Chapter 18 Bone Void Fillers
Bone and Bone Substitutes
One of the most common types of graft (second only to blood) is bone, with over 450,000 procedures using bone performed annually in the United States, and 2.2 million worldwide.1 Spine arthrodesis is the most common reason for autogenous bone harvest, with approximately 250,000 spinal fusions performed in the United States each year.2 Autogenous cancellous bone is the gold standard against which all other bone graft materials are compared. The osteogenic, osteoinductive, and osteoconductive properties of autograft are unequaled in stimulating bone repair. The procurement site of choice is the iliac crest because of the quantity and quality of available bone. Nevertheless, there are significant drawbacks to autograft, including procurement morbidity, limited availability, and increased operative time. In fact, iatrogenic complications originating from the graft procurement site represent a significant source of patient and physician concern. The primary operation may be successful, but the secondary procedure can result in increased patient recovery time and disability.3–6
By virtue of these drawbacks to both auto- and allograft, synthetic alternatives have been a very active area of research over the past 30 years. Nevertheless, only about 10% of the 2.2 million bone graft procedures annually performed worldwide involve synthetics, because of their perceived inferiority to native autograft and allograft.1 Drawbacks of many synthetics include poor resorbability, inclusion of animal or marine-derived components, variable handling characteristics, limited availability, and added cost. Until recently, synthetic grafts provided only osteoconductive properties, lacking osteoinductive and osteogenic potential. However, composite grafts that combine a synthetic osteoconductive matrix with osteoinductive growth factors and osteogenic cells have the potential to provide the advantages of autogenous bone graft—without its disadvantages. Numerous preclinical and clinical trials are under way to determine whether this potential can be realized.
Use of Cancellous Bone Grafts versus Substitutes
Role of Cancellous Bone
Cancellous bone can be considered a scaffold within which a variety of cell types interact to perform a wide array of essential functions, in addition to its importance as the nurturing microenvironment for hematopoiesis, myelogenesis, and platelet formation. Cancellous bone serves as an incubator that protects and grows the sources of its own maintenance and the renewal of pluripotent osteoprogenitor stem cells. The growth, migration, and differentiation of these bone-forming cells are regulated by local growth factors that are elaborated by the cells and platelets within the cancellous bone.7 In line with its role as a cell incubator, cancellous bone is highly porous and vascular. It demonstrates a limited weight-bearing function and is susceptible to collapse under compressive forces. Cortical bone surrounds and protects the cancellous bone. This dense structural material makes up the bulk of the skeleton and provides for its axial load-bearing capabilities.
General Characteristics of a Successful Bone Graft
Osteoinduction
Osteoinduction is the biologically mediated recruitment and differentiation of cell types essential for bone formation. Osteoinductive grafts supply factors that induce undifferentiated tissue to differentiate into bone.
Osteoconduction
Osteoconduction involves the apposition of growing bone to the three-dimensional surface of a suitable scaffold provided by the graft.8 Osteoconduction requires the structural and chemical environments that simulate those found in cancellous bone.9 The ideal scaffold provides dimensional stability and degrades at a rate commensurate with the speed of new bone formation.1
In addition, material for a successful bone graft must have good handling characteristics, be nontoxic (e.g., not leach chemicals into the circulation), and exhibit biomechanical characteristics (e.g., tension, compression, modules of elasticity) similar to those of cancellous bone. Spine surgeons currently are using a variety of materials, both stand-alone and in combination. Table 18-1 summarizes the biologic properties that constitute a graft’s osteointegrative capabilities (i.e., the formation of bony tissue around the implant without growth of fibrous tissue at the bone-implant interface).10,11
Graft Materials
Autograft
Pro
Autograft includes osteogenic bone and marrow cells as well as an osteoconductive matrix of cartilage, minerals, matrix proteins, and osteoinductive proteins associated with the matrix.12 Neither host rejection nor disease transmission is an issue with an autograft. The combination of these properties can result in high graft success rates. Many spinal fusion procedures (e.g., dorsal cervical. thoracic, and intervertebral) that use autogenous graft produce fusion rates higher than 90%.2
Con
Because the separation of body tissue from its blood supply results in cell death,2 the viability of autogenous bone as a living graft and host is severely compromised when it is harvested. Furthermore, the quality of the donor stock is not constant; it depends on many factors, such as the patient’s age, gender, health, and genetic disposition. Thus, the use of autograft does not always effect repair. This opens the door for alternatives. Although some spinal fusion procedures result in high fusion rates, the results are not uniform. Many common procedures, such as dorsolateral lumbar fusion, produce fusion rates as low as 56%.2,13 Although autogenous bone is regarded as the gold standard, its biologic performance is less than ideal.14
However, probably the greatest drawback to autograft use is the need for a second fascial incision and surgical dissection, with the attendant potential for complications.15 In fact, minor complications such as superficial infection, seroma/hematoma, temporary sensory loss, and mild or transient pain are common. Major complications occur at the donor site range in 0.7% to 39% of patients.2,16 These include infection, prolonged wound drainage, herniation of muscle and abdominal contents through the donor defect, deep hematomas, need for reoperation, pain lasting longer than 6 months, profound sensory loss, vascular and neurologic injury, unsightly scars, subluxation, gait disturbances, sacroiliac joint destabilization, enterocutaneous fistula, pelvic or iliac fracture, and heterotopic bone formation.17–19 Life-threatening complications include major vessel or visceral injury.
Neurologic injury may occur from dissection close to several nerves in the area (e.g., sciatic, lateral femoral cutaneous, and cluneal).6 Vascular injury to the superior gluteal vessels may occur from dissection too close to the sciatic notch. Chronic pain at the donor site, present in up to 25% of cases,20 may be attributable to excessive removal of bone from the sacroiliac region with violation of the sacroiliac joint.6
Hu and Bohlman6 reported a series of 14 patients who suffered a fracture at the iliac bone graft procurement site after spine fusion. Most of these patients were elderly women with chronic medical diseases. The authors, therefore, recommend iliac bone graft procurement with caution in this group to minimize the potential for these iatrogenic fractures. Based on subsequent cadaver studies, the authors recommend leaving at least 3 cm between the anterosuperior iliac crest and the graft procurement site21 and a maximum distance of 3 cm from the dorsal ilium.22
Although the risk of surgical complications theoretically can be minimized, certain procurement issues remain. These include increased operative time and blood loss, temporary disruption of donor-site bone structure, pain, vascular injuries, and cosmetic defects.12,20
Allograft
Allografts initially were used only for massive grafting where autograft use was impossible. However, by 1996 allografts constituted 34% of all bone grafts performed in the United States, an increase in use of more than 14-fold compared with just a decade earlier.14 Allograft has become the most common autograft substitute or extender for autograft.
