and cancer stem cells, Nature 414(6859):105–111, 2001.)48
Extensive work since Till and McCulloch’s original observations has confirmed that HSCs display the three basic properties of stem cells: they self-renew, their numbers are under strict genetic control, and they give rise to a diverse collection of differentiated cells.8,9 The next critical advance in stem cell studies was the application of fluorescent-activated cell sorting (FACS) to isolate subpopulations of bone marrow cells with varied expression of specific surface proteins. This technique allows the prospective isolation of cells that can then be injected into irradiated animals to assay for repopulating activity. Using this approach, it has been determined that a small number of long-term self-renewing HSCs (which make up approximately 0.01% of mouse bone marrow cells) gives rise to short-term self renewing HSCs, which can only reconstitute an animal’s hematopoietic system for approximately 8 weeks (see Fig. 72-1). These then give rise to multipotent progenitor cells that cannot self-renew. The multipotent progenitors then further differentiate into one of several more restricted progenitor cells, including (1) the common myeloid progenitor, which ultimately gives rise to granulocytes, monocytes, erythrocytes, and platelets; (2) the common lymphoid progenitor, which gives rise to B and T cells. With the exception of the special case of memory B and T cells, HSCs are the only cells in the hierarchy that have the capacity to self-renew for extended periods under normal conditions.
A second normal-tissue stem cell that has been well characterized in recent years is the mammary stem cell. Mammary glands are made up of tubuloalveolar units consisting of a stratified epithelium made up of an inner layer of luminal epithelial cells and an outer layer of myoepithelial cells. As with HSCs, a critical step in the identification of mammary stem cells (also known as mammary repopulating units [MRUs]) was the development of an in vivo clonogenic assay. This assay consists of “clearing” mammary fat pads in 21-day-old recipient mice of endogenous epithelium and then injecting populations of dissociated mammary epithelial cells from donor animals. Approximately 6 weeks later, the injected fat pads can be harvested and analyzed for the presence of donor epithelial structures.10 Using such assays, it was initially shown that entire tubuloalveolar structures can develop from transplantation of short segments of existing mammary glands and that these result from clonal outgrowths.11–13 More recently, multiple investigators have applied FACS to prospectively isolate MRUs, just as had previously been done for HSCs. These experiments revealed that mammary glands contain a relatively small subpopulation of cells (~0.2%-0.5% of epithelial cells within a gland) that are able to generate entire epithelial outgrowths starting from a single cell.14,15 These cells give rise to differentiated luminal and myoepithelial cells and are able to do this for multiple generations of transplants, thus documenting self-renewal.
Stem Cells and Cancer
Comparisons have long been made between normal-tissue stem cells and cancer cells, and the idea that undifferentiated cells may be the origin of cancers dates back as far as the work of Rudolf Virchow.16 Clearly, at least some cancer cells must self-renew, although the tight control on this process apparent in normal stem cells appears to be relaxed or even absent in tumors. Furthermore, the concept of differentiation has long been a critical diagnostic and prognostic marker for human cancer. Thus, when pathologists “grade” tumors, they closely evaluate the degree to which a tumor represents the differentiation pattern of its tissue of origin. In many tumor types, this attribute predicts patient outcome. These observations are consistent with the idea that cancers may contain stem cells. Above all else, the concept of tumor heterogeneity suggests that cancers may contain a population of self-renewing cells that can give rise to differentiating progeny.
Competing Models of Tumor Heterogeneity
Two important clinical and experimental observations related to heterogeneity of cancer cells within tumors served as further impetus to the development of the hypothesis that tumors may contain malignant stem cells. Tumor heterogeneity refers to observations that although cancer formation is believed to be a clonal process beginning with a single transformed cell, not all malignant cells within a tumor are the same. Often, the variability within a tumor’s malignant cellular compartment is reminiscent of the normal tissue from which it is derived, and specifically of the various stages of differentiation that characterize these tissues. As such, cancer can be viewed as a caricature of the normal tissue from which it arose. For example, many moderately or well-differentiated squamous cell carcinomas form organized layers of tumor cells that are histologically similar to normal stratified squamous epithelia. Furthermore, interrogation of tumors with immunohistochemical techniques often reveals heterogeneity in the expression of differentiation markers relevant to the tissue of origin (Fig. 72-2). An extreme example of tumor heterogeneity can be found in teratocarcinomas, which can contain histologically diverse structures, including some resembling cartilage, muscle, bone, teeth, and hair. Conversely, many teratocarcinomas contain a small population of cells expressing immaturity markers such as placental alkaline phosphatase, beta–human chorionic gonadotropin, or α-fetoprotein, and elimination of these cells by chemotherapy is associated with positive clinical outcomes.17,18 Thus, many tumors contain evidence for partially intact differentiation mechanisms appropriate to their tissue of origin. This suggests that there may be a hierarchical relationship among tumor cells analogous to that seen in normal tissues.
