Calcium and phosphorus metabolism
Calcium and phosphorus are critical in cardiovascular, nervous, homeostatic, and muscular processes and in the function of many hormones and enzyme systems. Maternal calcium metabolism during pregnancy and lactation undergoes a series of hormone-mediated adjustments to enhance transport of this mineral to the fetus without long-term alterations in the maternal skeleton.35 Calcium serves as a second messenger; this calcium signaling is important in many reproductive processes, including fertilization, implantation and placental development and function.5
Calcium, phosphorus, and other minerals are transported across the placenta for fetal bone mineralization and skeletal growth. After birth the neonate loses the placental supply of calcium and must quickly establish homeostasis of this system to avoid metabolic derangements. This chapter discusses alterations in these substances and related hormones during pregnancy and the neonatal period. Calcium and phosphorus homeostasis in nonpregnant individuals is summarized in Box 17-1 on page 590 and in Figure 17-1. The roles of the major calcitropic hormones (parathyroid hormone, calcitonin, and vitamin D) are summarized in Table 17-1.
Table 17-1
Hormonal Actions Controlling Calcium and Phosphorus Levels
HORMONE | BONE | INTESTINE | KIDNEY |
Parathyroid hormone | Increased calcium release | Increased calcium reabsorption | |
Increased phosphorus release | Decreased phosphorus reabsorption | ||
Calcitonin | Decreased calcium release | May inhibit calcium and phosphorus reabsorption | Increased calcium excretion |
Decreased phosphorus release | Increased phosphorus excretion | ||
Vitamin D | Increased calcium release | Increased calcium absorption | Increased calcium reabsorption |
Increased phosphorus absorption | Increased phosphorus reabsorption |
Maternal physiologic adaptations
Calcium and phosphorus metabolism is altered during pregnancy, with an increase in the amount and efficiency of intestinal calcium absorption. During pregnancy, absorption increases to 50%, versus 20% to 25% in nonpregnant individuals.56,65 The increased absorption is mediated primarily by increased 1,25-dihydroxyvitamin D (1,25-[OH]2D).62 Calcium accumulation in the fetus by term totals 28 to 30 g.36,51,59 Most of this accretion (approximately 25 g) occurs in the third trimester and is used for fetal bone formation and mineralization.56 Maternal calcium metabolism undergoes further changes during lactation to meet the calcium needs of the growing infant. Understanding of changes in maternal calcium metabolism in pregnancy and lactation has grown in recent years with improved assay techniques and recognition of the roles of parathyroid hormone–related peptide (PTHrP) (see Box 17-2 on page 591).44 PTHrP increases in the first trimester and is critical for placental calcium transport and thought to help protect the maternal skeleton from excess bone loss.47 PTHrP may also help mediate changes in vitamin D and PTH.73
Antepartum period
Calcium homeostasis during pregnancy is interrelated with changes in extracellular fluid volume, renal function, and fetal needs. The mother meets the fetal requirement for calcium primarily by increasing intestinal calcium absorption. These change are mediated by increased production of 1,25-(OH)2D and PTHrP and under the influence of hormones and growth factors such as estrogens, prolactin (PRL), human placental lactogen (hPL), placental growth factor, and insulin-like growth factor-1.51,56 These substances increase intestinal absorption of calcium in pregnancy, decrease urinary excretion, alter maternal bone calcium turnover, and stimulate synthesis of both PTHrP and 1,25-(OH)2D.35,56,65 Changes in calcium and phosphorus homeostasis during pregnancy are summarized in Table 17-2 and Figure 17-2.
Table 17-2
Minerals and Hormones Involved in Calcium Homeostasis
MINERAL/HORMONES | MOTHER | FETUS | NEWBORN |
Total calcium* | Low | High | Falls‡ |
Ionized calcium* | Low normal | High | Falls |
Magnesium* | Low normal | High normal | Falls |
Phosphorus* | Low | High | Rises‡ |
Parathyroid hormone | Low | Low | Rises |
Calcitonin | Normal/High | High | Falls |
25(OH)D* | Variable | Variable | Variable |
1,25(OH)2D | High | Low | Rises |
Parathyroid hormone–related protein | High† | High |
‡Toward nonpregnant adult values.
From Nader, S. (2004). Other endocrine disorders of pregnancy. In R.K. Creasy, R. Resnik, & J.D. Iams (Eds.), Maternal-fetal medicine: Principles and practice (5th ed.). Philadelphia: Saunders.
Calcium
Maternal total serum calcium levels fall progressively beginning soon after fertilization and decrease by an average of 1 to 1.5 mg/dL (0.25 to 0.38 mmol/L). Calcium reaches its lowest levels at 28 to 32 weeks, followed by a plateau or slight rise to term.62,85 Serum calcium levels during pregnancy average 9 to 10 mg/dL (2.3 to 2.5 mmol/L)—a decrease of 5% to 6%.62 The decrease in serum calcium is a relative decrease, in that it is primarily related to and parallels the fall in serum proteins, especially albumin, with a decrease in both total and bound calcium.36,51,56 Other factors that contribute to alterations in serum calcium include increased plasma volume and hemodilution, increased urinary calcium excretion, and fetal transfer (primarily in the third trimester).44,62 Ionized calcium (physiologically active form) does not change significantly and is stable or in the low normal range.35,36,44,51
Calcium absorption occurs by active transport in the duodenum and proximal jejunum and by passive mechanisms in the distal jejunum and ileum. Intestinal absorption of calcium doubles during pregnancy, with a positive calcium balance noted by as early as 12 weeks’ gestation that continues to the third trimester.18,35,51 The early increase allows the mother to store calcium throughout pregnancy to meet the high fetal demands in the latter part of the third trimester.44 The rise in calcium absorption parallels the rise in 1,25-(OH)2D, which is the primary mediator of this change. However, since the increased intestinal absorption begins prior to the increase in 1,21,25-(OH)2D, there are likely other as yet unknown mechanisms.40 Estrogens and other hormones may up-regulate intestinal calcium transporter genes independent of the influence of vitamin D.80 Prolactin also has a calcitropic role during pregnancy as well as during lactation.17
Urinary calcium excretion parallels the rise in intestinal calcium absorption.35,36 Urinary excretion increases by 12 weeks, with an average increase from the nonpregnant value of 160 to 240 mg/dL (40 to 60 mmol/L) in the third trimester.56,62 This change is due to up-regulation of 1α-hydroxylase (enzyme involved in 1,25-[OH]2D synthesis) activity by PTHrP, estrogens, prolactin, and human placental lactogen as well as the increased glomerular filtration rate, and occurs even when the maternal diet is calcium deficient.18,65 After 36 weeks, urinary calcium excretion decreases by about 35%, increasing calcium availability by approximately 50 mg/day. Because fetal needs at this point are approximately 350 mg/day, however, other maternal calcium sources (i.e., dietary sources or the maternal skeleton) are essential.62
Parathyroid hormone
PTH levels fall to low-normal in the first trimester and may become undetectable in women with adequate calcium and vitamin D intake, and increase to mid-normal ranges by term in these women.18,36,44 Newer assays suggest PTH levels decrease to 10% to 30% of prepregnant values before increasing to term.51 The initial decrease is due to the increased 1,25-(OH)2D in response to increased PTHrP, which may contribute to changes in parathyroid function during pregnancy.6,27,35,36,51,87
Vitamin D
Both free and bound levels of 1,25-(OH)2D rise early in pregnancy, double by 10 to 12 weeks’ gestation, and remain high to term.6,35,36,44,51,65 Maternal serum levels of 1,25-(OH)2D are 50% to 100% higher by the second trimester and up to 100% higher in the third trimester.40 Vitamin D–binding protein also increases, possibly due to the increased estrogen.6,61 Changes in 1,25-(OH)2D are not mediated by PTH, because levels of this hormone are low-normal during the first trimester, but are under the influence of estrogens, PRL, hPL, and especially PTHrP, which increases levels of the enzyme 1α-hydroxylase needed for production of 1,25-(OH)2D and suppresses maternal PTH.6,18,40,51 The increased 1,25-(OH)2D comes primarily from increased production by the maternal kidney, with some from the decidua and fetoplacental unit.6,35,36,40,44,65,87 1,25-(OH)2D opens voltage-dependent calcium channels in intestinal cells to increase calcium absorption.51 Thus the doubling of 1,25-(OH)2D is paralleled by a twofold increase in intestinal calcium absorption. Serum 25-hydroxyvitamin D (25-[OH]D) levels (stored form) do not change significantly during pregnancy. The increase in 1,25-(OH)2D after 34 to 36 weeks has been associated with an increase in a vitamin D–binding protein and bound vitamin D. Thus intestinal absorption of vitamin D is enhanced throughout gestation. Renal clearance of 1,25-(OH)2D does not change during pregnancy.6
Calcitonin
Calcitonin levels are generally reported to be normal to high during pregnancy, particularly in the latter half, with about 20% of women having values outside the normal range.35,51 During pregnancy calcitonin is synthesized by the breasts and placenta in addition to the usual synthesis by the C cells of the thyroid gland. The increase in calcitonin with advancing pregnancy may stimulate the proximal renal tubule to increase 1,25-(OH)2D production.27 Increased calcitonin inhibits calcium and phosphorus release from the bones, counteracting the action of PTH (see Table 17-1). This may help prevent excessive reabsorption of bone calcium and conserve the maternal skeleton while simultaneously permitting the intestinal and renal actions of PTH and 1,25-(OH)2D to provide the additional calcium needed by the fetus.