Pro
Three factors have led to the surge in popularity of allograft.14 First, the National Organ Transplant Act increased overall availability. Second, donor screening and tissue processing have improved safety and quality of donated tissue. Third, the manufacture of new allograft forms (e.g., dowels) has greatly improved overall allograft utility and versatility. Perhaps the greatest advantage of allograft is its wide availability in a variety of physical forms that can be customized to specific applications. Machine tooling to shape structural allograft into forms such as wedges or threaded bone dowels can allow allograft to function as both bone graft and fixation device.2 Other advantages include the reduction of procurement morbidity, the potential for immediate structural support, and a reasonable success rate (>60%) reported for specific procedures (e.g., hip revision surgery, management of tumors in bone).23 Success rates for ventral-spinal lumbar fusions with allograft are comparable to those with autograft.24
Con
Allografts do not generate results equivalent to those of autografts.24 Allografts can vary greatly in initial bone quality, be of higher initial expense, transmit disease, and evoke immunogenic reactions.25 Processing constraints, required for patient safety, do not guarantee the absence of disease transmission or immunogenic reaction, but they do minimize risks posed by these adverse responses. One study of 1146 femoral heads considered suitable for bone-bank donation found unexpected disease in 8%, including three undiagnosed malignant bone tumors.26 Minimal processing of allograft (i.e., freezing freshly obtained bone) is not sufficient to inactivate the AIDS virus, as HIV transmission has been reported by this means.24
Processing renders the graft nonviable and mitigates osteoinduction potential by destroying proteins useful in recruiting bone cells and inducing new bone formation. Because the processed allografts are less representative of human tissue compared with autografts, allografts are not as readily received and incorporated by the host. Allografts are slower to be resorbed and not as completely replaced by new bone compared with autografts.24 The structural integrity of the processed bone complex also is compromised, and stability at the defect site, critical for rapid healing and return to function, is more difficult to achieve.2,27 Results are especially poor for dorsal lumbar fusion,24 and lower reported fusion rates for allograft implants compared with autograft-only implants were found in two studies.2
The quantity of allograft material is constrained by limited supply; tissue banks report difficulty with procurement because of fear of gross disfigurement at the donor site.28 Donor-to-donor variation results in uncertain, nonuniform quality.29 Bone quality varies with donor age and gender; even same-size bones from different anatomic sites in a single donor can vary in strength by as much as 20%.27
A low-grade inflammatory reaction typically is associated with allograft.25 This immune response may contribute to allograft failure (i.e., fracture and nonunion).24,30,31 Because of an initial intense inflammatory reaction, new capillaries are easily thrombosed, resulting in a delay in vascularization and osteoinduction.24 Even at maturation, necrotic bone can account for as much as 50% or more of the graft.24
A literature review of animal studies suggests a correlation between histocompatibility difference and allograft failure, both biologically and biomechanically.30 In a mouse model, the immunologic reaction appears to be specific to donor antigen and consists of killer/suppressor T cells, which are associated with soft tissue rejection.30 In humans, alloreactivity appears similar to the animal findings, resulting in an overall sensitization rate of 67%, higher than that seen after blood transfusion (12–50%).23,32 The immune response system may share common bone marrow-derived precursors and cytokines with the bone remodeling system, explaining the potential interaction of the immune response with bone remodeling.30 The most convincing evidence of a causal relationship between immunogenicity and poorer outcome is that among 29 patients studied who received allograft, those lacking sensitization to class II antigens achieved better clinical results than did sensitized patients.23
The two types of allograft in common use, fresh-frozen and freeze-dried, differ in their processing, which gives each different advantages and disadvantages. Fresh-frozen allografts retain BMP, are stronger and more completely incorporated in host bone than freeze-dried grafts,24 but also are the most immunogenic and have produced documented HIV transfer. Freeze-dried allograft is the least immunogenic and has caused no documented HIV or viral disease transmission. However, its BMP is destroyed, and it has the most compromised mechanical integrity, with decreased graft strength of up to 50% relative to freshly frozen allograft.2,27
Demineralized Bone Matrix
Demineralized bone matrix (DBM) is thought to possess more osteoinductive properties than regular allograft because of enhanced bioavailability of growth factors following the demineralization process.2,25 DBM gels and putties have become widely used in spinal fusion surgery since 1990, with about 500,000 mL used for implants each year in the United States.2 The first widely available DBM preparation was a gel consisting of DBM combined with a glycerol carrier. One retrospective study assessed the augmentation of local bone autograft with a DBM/glycerol composite for dorsolateral lumbar spine fusion as a means to avoid second-site autologous bone harvest. The control group used iliac crest autograft alone. The percentage of patients undergoing fusion was similar in both groups (60% and 56% for DBM and controls, respectively; P = .83).33 Although prospective clinical studies are under way, available data suggest a role for DBM as a bone-graft extender, rather than as a bone-graft substitute, in spinal surgery.2 Now there are several commercially available DBM substances for clinical use. Wang et al. studied the osteoinductibility of each DBM by comparing the usefulness of the different types of DBM as a bone graft substitute in an athymic rat spine fusion model. He reported that there are significant differences between some of the tested products, although all products claim to have significant osteoinductive capabilities. He noted that several factors such as differences in preprocess handling, varying demineralization times, final particle size, terminal sterilization, the differences in the carrier, and donor viability are expected to influence the properties of a DBM product. He also emphasized that a specific, sensitive, and reliable screening assay of the osteoinductive properties of DBM and objective information about each product’s osteoinductivity are much needed.34
Xenograft
Xenograft bone tissue is harvested from animals. Because of their immunogenicity, xenograft preparations generally have proven impractical for clinical use. Removal of proteinaceous and fatty materials during processing, as is done in the preparation of Kiel bone, Bio-Oss (Osteohealth, New York), or Oswestry bone, reduces immunogenicity to a degree.35 However, the processing required to produce this type of graft removes the osteoinductive matrix proteins. To guarantee viral inactivation, all such proteins must be removed. Processing strategies, such as freezing and freeze-drying, are less common than in the past because of unacceptable disease-transmission risk. Chemical washes have become more prevalent, but these tend to reduce or eliminate osteoinductivity.