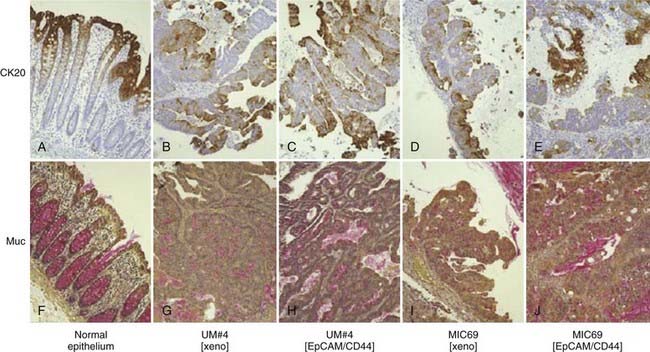
(From Dalerba P, Dylla SJ, Park IK, et al: Phenotypic characterization of human colorectal cancer stem cells, Proc Natl Acad Sci U S A 104(24):10158–10163, 2007.)38
The second major observation regarding heterogeneity of tumor cells stems from data indicating that only a small subset of cells within most tumors has the capacity to form new tumors upon in vivo transplantation or colonies upon in vitro culture. In other words, tumor cells are heterogeneous in their capacity to proliferate indefinitely. For in vivo tumor transplantation, this is reflected in the large numbers of tumor cells that generally need to be transplanted to yield a tumor. For primary human and mouse tumors, this number often resides in the thousands or millions.19–21 Similarly, less than 1% of primary tumor cells cultured in vitro are generally able to give rise to colonies (i.e., low plating efficiencies) even under the most optimal culture conditions available.21–23
Two predominant models were developed to explain tumor heterogeneity (Fig. 72-3). The first, known as the stochastic model, is the classic view and argues that all malignant cells within a tumor have the potential to give rise to new tumors, but that this can only occur under ideal conditions and therefore is a relatively rare event. In this model, histologic and molecular heterogeneity are attributed to microenvironmental differences or to genomic instability24 of cancer cells. The second model, known as the cancer stem cell model, argues that, like the normal tissues from which they are derived, tumors contain a cellular hierarchy in which only a subpopulation of malignant stem cells can proliferate indefinitely and thus give rise to new tumors. Although the remaining cancer cells, often called nontumorigenic cancer cells, can proliferate, the number of divisions they can undergo is limited and they therefore can contribute to tumor bulk but cannot form new tumors. In the cancer stem cell model, the histologic and molecular heterogeneity seen in most tumors is largely explained by the partially intact differentiation programs that exist within tumors and that attempt to recapitulate the normal development of the tissue of origin. Importantly, the well-established notion of genomic instability within cancers fits within the framework of the cancer stem cell model, and suggests that tumors may contain subclones of cancer stem cells that give rise to genomically distinct nontumorigenic cells.
Cancer Stem Cell Nomenclature
As with any field of scientific inquiry, it is critical that the terminology used in the burgeoning cancer stem cell field is similar among various investigators. This has been a problem in recent years, as various investigators have used terms such as cancer stem cells, tumorigenic cells, or tumor-initiating cells to refer to ostensibly similar but not necessarily identical subpopulations of tumor cells. With this in mind, a recent American Association for Cancer Research workshop defined cancer stem cells as those cells within a tumor that possess the capacity to self-renew and to give rise to the heterogeneous lineages of cancer cells that compose the tumor.25 For the remainder of this chapter, we use the term cancer stem cell in accordance with this definition. It is important to point out that the definition hinges on the concept of self-renewal, which, as previously discussed, is not synonymous with cellular proliferation because it also reflects the differentiation and future mitotic potential of daughter cells.