Changes in bone formation and density
Uncoupling of bone reabsorption and formation is seen during pregnancy, with increased reabsorption during the first two trimesters and increased formation in the third trimester.51 For example, markers of bone reabsorption are reported to increase through 28 weeks’ gestation, whereas bone formation markers remain stable to 28 weeks and then increase to term.18,36,51 Maternal bone formation increases early in pregnancy with increased storage of calcium in maternal bones. Maternal bone growth is associated with increases in bone formation and reabsorption markers such as bone alkaline phosphatase and procollagen peptides in the blood.53,56,65 Osteocalcin, which normally increases with bone formation, is lower in pregnancy, although some increase is noted in late pregnancy. This may be due to increased placental calcium uptake.44,87 Bone turnover increases in the third trimester at the time of peak calcium transfer to the fetus. During this time, maternal bone stores are mobilized to meet fetal demands.35,62 However, the fetal calcium accumulation of 28 to 30 g represents only a small proportion of maternal skeletal stores. The changes in maternal bone are transient.31,56
Studies of bone mineral density during pregnancy have been inconsistent with small sample sizes and other methodological problems, including time of follow-up and other confounding factors.18,22,30,31,49,53 However, most indicate that bone mineral density decreases by 2% to 5% during pregnancy and lactation, especially in the trabecular bone sites (lumbar spine and hips).11,18,40,53,55 This is balanced by increases in the periosteal and endosteal surfaces of cortical bones (arms, legs).53,56,65,86 Individual variations are seen with an increased risk of loss with frequent pregnancies, short time between pregnancies, adolescent women, lower calcium intake during pregnancy, multiple gestation, and heparin use.56,65 There do not appear to be any long-term effects of maternal skeletal mass or bone density changes during pregnancy, and bone mineral density has been found to be similar in postmenopausal women who have or have not been pregnant.31,56 A decrease in hip fractures has been reported in several studies in women who have had children, perhaps because pregnancy-associated changes in calcium and phosphorus metabolism may improve mechanical resistance of the upper femur.56,57,61.
Intrapartum period
Calcium is essential for activation of myosin light chain kinase in smooth muscle and thus myometrial contraction. Without calcium, much of which comes from extracellular sources, myometrial contraction does not occur. PTHrP levels in the myometrium and amnion decrease at the onset of labor. The role of calcium in uterine contraction is discussed in Chapter 4.
Postpartum period
Serum calcium, PTH, and calcitonin gradually return to prepregnant values by 6 weeks postpartum in nonlactating women.36,62 Resumption of menses is associated with increases in calcium absorption, PTH, and, in most studies, 1,25-(OH)2D.65 In lactating women, changes in calcium metabolism continue in order for the mother to provide adequate calcium for infant growth and development.65 Lactation is a greater challenge to calcium homeostasis than pregnancy. During lactation the woman must provide 280 to 400 mg/day of calcium.44,56 Exclusive breastfeeding for 6 months leads to four times the calcium loss than in pregnancy.36 In the lactating women, serum calcium levels are slightly decreased with a slight increase in ionized calcium (although still within normal limits), while phosphorus, PTH, PTHrP, and 1,25-(OH)2D levels are all increased. PTH levels are low normal or slightly lower if the woman has adequate calcium and vitamin D intake.36
PTHrP, primarily from mammary tissue, increases and plays an important role in controlling breast calcium content.6,32,44,51,56 PTHrP is important in regulating movement of calcium and phosphorus from maternal bone to breast milk, renal tubular calcium reabsorption, and suppressing PTH.51 Suckling and prolactin increase PTHrP, which in conjunction with low estradiol levels, up-regulates bone reabsorption of calcium.36 PTHrP levels may show a pulsate pattern in response to suckling. Intestinal calcium absorption is not increased in lactation as it was during pregnancy. However, renal calcium excretion is reduced, conserving calcium for milk production.44,51 Thus the calcium demands of lactation are met primarily through reabsorption of maternal skeletal calcium, probably mediated primarily by PTHrP, and reduction in renal excretion of calcium.36 Changes in calcium metabolism during lactation are summarized in Figure 17-2.
Markers of bone turnover are increased in early lactation but decrease after 6 to 12 months, even with continuation of lactation.65 Maternal bone density decreases during lactation, with up to 7% of the maternal bone mass lost by 9 months’ lactation.39 These changes are most prominent in the trabecular bones of the axial skeleton and hip (e.g., pregnancy and lactation are associated with a 2% to 10% loss of bone mass in the spine and hip), in the first six months of lactation, with wide individual variation.3,18,51,56,65 Calcium losses do not continue beyond 6 months even with continued lactation.51 Calcium supplementation during lactation does not prevent these losses.18 These are reversible changes with no long-term adverse effects in most women as the maternal skeleton recovers the calcium within 3 to 6 months of weaning (with a regain of bone mineral density of 0.5% to 2% per month).18,36,56,65 Very rarely bone reabsorption may be excessive, with fractures and a clinical diagnosis of osteoporosis.35,44,65
Changes in the maternal skeleton are reversed in the later stages of lactation and with weaning. Markers of bone reabsorption increase in the first 5 to 12 months of lactation and then decrease.65 During weaning, there is decreased suckling and milk volume and increase in estradiol levels. PTHrP levels decrease.56 After weaning, PTHrP and PTH levels are elevated, intestinal absorption increases, and urinary calcium losses decrease.44,65 These changes may help the woman regain her stores. By 3 to 5 months after lactation, bone mineral status is similar or higher in lactating women than in nonlactating women, regardless of the length of lactation.65 Increases in PTH and 1,25-(OH)2D after weaning help restore the maternal skeleton.65 Lactation physiology is discussed further in Chapter 5.
Clinical implications for the pregnant woman and her fetus
Maternal nutritional needs
During pregnancy an additional 400 mg/day of both calcium and phosphorus is recommended, especially during the second and third trimesters. This results in a total calcium intake of 1200 mg/day in the pregnant woman (or 1600 mg/day in the pregnant adolescent).44 The effect of supplemental calcium intake on bone density during pregnancy is unclear, since changes in bone metabolism occur even with increased calcium intakes.65,83 There is no consistent correlation between dietary calcium intake or 1,25-(OH)2D bioavailability and intestinal calcium absorption. Hoskings notes that because the fetal skeleton contains only 28 to 30 g of calcium (far less than the average 1000 g of calcium in the adult skeleton), it is unlikely that fetal calcium needs cause clinical bone disease in the mother, but these needs may exacerbate the effects of existing low peak bone mass.27
Most studies have not shown significant increase in maternal bone mineral density with use of calcium supplements, although neonatal bone mineral density may be improved.36,51,75,77 However, women with low calcium intakes (less than 600 mg/day), adolescents, or women with multiple pregnancy may benefit from increased calcium intake or supplementation during pregnancy.1,37,56,65 Calcium supplementation during pregnancy has been associated with a reduced risk of preeclampsia (see Maternal Calcium Metabolism and Pregnancy Complications), increased birth weight, decreased risk of preterm delivery, decreased fetal lead exposure (calcium decreases circulating lead in the mother), and lower infant blood pressures.6,26,77,82 Calcium and phosphorus needs during lactation are discussed further in Chapter 5.