Ceramics
Noninjectable Ceramics
Although they exhibit different chemical properties from tissue grafts, ceramics provide off-the-shelf availability of consistently high-quality synthetic materials that have no biologic hazards. After incorporation, the strength of the repaired defect site is comparable to that of cancellous bone.36 Therefore, ceramics can be used as an alternative or as an addition to either cancellous autograft or allograft37 or as a cancellous bone void filler or bone graft extender or in sites where compression is the dominant mode of mechanical loading.
In a randomized, prospective study of 341 patients undergoing dorsal spinal fusion for idiopathic scoliosis, patients received autograft or synthetic porous ceramic blocks (macroporous biphasic calcium phosphate [MBCP], Triosite, Zimmer, Inc., Warsaw, IN; a mixture of hydroxyapatite and tricalcium phosphate).38 Curve correction, curve maintenance, pain, and function were comparable between the two groups 18 months postoperatively. However, wound complications were more common in the autograft group—14 patients experienced delayed healing, infection, or hematoma compared with only 3 wound complications in the MBCP group. In addition, 15 autograft patients had pain at the donor site at 3 months. Other donor-site complications at 3 months included seven infections, two hematomas, and four cases of delayed healing. Histologic findings showed new bone incorporating into the MBCP—evidence of good osteoconduction. These results suggest that synthetic porous ceramic is a safe and effective substitute for iliac graft autograft in this patient population.
Another prospective study of 106 cases of lumbar spinal fusion used MBCP granules mixed with autogenous bone chips and bone marrow obtained from the local spine.39 Dorsal deformity correction using semi-rigid instrumentation was performed in all patients. Only six nonunions were observed (three resulting from primary spondylolisthesis), suggesting a high success rate for MBCP in spinal fusion involving a semi-rigid instrumentation. The authors conclude that because the degenerative spine is not favorable to fusion, this technique offers an alternative to autograft to reduce patient morbidity from iliac bone harvest.39
Rapidly Resorbing Ceramics
Scaffolds of tricalcium phosphate (the α and β forms have different crystalline structures but the same elemental and stoichiometric characteristics; the α form is formulated at 1200°C and the β form is formulated at 800°C) and calcium sulfate have been used as synthetic bone void fillers for more than 20 years.40,41 Calcium phosphate contains stoichiometric amounts of calcium and phosphorus, 39% and 20% by weight, respectively, similar to those found in natural bone.42 It produces calcium-phosphate–rich microenvironments that stimulate osteoclastic resorption and then osteoblastic new bone formation, resulting in new bone formation within the resorbed implant.43 Less porous formulations resorb before complete bone ingrowth is achieved.25 The rate of resorption and the porosity of several bone substitutes are presented in Tables 18-2 and 18-3.
Ceramic | Speed of Resorption |
---|---|
Hydroxyapatite | Slow |
β-Tricalcium phosphate | Intermediate |
α-Tricalcium phosphate | Rapid |
Calcium sulfate | Very rapid |
TABLE 18-3 Porosity and Osteoconductivity of Ceramics
Ceramic | Porosity/Osteoconductivity |
---|---|
Calcium phosphate | Very little |
Hydroxyapatite | Little |
α-Tricalcium phosphate | Intermediate |
β-Tricalcium phosphate | Very high |
Calcium sulfate (plaster of Paris) is available in pellet form. Although calcium sulfate is considered an osteoconductive bone graft substitute, its rapid resorption rate creates doubt about its ability to maintain a three-dimensional framework to support osteogenesis.44
Intermediate Resorbing Ceramics
β-Tricalcium phosphate (β-TCP) is one of the most commonly used bone graft alternatives. In the process of being resorbed, it can enrich the local environment with osteogenic substrates that, in turn, can be used by activated osteoblasts. Many highly porous β- TCP ceramics are commercially available. Currently they differ from previous β-TCP formulations in that they have a broad range of pore sizes (<1 μm to 1000 μm), and sponge-like interconnected microporosity endowed with excellent wicking and hydrophilic properties. These attributes facilitate the migration of bone-forming cells, growth factors, and phagocytic cells into it, enhancing the process of new bone development and its resorption.45
Slowly Resorbing Ceramics
Hydroxyapatite, another ceramic-based synthetic bone graft, can be formed into a three-dimensional structure that is rigid and stable. Controlling its pore diameter and the degree of pore interconnection can allow for rapid incorporation of new bone into it, which leads to a rigid mechanical bond between the implanted material and the host tissue. Although hydroxyapatite itself has some drawbacks, such as slow resorption and brittleness,46 a mixture of hydroxyapatite, β-TCP, and calcium carbonate can provide better osteoconduction for bone production, as well as long-term stability, leading to successful incorporation into a bone fusion mass.47
Thalgott et al. reported a 100% fusion rate using a hydroxyapatite with a pore diameter of 200 μm in combination with rigid plating for ventral cervical decompression and fusion.48
Injectable Ceramics: Calcium Phosphate Cement
Injectable calcium phosphate cement (CaP cement) is a paste of inorganic calcium and phosphate that hardens in situ with a low exothermic temperature, and cures by a crystallization reaction.44 It then slowly transforms into bone over 3 to 4 years. The resulting bone filler has a biologic response and compressive strength similar to that of cancellous bone, and has shown promise for some clinical applications such as use as an adjunct to fixation in both femoral neck and intertrochanteric hip fractures.44,49
The primary drawbacks to injectable calcium phosphate are slow resorption, low porosity, and high commercialization costs. Extravasation into surrounding soft tissues, or intra-articular extrusion also may occur, although these adverse events have been rarely reported.49
Many attempts recently have been made to apply CaP cements instead of polymethylmethacrylate (PMMA) in vertebral augmentation procedures. One clinical study using calcium phosphate cement reported resorption and fragmentation of the cement mantle. The authors noted two problems specific to calcium phosphate: an increased washout tendency and a lower flexural and shear resistance. They concluded that routine use of CaP cement is not currently recommended for vertebral augmentation.50
Collagen
Animal-derived collagen has been used with synthetic calcium phosphate bone fillers to modulate physical properties of bone-filling agents and to deliver factors that stimulate bone formation. The admixture of collagen imparts a putty-like consistency to the bone graft that facilitates handling and placement at the time of surgery. Various configurations are now available for clinical use.51 These products are designed to be hydrated and implanted with autogenous bone marrow or bone graft, and have been used as bone graft extenders to increase the volume of bone graft into a defect when a sufficient volume of autograft is not readily available.44
One formation of a type І collagen/hydroxyapatite matrix, prepared in 50- × 20- × 5-mm sheets, was studied in 50 cases of lumbar posterolateral spine fusion combined with bone marrow aspirate (BMA) as the graft material and achieved a 92% radiographic fusion rate.51 In contrast, an animal study reported that solid spinal fusion was not detected by manual palpation or on radiographs in any of the rabbits treated with the same collagen matrix combined with heparinized bone marrow.52
Nonbiologic Osteoconductive Substrates
The advantages of nonbiologic osteoconductive substrates include absolute control of the final structure, no immunogenicity, and excellent biocompatibility.43 Examples include degradable polymers,53 bioactive glasses, and porous metals such as tantalum.
