Chapter 2 Basic Principles in Flap Reconstruction
Reconstruction of wounds has long been a challenge to surgeons. Whereas some wounds may be closed in a simple linear fashion, others require complex movement of local or distant tissue to restore functional and anatomic relationships and to optimize the cosmetic outcome. Wound management considerations must always include healing by second intention, primary approximation, grafts, and flaps.
Surgeons have used skin flaps to repair wound defects for centuries. The term “flap” was derived from the Dutch word “flappe” during the sixteenth century. “Flappe” referred to something that was fastened by one side and hung broad and loose. As early as 700bce, Sushrata Samita first documented his technique of reconstructing a large nasal tip defect with a cheek flap.1 Since that time, surgeons’ knowledge of biology, anatomy, and physiology has greatly increased. This understanding allows us to significantly decrease the likelihood of surgical complications such as flap failure. This chapter addresses some basic principles of flap reconstruction including flap design, construction, and classification. The authors’ experience with flap reconstruction of facial wounds will be utilized to emphasize the particular advantages of flap procedures.
BASIC TERMINOLOGY
A skin flap is a construct of skin and subcutaneous tissue with a partially intact vascular supply that is relocated or repositioned from a donor site to a recipient site. The recipient site is called the primary defect and, in dermatologic surgery, is usually the wound resulting from the removal of a tumor (Figure 2.1). The secondary defect is defined as the wound that is created by cutting, lifting, and sliding the flap to fill the primary defect (Figure 2.1). The base of the flap is that area that remains attached to the skin adjacent to the defect. It is this base, also known as the pedicle of the flap, which contains the vascular supply necessary for initial flap survival. The tip of the flap is the portion of the flap furthest from the flap’s base.
FLAPS DEFINED BY BLOOD SUPPLY
Blood flow is obviously the most crucial factor for flap viability. Vascular perfusion pressure is a description of the force of blood flow through a vessel. Perfusion pressure and blood flow in the body’s vessels are analogous to water flowing from a faucet through a lawn hose. The greatest pressure and highest flow will be at the faucet head. As water travels away from the faucet, its pressure and flow decrease in strength. To maintain sufficient flow to the distal tip of the hose, the perfusion pressure within the hose must exceed the resistance of the hose. Similarly in the human body, the perfusion pressure of any vessels that supplies the skin must exceed the capillary resistance in order to maintain vessel patency and preserve continued blood flow. A critical pressure is required to maintain patency of capillaries. Below this certain pressure, the capillaries will close and insufficient blood will be supplied to the distal portions of the flap. The greater the distance from the feeding artery or arteriole, the lower the perfusion pressure will be (Figure 2.2). Thus, beyond a certain distance, the flap tip will no longer receive blood nutrients, and it will thus necrose.2,5
Perfusion pressures have dictated that flaps should be designed with appropriate flap length to pedicle width ratios. Until 1970, it was widely believed that the viable length of a flap was directly proportional to the width of the base. Surgeons believed that in order to double the length of the flap one would simply need to double the width of the flap base, thereby including a sufficient number of vessels in the pedicle to sustain the flap tip. Many felt that there was no ultimate limit to flap length. In 1970, Milton disputed this conventional hypothesis by publishing his research on axial flaps in the pig model. He discovered that “flaps made under the same conditions of blood supply survive to the same length regardless of width.”3 Daniel and Williams confirmed Milton’s research on axial flaps and further studied survival of random pattern flaps. Although they concluded that “an increase in width did not result in an increased length of survival,”4 their data showed a trend toward wider pedicles allowing greater flap length survival. Clinical experience with random flap survival echoed this survival trend.
In 1979, Stell conducted further studies utilizing the pig model and extrapolated his findings to humans. His research supported the traditional hypothesis that the viable length of a flap is indeed dictated by its width, but there is an upper limit of length survival that cannot be increased by increasing the pedicle width.5 Also contrary to the hypothesis, viable flap length was not directly proportional to pedicle width. Instead, viable length appeared to be predicted with the formula [67.3 – (121.4/√Width)]. In his paper, Stell extrapolated his findings in the pig model to humans using a formula based on aorta size and surface area. By multiplying the maximum surviving random flap length on the pig abdomen by a factor of 2.25, he predicted a maximum random flap length of 12.5 cm on the human abdomen. Clinical experiences with random flaps on the human abdomen are similar to this predicted measurement. Beyond a certain pedicle width, greater recruitment of random vessels cannot support the flap tip (Figure 2.2). Overall, perfusion pressure limits the ultimate length of both axial and random flaps.