Vitamin D intake is critical in maintaining calcium homeostasis. Vitamin D intakes of 400 international units (10 mcg) per day are recommended in pregnancy, although some question whether these values are too low.6 Vitamin D helps ameliorate fluctuations in the calcium-to-phosphorus ratio and enhances calcium absorption. Five percent to 29% of pregnant women in the United States may have an inadequate vitamin D status.15 Alterations in calcium and bone metabolism, including increased risk of maternal osteomalacia and neonatal hypocalcemia, tend to occur primarily in women who have diets that are low in both calcium and vitamin D.44 Supplementation is recommended for women with low levels prior to pregnancy, low dietary intakes, and minimal sunlight exposure, although studies of the efficacy of supplementation are limited.36,40,50,58 Low intake of vitamin D during pregnancy has been associated with preeclampsia, gestational diabetes, increased cesarean section, bacterial vaginosis, preterm delivery, and lower weight gain and altered fetal mineral accretion, although some data for these effects are contradictory.2,6,12,15,36,38,40,82
Adequate intake of phosphorus is as important as that of calcium because these two minerals exist in a constant of solubility in the blood (see Box 17-1 on page 590). Excess dietary phosphorus binds calcium in the intestine, limiting absorption; excess blood phosphorus leads to increased urinary excretion of calcium. Therefore it is essential for the diet of pregnant and lactating women to be balanced in regard to these substances. Foods such as processed meats, snack foods, and cola drinks have high phosphorus but low calcium levels.
Leg cramps
Sudden tonic or clonic contraction of the gastrocnemius muscles and occasionally the thigh and gluteal muscles is experienced by 25% to 50% of all pregnant women.25,63,79 These cramps are felt most frequently at night or on awakening and are most common after 24 weeks’ gestation.89 Leg cramps are also more common in sedentary versus active pregnant women.
Cramps may be associated with a lower threshold for increased neuromuscular irritability due to decreased serum ionized calcium levels combined with increased serum inorganic phosphate levels, along with the hormonal and biochemical alterations of pregnancy.62,63 Systemic relaxin may decrease calcium movement into muscle, increasing the risk of leg cramps.63 The incidence of leg cramps is not correlated with ionized calcium levels. Muscular irritability in pregnancy also arises from the lowered calcium levels and mild alkalosis caused by changes in the respiratory system (see Chapter 10).63 Interventions have included reducing milk intake (although milk is rich in calcium, it also contains large amounts of phosphate); supplementation with magnesium lactate or citrate; or use of aluminum hydroxide antacids to promote formation of insoluble aluminum phosphate salts in the gut, thus reducing absorption of phosphorus.62 Young and Jewell found the best evidence for treatment with magnesium lactate or citrate.89 Calcium supplements have often been used although data to support their efficacy are weak.25,89 Thus the specific basis for leg cramps in pregnant women and the most effective interventions remain unclear.
Maternal calcium metabolism and pregnancy complications
Women with acute and chronic hypertension during pregnancy tend to have lower serum calcium and higher magnesium levels.35,51 Decreased calcium increases vascular resistance.64 The incidence of preeclampsia has been reported to vary inversely with calcium intake, primarily in women at high risk for low calcium intake.26 A recent meta-analysis of 12 studies of calcium supplementation and preeclampsia found that the risk for preeclampsia decreased by 50% (range 31% to 67% reduction) and concluded that the “reduction in preeclampsia, and in maternal death or severe morbidity, support the use of calcium supplementation, particularly for those with low dietary intake.26 Calcium supplementation during pregnancy was also found in this meta-analysis to reduce the risk of preterm birth; another more recent meta-analysis also found a significant protective benefit of calcium supplementation in the prevention of preeclampsia, but found no additional benefit in preventing preterm birth or low birthweight.16,26
Women on long-term heparin therapy for thromboembolism during pregnancy may occasionally develop heparin-induced osteopenia. Heparin inhibits 1α-hydroxylation of 25-(OH)D, decreasing levels of 1,25-(OH)2D; altering calcium homeostasis; and increasing bone calcium absorption.44,51 Calcium status should be monitored carefully in women receiving this therapy.
Disorders of the parathyroid glands are rare. The diagnosis of primary hyperparathyroidism may be obscured by pregnancy changes in calcium metabolism. Pregnancy may provide some protection to women with this disorder, with 39% to 80% becoming asymptomatic during pregnancy. This is often followed by an acute exacerbation postpartum. Moderate to severe forms of this disorder may lead to maternal hypercalcemia with fetal parathyroid suppression and hypocalcemia and risk for neonatal tetany.35,44,51,64 In women with hypoparathyroidism the normal replacement dose of vitamin D may need to be increased due to the increased vitamin D–binding hormone in pregnancy.35,44,51,64
Osteoporosis is a rare complication of pregnancy and seems to be associated with various factors—such as chronic heparin, anticonvulsant, or steroid use, low bone mineral density prepregnancy, skeletal abnormalities, or excessive reabsorption secondary to chronic inadequate calcium intake, low 1,25-(OH)2D stores, or excessive parathyroid hormone–related peptide (PTHrP)—rather than pregnancy-induced alteration in calcium metabolism.18,35,44 Similarly the also rare pregnancy-associated osteoporosis of the hip is thought to be unrelated to alterations in mineral balance in pregnancy.14,35 This condition, seen primarily in primigravida lactating women with small body builds, is self-limiting and usually resolves by 6 to 12 months postpartum.31
Maternal-fetal interactions
Maternal-placental-fetal calcium metabolism is interrelated. As noted earlier, fetal calcium accumulation is mediated by increased maternal absorption of calcium. Calcium is actively transported across the placenta, mediated primarily by PTHrP to maintain a 1:1.4 maternal to fetal calcium gradient.28,35,62,69,87 Calcium transport increases from 50 mg/day (20 weeks) to greater than 150 mg/day (mean 200-300 mg/day) at term.5,17,31,35,36,46 About 80% of fetal mineral accretion occurs after 25 weeks, with peak accretion occurring from 34 to 38 weeks’ gestation coinciding with bone development.18,29,33,34 Total fetal calcium accretion increases during pregnancy from 100 mg (4 months) and averages 25 to 30 g (generally 28-30) at term.36,51,59,64,69 Fetal serum calcium (10 to 11 mg/dL [2.5 to 2.8 mmol/L]) is about 1 mg/dL (0.25 mmol/L) above maternal values.44 The higher fetal values are primarily due to increased ionized calcium.
Fetal calcium accretion and active transport across the placenta are independent of maternal calcium levels and stores. Calcium movement across the placenta involves three phases: (1) passive bidirectional movement across the maternal-facing microvillous trophoblast membrane into the syncytiotrophoblast cytosol; (2) binding of calcium to calcium-binding proteins such as calmodulin for transport through the syncytiotrophoblast cytosol (binding buffers the calcium so that it does not disrupt cellular processes in the trophoblast); and (3) active transport across the fetal-facing basolateral trophoblast membrane into fetal circulation via several calcium channels and transporters.5,7,27,29 Placental transport of both calcium and phosphorus also involves insulin–like growth factor-1, which also stimulates 1,25-(OH)2D synthesis.69
PTHrP (see Box 17-2 page 591) regulates control of calcium transport across the placenta.28 PTHrP is the major factor in maintaining the fetal calcium level higher than maternal levels. PTHrP is also important for bone development.36 PTHrP is produced by the fetal parathyroid glands, skeletal growth plate, umbilical cord, amnion, chorion, and placenta.27,87 PTHrP levels are higher in the fetus than in adults.87 Fetal calcium levels are set at a specific level and appear to be maintained at that level regardless of maternal calcium level, even with maternal hypocalcemia.35 Although fetal calcium levels are maintained primarily by PTHrP-mediated placental calcium transport, movement of calcium in and out of fetal bone, fetal renal tubular reabsorption and excretion of calcium, and swallowing of amniotic fluid also have a role in maintaining fetal homeostasis.35 Lack of adequate PTHrP and PTH can lead to fetal growth restriction since both substances are critical for skeletal mineral accretion.69
The fetus accumulates 16 g of phosphorus, primarily in the third trimester, and 0.75 g of magnesium (with a peak of 60-75 mg/kg/day in the third trimester).46,69,70 Most of the fetal phosphorus is used for bone mineralization.69 Fetal phosphorus and magnesium levels are higher than maternal levels; these minerals are actively transported across the placenta.39,62,69,72 Magnesium is transported to the fetus in increasing amounts after the fifth month.20 Fetal magnesium levels depend on adequate placental function and maternal stores. Placental insufficiency and inadequate nutritional intake increase the risk of neonatal hypomagnesemia. Transplacental passage of magnesium is influenced by maternal level; for example, administration of large amounts of magnesium sulfate to the mother leads to elevated magnesium in both mother and fetus.23
Fetal mineral homeostasis is not heavily dependent on vitamin D.46 Placental transport of 1,25-(OH)2D is low and the fetus is a main source of this substance.4,40,62,75 The placenta also synthesizes 1,25-(OH)2D and contains 1,25-(OH)2D receptors and key enzymes such as 1-hydroxylase needed for vitamin D metabolism.4 The fetus is dependent upon maternal 25-(OH)D, which is readily transported across the placenta, because fetal hepatic enzyme processes are limited. The 25-(OH)D is 1α-hydroxylated to 1,25-(OH)2D in the fetal kidneys.35,44 Maternal vitamin D deficiency is associated with an increased incidence and severity of neonatal hypocalcemia. PTH and calcitonin do not appear to cross the placenta.44,62,75 Vitamin D is an important factor in the regulation of cellular differentiation and apoptosis.41 Insufficient vitamin D during gestation can affect development of the fetal skeleton, immune system, and brain and may alter fetal programming, increasing the risk of adult-onset disorders.41 Maternal vitamin D deficiency during pregnancy has been associated with neonatal hypocalcemia, impaired growth, and later problems in offspring including skeletal problems, type 1 diabetes, altered immunotolerance, risk of autoimmune disease, food allergies, and altered programming of long bone development.6,13,36,40,41,42,71,82
Summary
Calcium and phosphorus are essential minerals for many body processes and growth. Alterations in metabolic processes related to these elements during pregnancy can alter maternal, fetal, and infant health status. Health can be promoted by careful assessment and monitoring of maternal and fetal status and initiation of appropriate interventions. Recommendations for clinical practice related to calcium and phosphorus metabolism during pregnancy are summarized in Table 17-3.