Qualitative Assessment of Ceramics
A summary of the overall advantages and disadvantages of various bone graft materials is presented in Table 18-4. The table provides an overview of the clinical and economic aspects of the ceramics available today. These ceramics can perhaps be best judged by their resorption and porosity characteristics (see Tables 18-2 and 18-3). Of particular note in this regard is the importance of using a bone substitute that resorbs (and remodels) at a rate similar to that of cancellous bone (intermediate; see Table 18-2) and that is highly porous (see Table 18-3).
A canine study was undertaken to determine the rate of new bone ingrowth into defect sites repaired with either porous β-TCP synthetic cancellous bone void filler or hydroxyapatite-coated calcium carbonate.54 Cylindrical canine metaphyseal defects measured 10 × 25 mm in canine humeri. Bone ingrowth and scaffold resorption were quantified using standard histomorphometric techniques for specimens examined up to 1 year postimplantation.
As early as 3 weeks, β-TCP showed resorption (clearing) from the center outward and some immature bone formation (Fig. 18-1). At 6 weeks, the volume of new bone formed throughout the implants exceeded, by approximately 20%, the volume of original bone in areas adjacent to the defects (Fig. 18-2). Remodeling was essentially complete in 6 to 12 weeks, with bone density in the normal range and the scaffold almost completely resorbed by the end of the period. By 24 weeks, the trabecular orientation of the implant approximated the normal stress patterns of adjacent bone. Serial histologic and radiographic assessments made at 12, 24, and 52 weeks demonstrated comparable architecture and density between new bone within the defect sites and the adjacent original bone. The stress-strain curve of β-TCP was nearly identical to bone at 24 weeks and at 52 weeks (Fig. 18-3). In contrast, the calcium carbonate stress-strain curve differed markedly from bone at 24 weeks. Radiographic diffraction data of the β-TCP implants confirmed a 95% match to normal bone at 1 year.55
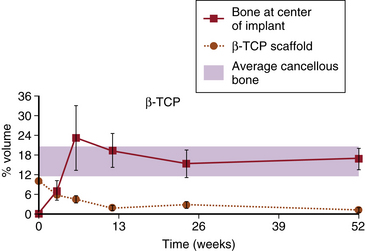
FIGURE 18-2 Rates of new bone ingrowth and resorption. β-TCP, β-tricalcium phosphate.
(Redrawn and modified from Erbe E, Clineff T, Lavagnino M, et al: Comparison of Vitoss™ and ProOsteone 50OR in a critical-sized defect at 1 year [abstract]. Presented at Annual Meeting of the Orthopaedic Research Society, February 25–28, 2001, San Francisco. Abstract 975.)
These results were superior in several respects to those of earlier, similar studies using other ceramics. Bruder et al.56 had found that a ceramic composed of hydroxyapatite and β-TCP produced no callus around the defect site or the host bone in the first 16 weeks after implantation, indicating inadequate bone healing. Both Bruder et al.56 and Kon et al.57 found that, using traditional synthetics, bone formation was most prevalent at the periphery of the defect. Bruder et al.56 noted that the only new bone that formed as a result of the osteoconductivity of the porous ceramic was an extension of the outgrowth from the cut ends of the host bone. In contrast, one of the striking observations about β-TCP is the initiation of resorption and osteogenesis at the center of the repaired defect site. In summary, Bruder et al.56 found that hydroxyapatite bone substitute was not ideal for the treatment of segmental defects because of its brittle nature, susceptibility to fracture, and lack of completely interconnected pores.
Biologic and Synthetic Composite Grafts
A composite graft can be defined as any combination of materials that includes both an osteoconductive matrix and an osteogenic or osteoinductive material. The carrier matrix could be any of the osteoconductive materials listed in Table 18-1. When the osteoconductive scaffold is mixed with bone marrow aspirate (BMA), the newly formed composite graft may acquire osteogenic and osteoinductive potential, thus providing a competitive alternative to autograft. Other biologic agents that may impart this potential include osteoblastic progenitor stem cells, blood, platelet-rich plasma, and osteoinductive growth factors such as BMP, transforming growth factor, and fibroblast growth factor.58
Osteogenic and Osteoinductive Bone Marrow Aspirate
Background
In theory, osteoblast progenitor cells could be obtained from the periosteum of long bones, the peritrabecular connective tissue, or the bone marrow, and combined with a synthetic scaffold.59 This strategy is designed to minimize the need for chemotaxis and massive proliferation of osteoblast progenitor cells into the defect. Logically, the direct implantation of progenitor cells should lead to more rapid, uniform, and reliable healing of bone defects. A major challenge to this approach is the need to identify more fully the proper type and source of cells for autologous cell therapy.56 However, for now, extensive clinical experience with bone marrow transplantation makes bone marrow a practical choice as a source of progenitor and osteoinductive cells.60
A number of studies have looked at BMA and its positive effects on bone formation both in vitro61 and in vivo.15,61,62 All of the critical cellular components that contribute to bone growth are present in BMA. Identifiable cell types include both fibroblasts and undifferentiated stromal cells. Osteoprogenitor stem cells are estimated to constitute 1 in 50,000 bone marrow cells in young patients and 1 in 2,000,000 cells in elderly patients. They are the most useful of bone tissue cells because they can differentiate into four other cell types (osteoblasts, adipose cells, chondroblasts, and fibroblasts) and modify their morphologic/functional attributes as needed.63 The mesenchymal stem cells have the potential to differentiate into a variety of tissues, including bone, cartilage, tendon, ligament, and adipose aggregations. Consequently, BMA is an abundant source of osteogenic cells for immediate transplantation. Animal research suggests that precursor cells in bone marrow proliferate and differentiate after transplantation. Among the cells derived from such proliferation are active osteoblasts that drive the process of new bone formation.60,64
Many investigators are engaged in characterizing the progenitor populations present in bone marrow, and in vitro studies of bone marrow–derived osteoblastic progenitors have helped define the potential role of the many growth factors involved in regulating osteoblast differentiation.7 Uncontrolled clinical trials suggest that mixtures of aspirated bone marrow and autograft may be effective in treating nonunions,5,65 but prospective trials using bone marrow alone have not been performed.66
Another advantage of BMA is its availability and the relative safety of its harvest. It is the only source that requires neither an open surgical procedure nor the added time and cost of in vitro cell growth.25 Cell progenitors derived from bone marrow can be harvested by aspiration from patients, with limited dilution by peripheral blood, as long as the volume of aspirate from a single site is held to 2 mL or less.60 Furthermore, the number of progenitors available in a graft site can be increased by concentration, if necessary, to further enhance the biologic result of bone grafting.67,68 For subsequent use in transplantation, stem cells also can be cultured and expanded to many times their original number.