Evidence Supporting the Cancer Stem Cell Model
If the cancer stem cell hypothesis is correct in stating that only a specific subset of tumor cells has the capacity to self-renew and give rise to all of the other cells within a tumor, then it follows that immature cancer cells should be able to drive tumor formation, whereas mature tumor cells should be unable to do so. Investigations of mouse teratocarcinoma and human leukemia provided some of the earliest evidence for the existence of cancer stem cells. Teratocarcinomas arise from germ cells and produce mature cells from all three embryonic layers upon transplantation. Illmensee and Mintz showed that when placed in a normal blastocyst, teratocarcinoma cells can differentiate into mature, nontumor tissues,26,27 thus supporting the existence of differentiation mechanisms within tumors akin to those of normal tissues.
Genetic studies of leukemia by Fialkow and colleagues provided the first strong evidence for the existence of cancer stem cells. They looked at the expression of isozymes of the X chromosome–linked enzyme glucose-6-phosphate dehydrogenase (G6PD) in mature blood cells of women with leukemia and found that some of these postmitotic cells were derived from the leukemic clone.28–30 In some cases, the leukemic clone gave rise to mature cells in multiple lineages (e.g., erythrocytes, granulocytes, and platelets). This definitively showed that immature cancer cells maintained the leukemia, whereas at least some of their progeny had lost the ability to proliferate.
Given that maturity markers can, at least in part, be used to distinguish cells that maintain a tumor from those that cannot, it should be possible to prospectively isolate cancer stem cells and test their clonogenic ability. Proof of this principle was first established for acute myelogenous leukemia (AML) by applying the techniques of normal stem cell biology first pioneered in the discovery of HSCs31,32 to the study of leukemias. The general approach, which has become standard in the cancer stem cell field, was to use flow cytometry to isolate phenotypically distinct subpopulations of leukemia cells based on the variable expression of surface proteins. The sorted subpopulations were then injected into immunocompromised nonobese diabetic–severe combined immunodeficient (NOD-SCID) mice and the mice were monitored for engraftment of human leukemia. Using this approach it was determined that for the majority of AMLs, leukemic stem cells (LSCs) reside in the CD34+CD38− subpopulation, and that only these cells can lead to engraftment of human leukemia in NOD-SCID mice. The leukemic blasts, generally displaying the CD34−CD38+ immunophenotype, were unable to transplant the disease.33,34
Within a few years of this pioneering discovery, other investigators showed that cancer stem cells can also be prospectively isolated from solid tumors. This was first accomplished for human breast cancers. As for AML, NOD-SCID mice were used as xenotransplant recipients for flow cytometrically sorted subpopulations of tumor cells from primary human tumors. It was found that for all tumors analyzed, tumorigenic activity was highly enriched in cancer cells with the CD44+CD24−/low-lineage− immunophenotypes (Fig. 72-4A). Injection of as few as 100 of these cells led to tumor formation in mice whereas injection of tens of thousands of the remaining nontumorigenic cancer cells did not.35 Importantly, tumors arising in mice injected with CD44+CD24−/low-Lineage− cells contained the entire diversity of cancer cells found in the primary tumor, including cells with the cancer stem cell immunophenotype and a large population of cells with the nontumorigenic cell immunophenotype. Retransplantation of cells from first-generation xenografts led to similar results. These data indicate that human breast cancers contain a hierarchy of cancer cells.
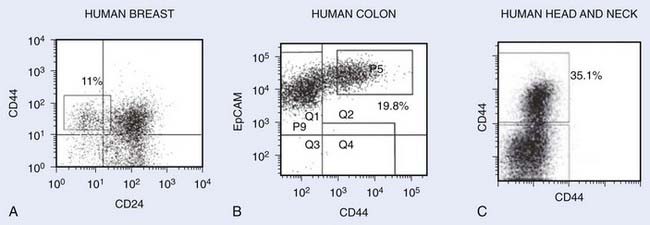
FIGURE 72-4 • Representative flow cytometry analysis of various tumors after dissociation into single-cell suspensions. Cells shown on the plots do not include dead cells and noncancer cells (i.e., lineage cells consisting of hematopoietic cells, endothelial cells, and fibroblasts). The numbers represent the percentage of cells with the markers of cancer stem cells. A, Human breast tumor with cancer stem cells exhibiting CD44+CD24−Lin− phenotype.35 B, Human colon tumor with cancer stem cells exhibiting EpCAM+CD44+Lin− phenotype.38 C, Head and neck tumor exhibiting CD44+Lin− phenotype.40