Table 17-3
Recognize the usual changes in calcium and phosphorus metabolism during pregnancy (pp. 589-592, Figure 17-2, and Table 17-2).
Assess and monitor maternal nutrition in terms of calcium, phosphorus, and vitamin D intake (p. 594).
Counsel women regarding calcium, phosphorus, and vitamin D requirements to meet maternal and fetal needs during pregnancy (pp. 594-596).
Monitor fetal growth (pp. 595-596).
Know usual parameters for serum calcium during pregnancy (p. 589).
Evaluate diet of women complaining of leg cramps (p. 594).
Counsel women regarding leg cramps and appropriate interventions (p. 594).
Recognize usual changes in calcium and phosphorus metabolism during lactation (p. 593, Figure 17-2).
Assess and monitor nutrition during lactation in terms of calcium, phosphorus, and vitamin D intake (p. 593).
Counsel women regarding calcium, phosphorus, and vitamin D requirements to meet maternal needs during lactation (pp. 593-594 and Chapter 5).
Counsel women with pregnancy complications regarding calcium intake during pregnancy (pp. 594-595).
Development of calcium and phosphorus metabolism in the fetus
Anatomic development
Calcium and phosphorus metabolism is regulated by a variety of hormones, including parathyroid hormone (PTH), vitamin D, and calcitonin. This section reviews development of the parathyroid glands; development of the thyroid glands (site of calcitonin synthesis) is discussed in Chapter 19. Because calcium and phosphorus are critical for bone mineralization processes, skeletal growth is also considered.
Parathyroid glands
Many structures of the head and neck—the maxillary process, mandibular arch, several muscles of the jaw, hyoid and ear bones, thyroid, and cricoid cartilage—develop from the branchial or pharyngeal arches. These are bars of mesenchymal tissue separated by pharyngeal clefts. The pharyngeal pouches are outpouchings along the lateral walls of the pharyngeal gut. Structures that develop from these pouches include the palatine tonsils, thymus, primitive tympanic cavity, and (from the third and fourth pouches) the parathyroid glands (Figure 17-3).48
The third and fourth pharyngeal pouches develop bulbar and ventral portions. The inferior parathyroid glands differentiate from the dorsal bulbar portion of the third pharyngeal pouch during the sixth week. The ventral portion of this pouch forms the thymus. The parathyroid glands initially migrate caudally and medially with the thymus, later separating and attaching to the inferior portion of the dorsal surface of the descending thyroid gland (see Chapter 19). The superior parathyroid glands develop from the dorsal bulbar portion of the fourth pharyngeal pouch by the sixth week and attach to the superior portion of the dorsal side of the caudally migrating thyroid gland (see Figure 17-3).48 Parathyroid glands are active by 12 weeks, but function is suppressed by the high serum calcium concentrations in the fetus.23,51,69
Skeletal development and growth
Skeletal growth occurs in two phases. During fetal life a cartilage anlage (primordium) is formed that is later replaced by bone. Bone formation also occurs by differentiation of mesenchyme directly into bone cells. Later linear growth depends on cartilaginous growth in the endochondral ossification centers at the epiphyses; appositional growth of the skeleton depends on the laying down of new bone by bone-forming cells with subsequent remodeling (reabsorption of existing bone followed by formation of new bone). Bone formation and remodeling are a cyclic process that occurs continuously throughout life. During growth, bone formation is greater than remodeling. Once maximal growth is achieved, the skeletal mass is stable for 10 to 15 years, and remodeling and bone formation occur at the same rate. With aging, remodeling is greater than bone formation, with a gradual loss of bone mass. Excessive differences between bone formation and remodeling can lead to osteoporosis and compromised skeletal integrity. Stress, such as subjecting bone to heavy loads, or stress that occurs with strenuous exercise, stimulates osteoblastic deposition of new bone, leading to thickening of bones.24,67
Skeletal development begins in early embryonic life (apparent by the eighth week) and continues well into postnatal life.51 Bone consists primarily of organic matrix and bone salts. Compact bone is 30% organic matrix and 70% bone salts; newer bone has a more organic matrix. The organic matrix is composed primarily of collagen fibers that give the bone its tensile strength. The rest of the matrix is ground substance, consisting of extracellular fluid and proteoglycans, which may assist in controlling deposition of calcium salts. Crystalline bone salts (hydroxyapatites) give bone compressional strength and consist primarily of calcium and phosphorus with small deposits of sodium, potassium, magnesium, and carbonate salts.24,67
Bone is formed by either intramembranous or endochondral ossification. Intramembranous ossification is the process involved in formation of bones such as the skull, mandible, and maxilla.67,69 With intramembranous ossification, the fibrous mesenchyme condenses to form a collagenous membrane in which some cells differentiate into osteoblasts. Osteoblasts produce a collagenous material and ground substance to fill the extracellular spaces. The collagen polymerizes to form collagen fibers and the tissue becomes osteoid and similar to cartilage.67,69 Osteoblasts later secrete alkaline phosphatase, which leads to deposition of calcium salts in the form of calcium hydroxyapatite crystals, with gradual conversion of the osteoid to bone. Some osteoblasts are trapped within lacunae in the bone matrix and develop into osteocytes. The bone matrix grows in all directions as spicules and ossification centers are established.
The osteoblasts deposit spongy bone first, followed by plates of compact bone (periosteal ossification). Spongy bones are filled with fibrous and cellular mesenchymal derivatives that later differentiate into elements characteristic of red bone marrow (reticular tissue, fat cells, sinusoids, and developing blood cells). Bone growth is accompanied by remodeling in which much of the original matrix is reabsorbed by osteoclasts simultaneously with formation of new bone by osteoblasts. During this process the osteoclasts project villi that secrete proteolytic enzymes to dissolve the organic matrix and citric, lactic, and other acids that cause solution of bone salts.24
The long bones of the appendicular and axial skeleton form by endochondral ossification in which the condensed mesenchymal cells give rise to hyaline cartilage models that are shaped like the eventual bone. This cartilage is eroded locally and destroyed as bone is formed. Endochondral ossification involves the progressive destruction of cartilage, deposition of calcium salts, and formation of a central area of spongy bone (that will later develop a red marrow matrix) surrounded by compact bone. This process begins in the middle of the bone shaft and progresses toward the epiphysis. At birth the long bones consist of central ossification centers and bony shafts with cartilaginous ends. Secondary areas of ossification later appear in the epiphyses.48,67
Bone growth and mineralization are mediated by a variety of regulating hormones and growth factors including calcitropic hormones (e.g., PTH, vitamin D, calcitonin); parathyroid hormone–related peptide (PTHrP); systemic growth-regulating hormones (e.g., growth hormone, insulin, glucocorticoids, thyroid hormones, sex steroids); circulating growth factors (e.g., somatomedin, insulin-like growth factor [IGF], epidermal growth factor, platelet-derived growth factor, fibroblast growth factor); and local factors (e.g., osteoclast activity factor, cartilage-derived growth factor).67,69,72 In addition, maternal diet (especially vitamin D), physical activity, and smoking during pregnancy may also influence fetal bone mineral acquisition.19
Functional development
Fetal mineral requirements are met by transport of calcium, phosphorus, magnesium, and other minerals across the placenta (see Maternal-Fetal Interactions). Eighty percent of calcium and phosphorus accretion occurs in the third trimester.72 From 25 weeks’ gestation to term, bone mineralization increases fourfold and fetal calcium acquisition ranges from 92 to 119 mg/kg/day or higher (up to 350 mg/day or a mean of 200 mg/day at term) and phosphorous from 2.51 to 3.44 mg/kg/day (Figure 17-4).33,35 In contrast, calcium accretion immediately after birth increases from 15 mg/kg on day 1 to 45 mg/kg on day 3.20 Phosphate levels peak at midgestation (15 mg/dL [4.8 mmol/L]) and then decrease to 5.5 to 7 mg/dL (1.8 to 2.3 mmol/L) by term.