Bone Marrow Aspirate and Bone Composites
Bone marrow has been used successfully to stimulate healing in tibial fractures, suggesting a promptly renewable and reliable source of osteogenic cells without the disadvantages of standard open-grafting techniques.17 In a study using human BMA taken from the femoral head and the iliac crest, the recovered cells demonstrated osteogenic potential in vivo in nude mice. The osteogenic potential was maintained as the cells were expanded in culture and enriched for grafting purposes.69 Autologous bone marrow aspirated from the iliac crest had a beneficial effect on osteogenesis in 25 of 28 patients when implanted with xenograft bone graft material (Kiel bone).70 The addition of bone marrow to autograft iliac crest bone graft facilitated greater bone formation and fusion success rates than did autograft alone (P < .05) in a rabbit dorsolateral spine fusion model.62 Furthermore, a study of 23 pediatric patients found that xenograft bone and other bone substitutes could be rendered osteogenic by combining these materials with fresh autologous bone marrow.71
Bone Marrow Aspirate and Synthetic Composites
A number of studies have assessed composites of bone marrow and synthetics for bone grafting.36,57,72 Ceramics of tricalcium phosphate, hydroxyapatite, and collagen hydroxyapatite were evaluated alone and with added BMA to assess their ability to heal defects created surgically in the canine radius. These implants also were compared with a graft of autogenous cancellous bone. The addition of BMA was essential for tricalcium phosphate and hydroxyapatite to achieve results comparable to those obtained with cancellous bone at 24 weeks.72
Studies on healing of canine femoral defects with implanted calcium phosphate cylinders loaded with cultured autologous mesenchymal stem cells showed that the addition of the cells augmented the development of new periosteal bone around the implants.56 Similarly, although porous hydroxyapatite bioceramic (HAC) alone was effective for repair of tibial defects (at 6 months), the introduction of bone marrow stromal cell populations into the composite resulted in far more extensive bone defect repair over a 2-month period.57 However, in both studies, even in the cell-loaded scaffold, a higher concentration of new bone began in the periphery. The authors speculate that this is the result of better survival of loaded cells within the outermost portions of the HAC cylinder, which probably are vascularized faster and more efficiently than more internal regions. They note that this may be related to the design of the implant device. The β-TCP animal study, which showed bone formation initiating within the center of the β-TCP carrier, supports this explanation. As early as 3 weeks after implantation, β-TCP showed resorption beginning from the center of the implant, perhaps because of the concentration of nutrients in the center.
The new generation of β-TCP implants brings unique biomechanical properties that make them particularly well suited for use with BMA in a composite graft. The broad range of interconnected porosity (1 μm to 1 mm) allows nutrient fluids to percolate throughout the structure and to support the migration of parenchyma into the scaffold, enhancing the processes of new bone development and scaffold resorption. The unique structure of ultraporous β-TCP is conducive, therefore, to rapid vascularization that is necessary for nourishing the seeded bone marrow, including bone-forming cells. In this respect, a β-TCP/BMA composite may be superior even to autograft, which suffers from anoxic cell death in the center of the graft because of the absence of vascularization (Fig. 18-4). One of the striking features of grafting with β-TCP compared with other synthetic constructs is the central pattern of bone formation, suggesting penetration of cells and nutrients.73
Thus, a composite made up of ultraporous β-TCP seeded with aspirated autologous bone marrow could deliver many of the positive qualities of an autograft and avoid most of the negative ones. The β-TCP scaffold supplies an osteoconductive surface for bone and tissue ingrowth. Ultraporosity facilitates infusion of bone matrix proteins and growth factors, making the β-TCP osteoinductive.74 Local and recruited osteogenic cells penetrate the composite and interact with the seeded bone-forming cells to impart osteogenic properties to β-TCP (Fig. 18-5). β-TCP seeded with BMA therefore can provide all three properties necessary for a successful bone graft that was previously satisfied by autografts, namely, osteogenesis, osteoinduction, and osteoconduction.
Bone Morphogenetic Protein and Synthetic Composites
Human recombinant bone morphogenetic proteins, including recombinant human BMP-2 (rhBMP-2) and recombinant human osteogenic protein 1 (rhOP-1 or rhBMP-7), are being investigated in human clinical trials and show promise as autologous bone graft substitutes.47,75–77 A carrier that can maintain optimal regional concentrations of BMP over a prolonged period is essential for the efficient utilization of BMPs. To date, several biomaterials have been used as carriers for BMP.46 Of these, hydroxyapatite (HAp) is considered particularly useful, because of its high affinity with BMP. To further enhance their osteogenic potential, HAp and other ceramics are now routinely combined with various active biologic substances.47
In Boden’s clinical trial, 25 patients underwent posterolateral lumbar spine fusion using 20 mg of rhBMP-2 with a carrier consisting of 60% hydroxyapatite and 40% tricalcium phosphate granules, resulting in a 100% fusion rate, although some patients did smoke during the postoperative period.66 Dawson performed a clinical study to investigate the use of rhBMP-2 on an absorbable collagen sponge combined with a ceramic-granule bulking agent as a bone graft substitute in single-level posterolateral lumbar spinal fusion, and achieved an 88% fusion rate.78 Human clinical trials also have shown the efficacy of OP-1 as both an alternative and an enhancer to autologous bone graft or spinal fusion. OP-1 putty (3.5 mg rhOP-1 to 1 g bovine bone collagen to 230 mg carboxy methyl cellulose) is now approved for use as a carrier.79
The delivery systems currently available for recombinant BMPs include DBM, synthetic polymers, type 1 collagen hyaluronic acid gels, and a variety of bone graft substitutes. However, Crawford et al. reported the higher incidence of dorsal cervical wound complications with rhBMP-2 on an absorbable collagen sponge, and emphasized the importance of determining optimal dosing and carrier to achieve the desired results of fusion.80 An alternate approach that may allow BMPs to produce even greater therapeutic effects may be attained in the future with gene therapy, which provides for the transfer of genetic information to a host cell that would express and produce endogenous BMP protein.75
Summary
After blood, bone is the most commonly transplanted tissue. Autogenous bone grafting is one of the most common orthopaedic procedures, performed in about 200,000 cases annually in the United States.81 Although materials of biologic origin are now generally used, their preference may diminish as well-characterized inorganic materials with off-the-shelf availability offer potential to eliminate procurement morbidity associated with autograft and to eliminate risk of disease transmission associated with allograft.
However, the development of a usable osteoconductive carrier has lagged behind the isolation and synthesis of osteoinductive growth factors. Preclinical data indicate that the enhanced capillarity of ceramics such as β-TCP may bring these bone-healing elements together in better balance. Advances in tissue engineering of synthetic composite grafts should demonstrate a synergy between components with results superior even to autogenous bone grafts.82 The improved understanding of osteoprogenitor cell function, and advances in procurement and cell separation, will provide for increased osteogenic capabilities with negligible harvest morbidity. Finally, BMPs and synthetic composites show significant promise in the arenas of bone healing and bone fusion. Further clinical information verifying the utility of BMA/bone composites, BMPs, and synthetic composites will be available in the near future.
Boden S.D., Kang J., Sandhu H., Heller J.G. Use of recombinant human bone morphogenetic protein-2 to achieve posterolateral lumbar spine fusion in humans: a prospective, randomized clinical pilot trial: 2002 Volvo Award in clinical studies. Spine. 2002;27:2662-2673.
Connolly J., Guse R., Lippiello L., Dehne R. Development of an osteogenic bone-marrow preparation. J Bone Joint Surg [Am]. 1989;71:684-691.
Fernyhough J.C., Schimandle J.J., Weigel M.C., et al. Chronic donor site pain complicating bone graft harvesting from the posterior iliac crest for spinal fusion. Spine. 1992;17(12):1474-1480.
Fleming J.E.Jr., Cornell C.N., Muschier G.F. Bone cells and matrices in orthopedic tissue engineering. Orthop Clin North Am. 2000;31:357-374.
Heary R.F., Schlenk R.P., Sacchieri T.A., et al. Persistent iliac crest donor site plan: independent outcome assessment. Neurosurgery. 2002;50(3):510-516.
Ludwig S.C., Boden S.D. Osteoinductive bone graft substitutes for spinal fusion: a basic science summary. Orthop Clin North Am. 1999;30:635-645.
Muschler G.F., Boehm C., Easley K. Aspiration to obtain osteoblast progenitor cells from human bone marrow: the influence of aspiration volume. J Bone Joint Surg [Am]. 1997;79:1699-1709.
Wang J.C., Alanay A., Mark D., et al. A comparison of commercially available demineralized bone matrix for spinal fusion. Eur Spine J. 2007;16:1233-1240.
1. Lewandrowski K.U., Gresser J.D., Wise D.L., Trantolo D.J. Bioresorbable bone graft substitutes of different osteoconductivities: a histologic evaluation of osteointegration of poly(propylene glycol-cofumaric acid)-based cement implants in rats. Biomaterials. 2000;21:757-764.
2. Sandhu H.S., Grewal H.S., Parvataneni H. Bone grafting for spinal fusion. Orthop Clin North Am. 1999;30:685-698.
3. Fernyhough J.C., Schimandle J.J., Weigel M.C., et al. Chronic donor site pain complicating bone graft harvesting from the posterior iliac crest for spinal fusion. Spine. 1992;17(12):1474-1480.
4. Gupta A.R., Shah N.R. Patel TCh, Grauer JN: Perioperative and long-term complications of iliac crest bone graft harvesting for spinal surgery: a quantitative review of the literature. Int Med J. 2001;8(3):163-166.
5. Heary R.F., Schlenk R.P., Sacchieri T.A., et al. Persistent iliac crest donor site plan: independent outcome assessment. Neurosurgery. March 2002;50(3):510-516.
6. Hu R.W., Bohlman H.H. Fracture at the iliac bone graft harvest site after fusion of the spine. Clin Orthop. 1994;309:208-213.
7. Lowery G.L., Kulkarni S., Pennisi A.E. Use of autologous growth factors in lumbar spinal fusion. Bone. 1999;25(suppl):47S-50S.
8. Bauer T.W., Muschier G.F. Bone graft materials: an overview of the basic science. Clin Orthop. 2000;371:10-27.
9. Cornell C.N., Lane J.M. Current understanding of osteoconduction in bone regeneration. Clin Orthop. 1998;7(355S):S267-S273.
10. Higuchi K.W. Osseointegration or osteointegration? [letter]. Oral Surg Oral Med Oral Pathol Oral Radiol Endod. 2000;89:132.
11. Siegel M.A. Osseointegration or osteointegration? [letter]. Oral Surg Oral Med Oral Pathol Oral Radiol Endod. 2000;89:132.
12. Arrington E.D., Smith W.J., Chambers H.G., et al. Complications of iliac crest bone graft harvesting. Clin Orthop. 1996;329:300-309.
13. Steinmann J.C., Herkowitz H.N. Pseudarthrosis of the spine. Clin Orthop. 1992;284:80-90.
14. Boyce T., Edwards J., Scarborough N. Allograft bone: the influence of processing on safety and performance. Orthop Clin North Am. 1999;30:571-581.
15. Truumees E., Herkowitz H.N. Alternatives to autologous bone harvest in spine surgery. Univ Penn Orthop J. 1999;12:77-88.
16. Sasso R.C., LeHuec J.C., Shaffrey C. The Spine Interbody Research Group: iliac crest bone graft donor site pain after anterior lumbar interbody fusion. J Spinal Disord Tech. 2005;18(Suppl 1):S77-S81.
17. Connolly J., Guse R., Lippiello L., Dehne R. Development of an osteogenic bone-marrow preparation. J Bone Joint Surg [Am]. 1989;71:684-691.
18. Dosoglu M., Orakdogen M., Tevruz M., et al. Enterocutaneous fistula: a complication of posterior iliac bone graft harvesting not previously described. Acta Neurochir (Wien). 1998;140:1089-1092.
19. Fernando T.L., Kim S.S., Mohler D.G. Complete pelvic ring failure after posterior iliac bone graft harvesting. Spine. 1999;24:2101-2104.