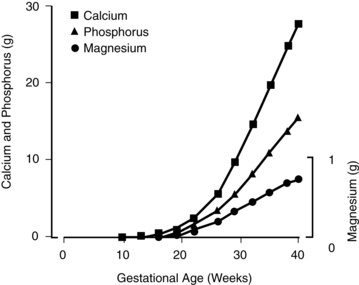
1,25-(OH)2D and PTH levels are low in the fetus and probably have a limited role in fetal calcium physiology.35,46 The parathyroid gland contains PTH by 10 to 12 weeks and actively secretes PTH by 25 to 26 weeks in response to decreased extracellular fluid calcium.6,48 The fetal parathyroid is less responsive to decreased serum calcium, perhaps because of suppression of the parathyroid by the relative fetal hypercalcemia or placental PTH production.6,28,62 The predominant hormone regulating fetal calcium homeostasis is PTHrP (see Box 17-2 on page 591) rather than PTH as occurs after birth and in adults, although PTHrP and PTH act synergystically.27,69
Cord concentrations of vitamin D metabolites are only about 20% of maternal levels.6,36 25-(OH)D is transferred from the mother since fetal liver processes for vitamin D metabolism are limited.35 Renal 1α-hydroxylation to form 1,25-(OH)2D occurs in the fetal kidneys, placenta, and decidua.46 The fetus needs to store vitamin D to cope with the relatively high calcium requirements of the early postbirth period. Vitamin D deficiency during fetal development can alter bone growth and development as well as other areas of development in the infant and child and has implications for the development of other disorders.1,46 These implications and fetal vitamin D synthesis are described further in Maternal-Fetal Interactions on pp. 595-596.
Calcitonin-containing cells appear in the thyroid at about 14 weeks’ gestation and secrete immunoreactive calcitonin from 28 weeks.46 Calcitonin levels are high in the fetus, with increasing concentrations during the third trimester. The role of calcitonin in the fetus is unclear; however, animal evidence suggests that calcitonin does not have a major role in fetal bone metabolism.35,46
Neonatal physiology
The newborn is relatively hypercalcemic and hyperphosphatemic when compared with the mother. The infant must quickly move from the intrauterine dependence on maternal calcium sources and placental hormones to independent extrauterine control of calcium and phosphorus metabolism and homeostasis with reliance on oral intake and bone stores. Failure to do so may lead to hypocalcemia or other metabolic abnormalities. Calcium and phosphorus homeostasis is summarized in Box 17-1 on page 590 and in Figure 17-1. The roles of the major calcitropic hormones (parathyroid hormone, calcitonin, and vitamin D) are summarized in Table 17-1.
Transitional events
At birth, maternal supplies of calcium and other minerals are no longer available to the infant. Within 24-48 hours of birth, the infant moves from the hypercalcemic, peptide PTHrP-dominated calcium metabolism to a parathyroid hormone (PTH) and 1,25-(OH)2D environment. In this environment, the infant must mobilize bone calcium and increase intestinal absorption to maintain serum calcium levels.35 Total and ionized calcium levels are higher in cord blood than in maternal serum; PTH is decreased, but PTHrP is increased.21,23,35,62,70 Cord blood magnesium levels are slightly increased and related to maternal levels, whereas phosphorus levels are low.21,69 Cord blood levels of calcitonin are reported to be 68% to 108% of maternal levels and correlate with maternal levels.23
Maternal serum 25-(OH)D levels at term correlate with cord blood levels, although cord blood values are about 20% lower; 1,25-(OH)2D levels are about half maternal values.6,46 At birth serum osteocalcin levels are two to three times higher than in adults. This reflects the rapid rate of fetal bone formation in the last weeks of pregnancy. Osteocalcin is a noncollagenous bone-specific protein that is released into the blood in proportion to the amount of bone formation. Levels increase from birth to 1 to 5 days and then decrease. Markers of bone formation decrease and markers of bone reabsorption increase for a few days after birth due to the transition to dependence on intestinal mineral absorption and the relatively low intake immediately after birth. After that time, both types of markers increase over the next few weeks.18
Calcium
The relative hypercalcemia at birth is followed by a physiologic hypocalcemia as both total and ionized calcium fall to levels lower than those found in older infants and about 1 mg/dL (0.25 mmol/L) lower than birth values.23,46,62 There is a rapid decrease in ionized calcium, especially in the first 4 to 6 hours after birth, reaching a nadir of 4.4 to 5.4 mg/dL (1.1 to 1.4 mmol/L) by 16 to 24 hours.35,37,46,54 Calcium levels generally stabilize in the next 24 to 48 hours and then increase along with increases in PTH and 1,25-(OH)2D.23,35 The fall in calcium is thought to be due to parathyroid suppression in late gestation (from elevated fetal calcium levels), loss of placental transport of calcium, and an increased PTH response.35,46 This nadir is more pronounced in infants who are preterm or born to mothers with diabetes or vitamin D deficiency.36 In term infants, total calcium values average 8 to 9 mg/dL (2 to 2.2 mmol/L), with a range of 8 to 11 mg/dL (2 to 2.8 mmol/L), and are slightly lower in preterm infants. Ionized calcium levels are 4 to 4.6 mg/dL (1 to 1.5 mmol/L).28,69 Calcium levels at birth correlate inversely with gestational age.33,62,69 The length and degree of the postbirth physiologic hypocalcemia are also correlated inversely with gestational age.23,69 In very low–birth weight (VLBW) infants this hypocalcemia may persist despite increasing levels of PTH and 1,25-(OH)2D.23,36
Intestinal absorption of calcium is correlated with both gestational and postbirth age, but the major factor in determining absorption is postnatal age.23 Immature intestinal function and decreased intake may limit calcium absorption. Much of the intestinal transport is primarily passive (via paracellular transport) in preterm infants since transcellular active transport, which is vitamin D dependent, is not yet mature.69 Renal calcium excretion is relatively efficient in both term and preterm infants, although increased renal sodium losses in VLBW infants may also increase calcium loss.23,69 Renal calcium excretion increases with gestational and postbirth age, ranging from 60 to 88 mg/day during the first 2 weeks to 180 mg/day by 3 to 12 weeks in the term infant and from less than 8 to 80 mg/day to 120 mg/day by 2 weeks of age in the preterm infant.20
Phosphorus
Phosphorus levels may decrease slightly during the first 1 to 2 days after birth but remain higher than those of adults.62 Renal excretion of phosphorus is delayed because of a decreased glomerular filtration rate and increased tubular reabsorption of phosphorus. Increased energy demands during birth with conversion of adenosine triphosphate (ATP) to adenosine diphosphate (ADP) lead to additional phosphorus release. Delayed feeding further elevates phosphorus levels because of tissue catabolism. Phosphorus levels are lower in small-for-gestational-age (SGA) infants and correlate with the degree of growth restriction. Levels are higher in formula-fed than breastfed infants and inversely related to serum calcium concentrations.69 The fractional excretion of phosphorus is increased in preterm infants, increasing the risk of phosphate deficiency.69
Parathyroid hormone
As serum calcium levels decrease over the first few days after birth, PTH levels gradually increase by about 24 to 48 hours.37 Levels are higher in hypocalcemic preterm infants.23 Preterm infants may have a transient pseudohypoparathyroidism for the first 72 hours secondary to immature bone and renal responses to PTH.23 By 3 to 4 days of age, the preterm infant’s parathyroid gland generally responds effectively to calcium. The infant of a diabetic mother may also have impaired PTH production initially; infants following perinatal hypoxic ischemic events may have a decreased PTH response to low calcium.69 PTH levels do not appear to change with oral administration of calcium supplements, but intravenous bolus administration of calcium can suppress parathyroid function.23 PTHrP decreases rapidly after birth to the low levels normally seen in adults. Its role in neonatal calcium homeostasis is unclear, but PTH is the major calcitropic hormone by 48 hours of age.35 The response of the neonatal kidneys to PTH is not well understood, although both term and preterm infants do respond to exogenous PTH.28 The amount of calcium excreted by the kidneys increases over the first 2 weeks after birth, perhaps mediated by the increasing glomerular filtration rate (see Chapter 11).28
Vitamin D