20. Summers B.N., Eisenstein S.M. Donor site pain from the ilium: a complication of lumbar spine fusion. J Bone Joint Surg [Br]. 1989;71:677-680.
21. Hu R., Hearn T., Yang J. Bone graft harvest site as a determinant of iliac crest strength. Clin Orthop. 1995;310:252-256.
22. Varga E., Hu R., Hearn T.C., et al. Biomechanical analysis of hemipelvic deformation after corticospongious bone graft harvest from the posterior iliac crest. Spine. 1996;21:1494-1499.
23. Friedlaender G.E., Strong D.M., Tomford W.W., Mankin H.J. Long-term follow-up of patients with osteochondral allografts: a correlation between immunologic responses and clinical outcome. Orthop Clin North Am. 1999;30:583-588.
24. Ehrler D.M., Vaccaro A.R. The use of allograft bone in lumbar spine surgery. Clin Orthop. 2000;371:38-45.
25. Fleming J.E.Jr., Cornell C.N., Muschier G.F. Bone cells and matrices in orthopedic tissue engineering. Orthop Clin North Am. 2000;31:357-374.
26. Palmer S.H., Gibbons C.L., Athanasou N.A. The pathology of bone allograft. J Bone Joint Surg [Br]. 1999;81:333-335.
27. Pelker R.R., Friediaender G.E. Biomechanical aspects of bone autografts and allografts. Orthop Clin North Am. 1987;18:235-239.
28. Carter G. Harvesting and implanting allograft bone. AORN J. 1999;70:660-670.
29. Henman P., Finlayson D. Ordering allograft by weight: suggestions for the efficient use of frozen bone-graft for impaction grafting. J Arthroplasty. 2000;15:368-371.
30. Friedlaender G.E. Bone allografts: the biological consequences of immunological events. J Bone Joint Surg [Am]. 1991;73:1119-1122.
31. Goldberg V.M., Powell A., Shaffer J.W., et al. Bone grafting: role of histocompatibility in transplantation. J Orthop Res. 1985;3:389-404.
32. Strong D.M., Friedlaender G.E., Tomford W.W., et al. Immunologic responses in human recipients of osseous and osteochondral allografts. Clin Orthop. 1996;326:107-114.
33. Sassard W.R., Eidman D.K., Gray P.M., et al. Augmenting local bone with Grafton demineralized bone matrix for posterolateral lumbar spine fusion: avoiding second site autologous bone harvest. Orthopedics. 2000;23:1059-1065.
34. Wang J.C., Alanay A., Mark D., et al. A comparison of commercially available demineralized bone matrix for spinal fusion. Eur Spine J. 2007;16:1233-1240.
35. Elves M.W., Salama R. A study of the development of cytotoxic antibodies produced in recipients of xenografts (heterografts) of iliac bone. J Bone Joint Surg [Br]. 1974;56:331-339.
36. Gazdag A.R., Lane J.M., Glaser D., Forster R.A. Alternatives to autogenous bone graft: efficacy and indications. J Am Acad Orthop Surg. 1995;3:1-8.
37. Browner B.D., Jupiter J.B., Levine A.M., et al, editors. Skeletal trauma: fractures, dislocations, ligamentous injuries, ed 2, vol 2. Philadelphia: WB Saunders, 1998.
38. Ransford A.O., Morley T., Edgar M.A., et al. Synthetic porous ceramic compared with autograft in scoliosis surgery: a prospective, randomized study of 341 patients [published erratum appears in J Bone Joint Surg [Br] 80(3):562, 1998]. J Bone Joint Surg [Br]. 1998;80:13-18.
39. Cavagna R., Daculsi G., Bouler J.M. Macroporous calcium phosphate ceramic: a prospective study of 106 cases in lumbar spinal fusion. J Long Term Eff Med Implants. 1999;9:403-412.
40. Doursounian L., Cazeau C., Touzard R.C. Use of tricalcium phosphate ceramics in tibial plateau fracture repair: results of 15 cases reviewed at 38 months. Available at http://bhd.online.fr/framesus.htm. Accessed Sept 10, 2003
41. McAndrew M.P., Gorman P.W., Lange T.A. Tricalcium phosphate as a bone graft substitute in trauma: a preliminary report. J Orthop Trauma. 1989;2:333-339.
42. LeGeros R.Z. Calcium phosphates in oral biology and medicine. Monogr Oral Sci. 1991;15:1-201.
43. Cornell C.N. Osteoconductive materials and their role as substitutes for autogenous bone grafts. Orthop Clin North Am. 1999;30:591-598.
44. Hak D.J. The use of osteoconductive bone graft substitutes in orthopaedic trauma. J Am Acad Orthop Surg. 2007;15:525-536.
45. Damron T.A. Use of 3D β-tricalcium phosphate (Vitoss®) scaffolds in repairing bone defects. Nanomedicine. 2007;2:763-775.
46. Morisue H., Matsumoto M., Chiba K., et al. Novel hydroxyapatite fiber mesh as a carrier for recombinant human bone morphogenetic protein-2 enhances bone union in rat posterolateral fusion model. Spine. 2006;31:1194-1200.
47. Brandoff J.F., Siber J.S., Vaccaro A.R. Contemporary alternatives to synthetic bone grafts for spine surgery. Am J Orthop. 2008;37:410-414.
48. Thalgott J.S., Fritts K., Giuffre J.M., Timlin M. Anterior interbody fusion of the cervical spine with coralline hydroxyapatite. Spine (Phila Pa 1976). 1999;24(13):1295-1299.
49. Larsson S., Bauer T.W. Use of injectable calcium phosphate cement for fracture fixation: a review. Clin Orthop. 2002;395:23-32.
50. Blattert T.R., Jestaedt L., Weckback A. Suitability of a calcium phosphate cement in osteoporotic vertebral body fracture augmentation. Spine. 2009;34:108-114.
51. Neen D., Noyes D., Shaw M., et al. Healos and bone marrow aspirate used for lumbar spine fusion. Spine. 2006;31:E636-E640.
52. Kraiwattanapong C., Boden S.D., Louis-Ugbo J., et al. Comparison of Healos/bone marrow to INFUSE(rhBMP-2/ACS) with a collagen-ceramic sponge bulking agent as graft substitutes for lumbar spine fusion. Spine. 2005;30:1001-1007.