Plasma concentrations of 25-(OH)D are lower than and correlate with maternal serum values and remain stable in both term and preterm infants in the first week.23 Term infants are able to effectively metabolize vitamin D in the liver and kidneys. The kidneys can convert 25-(OH)D to 1,25-(OH)2D by 28 to 32 weeks’ gestational age. However, vitamin D metabolism remains limited in the preterm infant because 25-hydroxylation by the liver does not occur at significant rates until 36 to 38 weeks’ gestation.75 In VLBW infants born at less than 28 weeks gestation the vitamin D activation pathway may remain immature.69 Absorption of exogenous vitamin D may also be limited because of immature fat absorption. Serum 1,25-(OH)2D levels increase during the first 48 hours, probably because of decreased serum calcium or increased PTH, and elevated levels persist for the first week.56 The increase parallels the decrease in serum calcium and increase in PTH in both term and preterm infants.23 This helps maintain calcium levels within physiologic limits by stimulating bone and intestinal reabsorption.23,35 In preterm infants, levels may remain higher for up to 7 to 9 weeks, reflecting rapid prenatal growth.23
Calcitonin
Calcitonin levels may be normal or slightly elevated at birth, followed by a surge that is reported to peak anywhere from two- to 10-fold over cord blood levels by 24 to 48 hours of age.69 Levels then plateau and decrease to childhood levels by 1 month.23,52 Calcitonin levels are inversely correlated with gestational age.52 Increased calcitonin may protect the infant from excessive bone reabsorption and promote mineralization during a period of active bone growth in the face of increased PTH and 1,25-(OH)2D. High calcitonin levels may contribute to the lower serum calcium levels and increased risk of hypocalcemia seen in preterm infants. Calcitonin levels remain high in preterm infants for a longer period of time, slowly decreasing over the first 2 to 3 months to reach normal levels by about 40 weeks’ postmenstural age.52
Clinical implications for neonatal care
A calcium-to-phosphorus ratio of 1.7:1 to 2.0:1 is thought to be ideal for human infants.20,69 The ratio of calcium to phosphorus can have a significant impact on mineral homeostasis. Hyperphosphatemia may lead to hypocalcemia by blunting the responsiveness of the bone to parathyroid hormone (PTH) and vitamin D. Conversely, low serum phosphorus levels can lead to reduction of calcium entry into bone, bone demineralization, and hypercalcemia.20 Infant formulas have higher phosphorus and lower ionized calcium concentrations than human milk. However, currently most commercial formulas have ratios that more closely approximate those of human milk.69,75 Although of calcium are lower in human milk than in cow’s milk formulas; the ratio of calcium to phosphorus promotes calcium-phosphorus homeostasis. The efficiency of intestinal calcium absorption increases up to twofold with human milk feedings. The American Academy of Pediatrics recommends that exclusively breastfed infants receive 400 international units of vitamin D daily (10 μg/day) beginning soon after birth, although compliance with this recommendation is low.60,84 Calcium and phosphorus levels of human milk are not adequate initially for VLBW infants, and supplementation is recommended (see Chapter 12).78
Calcium intake in preterm infants
Preterm infants may have difficulty maintaining an adequate calcium intake because of increased growth needs and a low intake. Calcium needs are increased in VLBW infants since they have missed the third trimester when much of the fetal calcium accretion occurs.72 In addition medications such as phenytoin, phenobarbital, and glucocorticoids can decrease intestinal calcium absorption.69 Calcium levels in mature breast milk and standard formulas are significantly below daily intrauterine calcium accretion rates in the third trimester.72 As a result, bone mineralization is reduced in infants fed these feedings. Preterm infants fed breast milk do have increased calcium absorption (60% to 70% versus 35% to 60% with formulas).23,67 Initially, extremely low–birth weight (ELBW) infants will need parenteral nutrition to supply calcium and phosphorus, although this often does not match intrauterine accretion rates.23,67 Increased levels of calcium and phosphorus can be delivered with formulations containing calcium glycerophosphate and monobasic phosphate (up to 86 mg/dL [21.5 mmol/L] of calcium and 46 mg/dL [14.9 mmol/L] of phosphorus). Fortification of human milk can increase calcium retention up to 60 mg/kg/day, and if the fortifier contains calcium glycerophosphate, up to 90 mg/kg/day.67 If supplementation is used for preterm infants fed human milk, calcium-to-phosphorus ratios of 1.7:1 are recommended to maximize intake and retention.67,69 Ratios should not be greater than 2:1 to prevent hyperphosphatemia. Preterm formulas come closer to duplicating intrauterine calcium accretion rates during the last weeks of the third trimester (120 to 150 mg/kg/day), but have a lower bioavailability of calcium than human milk.23,33,68,69 Vitamin D levels are increased in these formulas to enhance intestinal calcium absorption. Medium-chain triglycerides (MCTs) are also added to increase fat absorption and thus absorption of vitamin D and calcium.
Bone mineralization
After birth the neonatal skeleton continues to accrete calcium at a rate of approximately 150 mg/kg/day.35,37 To accomplish this, the infant must have adequate vitamin D and intestinal absorption of calcium. This may be difficult to achieve in VLBW and ELBW infants. Rigo and colleagues note that the goal for VLBW infants is postnatal growth similar to the intrauterine rate, with a slightly higher rate in ELBW infants.67 To reach this goal, these infants need not only adequate supplies of calcium, phosphorus, and other minerals, but also protein and energy for formation of the collagen matrix.67,69 VLBW infants have decreased postnatal bone mineralization and a significant delay in completing bone development in comparison with intrauterine rates.10 Prolonged (greater than 5 days) maternal magnesium sulfate administration has been reported to alter neonatal bone mineralization.88
Preterm infants are at risk for both rickets and osteopenia (reduction of bone mass with demineralization of the bone with or without signs of rickets). By term-corrected age, VLBW infants are still of lower weight and length than term infants with linear growth restriction and lower bone mineral mass and density seen in up to 22% of AGA preterm infants.8,37 Land and Schoenau suggest that one reason for this difference is that the fetus in utero experiences higher mechanical resistance to extremity movement than occurs after birth. Thus the preterm infant’s movements occur against lower resistance, which provides less stimulation for development.37 Significant bone mineralization problems, ranging from osteopenia to rickets, are seen in more than 30% of ELBW infants.33,67 Mineralization may also be delayed in SGA infants. Longitudinal follow-up is important after discharge to promote catch-up growth and optimal bone mineralization.67 Factors leading to inadequate bone mineralization are illustrated in Figure 17-5.
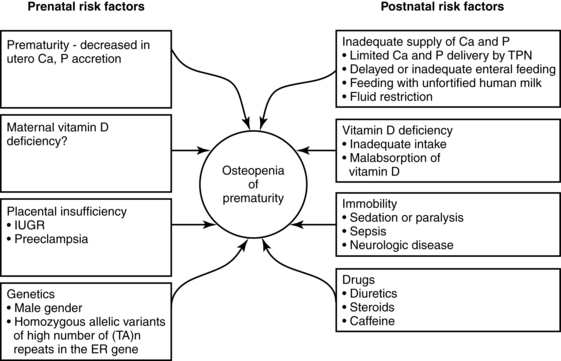
Alterations in neonatal calcium homeostasis
Neonatal hypocalcemia
The serum calcium level below which an infant is considered to be hypocalcemic varies in the literature from 7 to 8 mg/dL (1.75 to 2 mmol/L).33,62,69,70 Most sources use a lower limit of 7 mg/dL (1.75 mmol/L) in preterm and 7.8 to 8 mg/dL (1.95 to 2 mmol/L) in term infants.23,33 Ideally determination of hypocalcemia should be based on the ionized calcium fraction (less than 4.4 mg/dL [1.1 mmol/L]) because this is the biologically active form.8,20,23,33 In preterm infants the relationship between total and ionized calcium is atypical, so infants may have a greater decrease in total serum calcium than in ionized calcium.28
Hypoproteinemia or acid-base changes can alter calcium levels. Serum calcium is either ionized (physiologically active form) or undissociated and bound to protein or complexed to anions. Because these two forms of serum calcium are in equilibrium, hypoproteinemia lowers serum calcium levels. This equilibrium is influenced by acid-base status. Acidosis increases the movement of calcium from bone and decreases the amount of protein-bound calcium. As a result, levels of ionized calcium increase. Opposite effects are seen during alkalosis.20
Infants at greatest risk for hypocalcemia are preterm infants, those born to diabetic mothers, and those born after perinatal hypoxic-ischemic events. Possible pathogenic mechanisms are discussed below and are summarized in Table 17-4. Hypocalcemia due to decreased ionized calcium may occur after exchange transfusion, with renal dysfunction, after furosemide therapy, or with magnesium deficiency. Hypocalcemia with a decrease in ionized calcium but without a decrease in total calcium can occur with alkalosis, after exchange transfusion with citrated blood, or with elevated serum free fatty acids after lipid infusion.20 Alkalosis leads to a decreased affinity of albumin for calcium and thus less ionized calcium. A rapid shift in the amounts of ionized versus albumin-bound calcium can lead to significant hypocalcemia.69 Hypocalcemia in infants with fetal growth restriction is usually associated with prematurity or perinatal hypoxic-ischemic events.33
Table 17-4
Possible Pathogenic Mechanisms in Early Neonatal Hypocalcemia
CLINICAL PARAMETERS | POSSIBLE PATHOGENIC MECHANISMS |
Prematurity |
Hypocalcemia in the preterm infant.