53. Bostman O., Hirvensalo E., Makinen J., Rokkanen P. Foreign-body reactions to fracture fixation implants of biodegradable synthetic polymers. J Bone Joint Surg [Br]. 1990;72:592-596.
54. Erbe E., Clineff T., Lavagnino M., et al. Comparison of Vitoss™ and ProOsteone 50OR in a critical-sized defect at 1 year [abstract]. San Francisco: Presented at Annual Meeting of the Orthopaedic Research Society; February 25-28, 2001. Abstract 975
55. Marx J., Erbe E., Mesropian C. Structural evolution of Vitoss™ scaffold to bone in canine humeral defect at 1 year (abstract). San Francisco, CA: Presented at Annual Meeting of the Orthopaedic Research Society; February 25-28, 2001.
56. Bruder S.P., Kraus K.H., Goldberg V.M., Kadiyala S. The effect of implants loaded with autologous mesenchymal stem cells on the healing of canine segmental bone defects. J Bone Joint Surg [Am]. 1998;80:985-996.
57. Kon E., Muraglia A., Corsi A., et al. Autologous bone marrow stromal cells loaded onto porous hydroxyapatite ceramic accelerate bone repair in critical-size defects of sheep long bones. J Biomed Mater Res. 2000;49:328-337.
58. Ludwig S.C., Boden S.D. Osteoinductive bone graft substitutes for spinal fusion: a basic science summary. Orthop Clin North Am. 1999;30:635-645.
59. Garg N.K., Gaur S. Percutaneous autogenous bone-marrow grafting in congenital tibial pseudarthrosis. J Bone Joint Surg [Br]. 1995;77:830-831.
60. Muschler G.F., Boehm C., Easley K. Aspiration to obtain osteoblast progenitor cells from human bone marrow: the influence of aspiration volume. J Bone Joint Surg [Am]. 1997;79:1699-1709.
61. Majors A.K., Boehm C.A., Nitto H., et al. Characterization of human bone marrow stromal cells with respect to osteoblastic differentiation. J Orthop Res. 1997;15:546-557.
62. Curylo L.J., Johnstone B., Petersilge C.A., et al. Augmentation of spinal arthrodesis with autologous bone marrow in a rabbit posterolateral spine fusion model. Spine. 1999;24:434-438.
63. Anonymous Bone. Ross M.H., Romrell L.J., Kaye G.I., editors. Histology: a text and atlas. Baltimore: Williams & Wilkins. 1995:150-187.
64. Friedenstein A.J., Petrakova K.V., Kurolesova A.L., Frolova G.P. Heterotopic transplants of bone marrow. Transplantation. 1968;6:230-247.
65. Connolly J.F., Guse R., Tiedeman J., Dehne R. Autologous marrow injection as a substitute for operative grafting of tibial nonunions. Clin Orthop. 1991;266:259-270.
66. Boden S.D., Kang J., Sandhu H., Heller J.G. Use of recombinant human bone morphogenetic protein-2 to achieve posterolateral lumbar spine fusion in humans: a prospective, randomized clinical pilot trial: 2002 Volvo Award in clinical studies. Spine. 2002;27:2662-2673.
67. Kadilaya S, Lo H, Leong KVV, et al: Biodegradable polymers and synthetic bone graft in bone formation and repair. 1994. Presented at American Academy Orthopaedic Surgeons Symposium, Park Ridge, IL 11:317–324, 1994
68. Krzymanski G., Kalozak M., Wiktor-Jedrzejczak W. The use of bone-marrow-derived fibroblastoid cells and fresh bone marrow in the treatment of bone defects: an experimental study. Int J Oral Maxillofac Surg. 1997;26:55-60.
69. Haynesworth S.E., Goshima J., Goldberg V.M., Caplan A.L. Characterization of cells with osteogenic potential from human marrow. Bone. 1992;13:81-88.
70. Salama R., Weissman S.L. The clinical use of combined xenografts of bone and autologous red marrow: a preliminary report. J Bone Joint Surg [Br]. 1978;60:111-115.
71. Wientroub S., Goodwin D., Khermosh O., Salama R. The clinical use of autologous marrow to improve osteogenic potential of bone grafts in pediatric orthopedics. J Pediatr Orthop. 1989;9(2):186-190.
72. Johnson K.D., Frierson K.E., Keller T.S., et al. Porous ceramics as bone graft substitutes in long bone defects: a biomechanical, histological, and radiographic analysis. J Orthop Res. 1996;14:351-369.
73. Erbe E.M., Marx J.G., Clineff T.D., Bellincampi L.D. Potential of an ultraporous ß-tricalcium phosphate synthetic cancellous bone void filler and bone marrow aspirate composite graft. Eur Spine J. 2001;10:S141-S146.
74. Anker C.J., Holdridge S.p., Baird B., et al. Ultraporous ß-tricalcium phosphate is well incorporated in small cavitary defects. Clin Orthop. 2005;434:251-257.
75. Carlisle E., Fischgrund J.S. Bone morphogenetic proteins for spinal fusion. Spine J. 2005;5:S240-S249.
76. Mummaneni P.V., Haid R.W., Rodts G.E. Contribution of recombinant human bone morphogenetic protein-2 to the rapid creation of interbody fusion when used in transforaminal lumbar interbody fusion: a preliminary report. J Neurosurg Spine. 2004;1:19-23.
77. Muschler G.F., Hyodo A., Manning T., et al. Evaluation of human bone morphogenetic protein 2 in a canine spinal fusion model. Clin Orthop. 1994;308:229-240.
78. Dawson E., Bae H.W., Burkus J.K., et al. Recombinant human bone morphogenetic protein-2 on an absorbable collagen sponge with an osteoconductive bulking agent in posterolateral arthrodesis with instrumentation. J Bone Joint Surg [Am]. 2009;91:1604-1613.
79. Vaccaro A.R., Lawrence J.P., Patel T., et al. The safety and efficacy of OP-1(rhBMP-7) as a replacement for iliac crest autograft in posterolateral lumbar arthrodesis. Spine. 2008;33:2850-2862.
80. Crawford C.H., Carreon L.Y., McGinnis M.D., et al. Perioperative complications of recombinant human bone morphogenetic protein-2 on an absorbable collagen sponge versus iliac crest bone graft for posterior cervical arthrodesis. Spine. 2009;34:1390-1394.
81. Van Heest A., Swiontkowski M. Bone-graft substitutes. Lancet. 1999;353(suppl I):28-29.
82. Lane J.M., Tomin E., Bostrom M.P. Biosynthetic bone grafting. Clin Orthop. 1999;367S:S107-S117.