Hypocalcemia is seen frequently in preterm infants, and is inversely related to birth weight and gestational age, with serum calcium levels lower than 7 mg/dL (1.8 mmol/L) (total) or 3 to 3.5 mg/dL (0.75 to 0.88 mmol/L) (ionized) reported in 30% to 40% of preterm infants and up to half of VLBW infants.33,69,70 The hypocalcemic preterm infant is often asymptomatic, possibly because the ionized (physiologically active) calcium is not decreased to the same degree as the total serum calcium resulting in a higher ratio of ionized to total calcium.70 The hypocalcemia usually occurs within the first 24 to 48 hours after birth, often sooner than in term infants.28 Preterm infants are at greater risk since the rapid skeletal accretion of calcium seen in the fetus in the last weeks of gestation continues after birth, but in an environment without the benefit of the placental calcium pump and with immature intestinal absorption.35 Possible causes of hypocalcemia in preterm infants include lack of available calcium with decreased early intake, insufficient PTH release, and thus decreased PTH availability to move calcium out of the bones (although some recent studies do not support immaturity in parathyroid function in these infants), immature absorption, interference with calcium metabolism by acidosis, increased calcitonin levels, decreased vitamin D intake and absorption, and 1,25-(OH)2D in VLBW infants.20,23,33,69,70
Preterm infants may have an imbalance between calcium needs and PTH production, with increased calcium needs due to growth and a decreased supply as well as inadequate PTH response by the renal tubular cells.69 Increased renal sodium losses in VLBW infants may aggravate calcium loss.69
The preterm infant has a marked elevation of calcitonin at 2 to 5 hours that reaches a plateau at 12 to 24 hours; this may also contribute to the risk of hypocalcemia.69 Serum calcium values are negatively correlated with calcitonin at 12 to 24 hours of age, possibly impeding resolution of the hypocalcemia. Alterations in vitamin D metabolism or availability may also impede resolution. Hyperphosphatemia has also been observed, along with an occasional transient decrease in magnesium at 24 to 48 hours.33 The decrease in magnesium can be prevented by calcium, suggesting that hypomagnesemia is a consequence and not a cause of this hypocalcemia.33
Hypocalcemia in the infant of a diabetic mother.
Hypocalcemia occurs in about 50% of infants of insulin-dependent diabetic women, with an increased incidence also reported in infants of women with gestational diabetes.33,69 A lower incidence is seen in infants of women with tight maternal glycemic control during pregnancy. This form of hypocalcemia tends to appear within the first 24 hours of age and to be more severe and last longer than the hypocalcemia seen in preterm infants. The decrease in calcium level is directly correlated with the severity of the maternal diabetes.69
Cord blood of these infants contains decreased levels of PTH and of both total and ionic calcium. The cause of this form of hypocalcemia may be related to suppression of the fetal parathyroid by maternal hypomagnesemia and a relative maternal hyperparathyroidism seen in many diabetic women. These metabolic disorders in diabetic women are believed to be caused by increased urinary losses and may lead to chronic fetal hypomagnesemia and decreased PTH secretion.20 This results in a transient neonatal hypoparathyroidism. The pregnant diabetic woman has lower magnesium levels and a failure of the usual increase in PTH, although serum total and ionic calcium values are often within normal pregnancy limits.
Hypocalcemia in the infant of a diabetic mother (IDM) may also be related to immaturity in that it is seen more frequently in infants who are also immature. Because these infants are often hypomagnesemic also, magnesium therapy may be needed to correct both the hypocalcemia and hypomagnesemia.62 The increased bone mass is secondary to the effects of insulin and insulin-like growth factor-1 on bone formation. Because the increased bone mass increases calcium needs, this may also be a factor in the neonatal hypocalcemia in these infants.
Hypocalcemia and perinatal hypoxic-ischemic events.
With these events, tissue breakdown increases with release of phosphorus as well as accelerated conversion of ATP to ADP (to meet the increased energy demands), with subsequent phosphorus release. Administration of bicarbonate or hyperventilation with mechanical ventilators both increase pH, which alters protein binding and decreases ionized calcium.23 Alkalosis from bicarbonate therapy reduces the flux of calcium from bone and reduces serum ionized calcium.20 These events are also associated with an impairment of parathyroid function, increased calcitonin levels, and altered serum magnesium and phosphorus.69
Late neonatal hypocalcemia.
Late hypocalcemia is seen infrequently, but is more frequent in term than preterm infants and usually seen at 5-10 days.69 Late neonatal hypocalcemia may be due to decreased renal responses to PTH so that the kidneys reabsorb more phosphorus, which leads to a reduction in serum calcium levels, and may be exacerbated by the lower glomerular filtration rates in the neonate. This problem used to be seen primarily in formula-fed term infants with increased phosphate intake, but is uncommon with current formulas (although formulas continue to have higher phosphorus levels than breast milk).69 Other less frequent causes are malabsorption of calcium, hypomagnesemia, and hypoparathyroidism. Late hypocalcemia may be asymptomatic but is associated with tetany. Symptoms range from mild tremors to seizures.
These infants demonstrate hypoparathyroidism in the face of decreased serum calcium and a reduction in efficient parathyroid activity that extends the usual postnatal decrease in calcium beyond 72 hours. The cause of persistent hypoparathyroidism is unknown. These changes may be aggravated by other factors such as vitamin D deficiency or high phosphorus loads from cow’s milk formulas.33 Late hypocalcemia was seen more often in the past, when higher phosphate formulas were more common. The disorder is uncommon with current formulas and essentially nonexistent in breastfed infants. Late hypocalcemia is occasionally seen in preterm infants secondary to immature renal function, transient parathyroid dysfunction, or altered responsiveness to 1,25-(OH)2D.
Neonatal hypercalcemia
Hypercalcemia is rare in the neonate and is generally defined as a serum calcium level over 11.0 mg/dL (2.75 mmol/L), or ionized calcium over 5.4 mg/dL (1.35 mmol/L).69 Infants may be asymptomatic, especially if calcium is in the 11 to 13 mg/dL (2.8 to 3.3 mmol/L) range, or symptomatic (hypotonia, poor feeding, lethargy, vomiting, seizures, polyuria, and hypertension). Neurologic manifestations arise from the effects of calcium on nerve cells and cerebral ischemia.28 Polyuria is due to interference with the action of arginine vasopressin on the collecting ducts and can lead to dehydration. Hypertension is related to the vasoconstrictive effects of calcium and subsequent increased activity of the renin-angiotensin system. Hypercalcemia may be idiopathic, iatrogenic, or due to hypervitaminosis (vitamin A or D) or underlying metabolic or genetic disorders.
Alterations in neonatal magnesium homeostasis
Hypomagnesemia in the neonate (less than 1.6 mg/dL [0.66 mmol/L]) occurs most often in infants who are SGA, preterm, or born to insulin-dependent diabetic women.69 Clinical signs are often not seen until levels are less than 1.2 mg/dL (less than 0.49 mmol/L). Less frequent causes of neonatal hypomagnesemia include decreased intake due to malabsorption or short-bowel syndrome, increased losses with frequent exchange transfusions or loop diuretic use or aminoglycosides, maternal hyperparathyroidism, neonatal hypoparathyroidism, and hyperphosphatemia from cow’s milk formula.23 Occasionally tissue hypomagnesemia may be present with normal serum magnesium levels.33 Hypocalcemia and hypomagnesemia often occur simultaneously and symptoms are similar. Magnesium plays an important role in bone-serum calcium homeostasis and intestinal absorption of calcium (see Box 17-1 on p. 590). Decreased magnesium inhibits PTH secretion and response, with subsequent reduction in calcium levels.69 Transient hypomagnesemia due to magnesium depletion is sometimes seen with use of loop diuretics, aminoglycosides, amphotericin B, or in infants with renal problems.69
Hypermagnesemia (greater than 2.8 mg/dL [1.15 mmol/L]) is most often associated with administration of magnesium sulfate to the mother for treatment of preeclampsia or preterm labor prevention.69 This can lead to elevated neonatal magnesium levels during the first 48 hours and respiratory depression, hypotonia, flaccidity, ileus, and poor feeding (magnesium has a curare-like effect). These infants have lower calcium levels, although they are not necessarily hypocalcemic. Hypermagnesemia may be treated with calcium, which increases magnesium excretion.23
Neonatal osteopenia and rickets
Preterm infants, especially those ≤1500-g birth weight, are at increased risk of metabolic bone disease including osteopenia, rickets, and fractures.10,37,47,74 Osteopenia is a reduction in the bone of the skeleton often accompanied by osteomalacia (failure of mineralization of newly formed osteoid leading to hypomineralization of the bone matrix); rickets involves specific histologic, radiographic, and physical findings with accumulation of unmineralized osteoid, which interrupts bone growth plate mineralization.23,37,47 In preterm infants, osteopenia develops from inadequate mineral stores exacerbated by the mineral demands of extrauterine life and inadequate intake of calcium or phosphorus over an extended period of time during which the infant is growing rapidly.47 The cause is often multifactorial, including lower calcium and phosphorus stores, lower intake, prolonged use of total parenteral nutrition, especially calcium and phosphorus levels are low, and exposure to medications such as diuretics, anticonvulsants corticosteroids, and methylxanthines, and diuretics may contribute to metabolic bone disease in preterm infants.37,47,74,75 Factors predisposing to osteopenia in preterm infants are summarized in Figure 17-5.
Another factor may be the absence of bone loading in preterm infants who are hospitalized for prolonged periods.23,54 Bone loading normally occurs with passive and active exercise. Increased bone loading promotes bone formation; decreased bone loading leads to bone reabsorption.23 Most preterm infants with osteopenia have normal 25-(OH)D and 1,25-(OH)2D levels.23 Altered bone mineralization may continue into the preschool period and beyond and is associated with decreased linear growth.47,75,76
Rickets occurs because of a deficiency of calcium or phosphorus in extracellular fluid, usually associated with inadequate vitamin D, which ensures adequate intestinal absorption of these minerals (see Figure 17-5). This results in increased secretion of PTH, which stimulates osteoclastic breakdown and absorption of bone. Additional calcium is then available to maintain serum levels and prevent hypocalcemia. After a time the bone weakens and becomes stressed. Osteoblast activity is stimulated to replace the absorbed bone, leading to production of large amounts of osteoid (organic bone matrix) that never becomes completely calcified because of lack of calcium and phosphorus. Infants on total parenteral nutrition, with chronic health problems such as bronchopulmonary dysplasia, or on long-term diuretic therapy, have higher mineral requirements and are at greater risk for developing rickets. Acidosis increases urinary calcium losses and interferes with synthesis of 1,25-(OH)2D. Rickets has been reported in breastfed infants, but in most cases appears to be related more to lack of ultraviolet (sunlight) exposure of the mother than nutritional deficiencies per se. The greatest risks to the infant seem to occur if the mother’s diet is chronically deficient in vitamin D and she also does not get enough sun exposure (see Maternal-Fetal Interactions).46,47,75
Maturational changes during infancy and childhood
Age-related changes in calcium and phosphorus and their regulating hormones reflect needs of the infant and child to maintain calcium stability while providing for skeletal growth. Measures of bone turnover increase in the first few weeks, then stabilize from 4 to 10 weeks, and decrease after 10 weeks.10 Phosphorus levels decrease during the first year to 5 mg/dL (2.1 mmol/L) at 1-2 years, 4.4 mg/dL (1.8 mmol/L) by mid-childhood, and 3.5 mg/dL (1.5 mmol/L) by late adolescence.69 Reference ranges for serum calcium and phosphorus and urinary calcium excretion from birth to adolescence have been published.46 With increasing exposure to ultraviolet light, 25-(OH)D levels increase during this same period. Levels of 1,25-(OH)2D are higher than adult values for the first year. Levels of this biologically active metabolite may remain even higher in preterm infants for up to 3 months, perhaps to compensate for immature calcium absorption.23 Levels of 1,25-(OH)2D tend to be elevated during periods of growth. Serum magnesium levels tend to remain slightly higher than adult values in infants and young children.33
From 3 weeks to 6 months, serum calcium values in exclusively breastfed infants gradually increase, leading to a transient physiologic hypercalcemia.33 This increase may be related to decreased phosphorus intake due to the lower levels of phosphorus in human milk.75 Serum calcium levels do not change significantly in formula-fed infants from birth to 18 months. Serum calcium levels gradually decrease from 6 to 20 years. Intestinal absorption of calcium tends to be passive until after weaning. Absorption is mediated by high lactose levels that increase absorptive efficiency.27 Infants on standard formulas experience a progressive fall in serum parathyroid hormone (PTH) that reaches a nadir at about 3 months, at which time bone mineralization increases. This is analogous to the lowered PTH levels seen in utero and may promote extrauterine bone development. By 2 to 4 months, the renal response to exogenous PTH is similar to that seen in adults.27 Phosphate renal reabsorption decreases after weaning.69
Skeletal bone mass, size, shape, and properties continue to change throughout childhood and early adolescence and are affected by both genetic and environmental factors (such as diet, lifestyle, illness).18 During prepuberty, bone growth and mineralization are more rapid in the appendicular versus axial skeleton. Puberty stimulates rapid axial skeletal growth, which slows in later adolescence.18 The prepuberty period is characterized by a relative undermineralization that increases the risk of fractures. This is due to a lag of about 8 months between peak height velocity and peak mineral accrual.18
With the increased secretion of estrogens and other steroid hormones at menarche, skeletal mineral acquisition increases, stimulating longitudinal and radial skeletal growth for approximately 10 years, as well as increases in volumetric density.19 The first 4 years after menarche are characterized by a rapid increase in bone mineral density, followed by a slower increase in late adolescence and early adulthood.18 In women, peak bone mineral density is reached at 25-35 years (one third of this is achieved in the first 4 years after menarche).18
Summary
Calcium and phosphorus are essential minerals for many body processes and normal growth and development. Alterations in metabolic processes related to these elements can significantly alter the infant’s health status. Many of these problems can be prevented and normal growth and development promoted by careful assessment and monitoring of neonatal nutritional status and initiation of appropriate interventions. Recommendations for clinical practice related to calcium and phosphorus metabolism are summarized in Table 17-5.
Table 17-5
Recommendations for Clinical Practice Related to Calcium and Phosphorus Metabolism in Neonates
Know the usual changes in calcium, phosphorus, and magnesium metabolism during the neonatal period (pp. 598-600).
Monitor newborn calcium, phosphorus, and magnesium status during transition and in the early neonatal period (pp. 598-600).
Recognize factors that influence bone mineralization and growth (pp. 595-596, 597, 600-601).
Initiate early enteral feeding as appropriate (p. 600).
Monitor the nutritional intake of calcium, phosphorus, and vitamin D in infants (p. 600).
Monitor neonates for signs of excessive and inadequate intake of calcium, phosphorus, and magnesium (pp. 600-604).
Recognize infants at risk for hypocalcemia (pp. 601-603 and Table 17-4).
Know clinical signs of hypocalcemia (pp. 601-602).
Assess and monitor infants at risk for hypocalcemia (pp. 601-603).
Recognize infants at risk for hypomagnesemia and hypermagnesemia (p. 603).
Know the clinical signs of hypomagnesemia and hypermagnesemia (p. 603).
Assess and monitor infants at risk for hypomagnesemia and hypermagnesemia (p. 603).
Recognize and monitor infants at risk for late neonatal hypocalcemia (p. 603).
Recognize and monitor infants at risk for osteopenia and neonatal rickets (pp. 603-604).