The greater the perfusion pressure in the flap’s pedicle, the longer the flap can be without undergoing necrosis. Moreover, the greater the perfusion pressure at the flap’s base, the more narrow the pedicle may be. Arteries always have greater perfusion pressures than distal arterioles and capillaries. Accordingly, the longest viable flaps with the narrowest bases (largest length-to-base ratios) are arterial flaps. Stell5 found that the greatest length of a viable axial flap was approximately 60% greater than that of a random flap utilizing the same pedicle width. Since flap survival is based on blood supply,5–7 it was apparent that axial flaps are better perfused. Therefore, a paramedian forehead flap that utilizes the supratrochlear artery may be at least four times as long as the flap’s pedicle width (at least a 4:1 ratio) (Figure 2.3). Musculocutaneous flaps have the next greatest blood supply in the pedicle, followed by fasciocutaneous and random flaps in descending order.
The commonly utilized random pattern flaps are largely supported by the redundant subdermal vascular plexus in the skin. Figure 2.4 highlights the innate vasculature of the skin upon which flap survival depends. The deeper, muscular-based arteries supply the subdermal plexus, which subsequently perfuses the intradermal plexus. The intradermal vasculature alone is usually unable to support tissue viability due to low perfusion pressures and blood flow within these distal capillaries.2,8 However, the subdermal plexus, found in the mid to superficial subcutaneous fat, contains both arterioles and capillaries with sufficient perfusion pressure to sustain tissue viability following flap movement. This anatomy is critical to understand when undermining (dissecting under the flap and its surrounding area to allow tissue movement) a cutaneous flap. If undermining is performed superficial to the subdermal plexus (ie, within the dermis), the flap has a significantly increased chance of undergoing tissue ischemia.
The perfusion pressures of random cutaneous flaps vary with the flaps’ locations on the body.3–5,7,9–13 As soon as a random pattern flap is incised and raised, there is a significant decrease in the perfusion pressure to the distal, disrupted skin.14,15 Fortunately at normal skin temperature, the amount of blood flowing through the facial skin is ten times greater than that needed to supply the skin’s basic metabolic needs.16–19 Other areas of the body are not as well supplied with this redundancy of blood supply. A general rule is that the more distant the surgical flap is located from the heart, the less the perfusion pressure will be. Hence, a flap on the leg should be designed with a smaller flap length-to-pedicle width ratio when compared to a flap on the face.
There is typically a maximum length-to-width ratio for any random cutaneous flap that will generally allow adequate flap perfusion. In general, the longer the required flap, the wider the pedicle must be to ensure flap survival. With a wider flap base, there are a greater number of subdermal vessels employed to supply the most distal periphery of the flap. However, one must remember that these vessels at the pedicle of the random flap all share a common perfusion pressure. As previously discussed, there is a point at which no matter how many extra vessels are recruited at the base, the distal perfusion pressure will be less than the critical closing pressure of the arterioles and capillaries (Figure 2.2).3,5,7,15,20 When the perfusion pressure is less than this intravascular resistance, the flap receives diminished nutrients and necrosis can ensue. Generally, random cutaneous flaps of the face with a maximum length-to-base ratio of 3:1 survive.21–23 On the trunk and legs, the maximum length-to-base ratio is considered to be 2:1.12,21,22,24,25 An axial flap on the face may have a 4:1 or greater ratio depending on the arterial supply of the pedicle.21,60 Remember that these ratios are only guidelines. Patient factors such as tobacco abuse, medical comorbidities, and a history of radiation therapy or prior surgeries in the area will all influence local perfusion pressures and the maximum safe length-to-width ratios of the flaps.
FLAP PHYSIOLOGY
Flap survival is dependent on various factors including blood flow, angiogenesis and vascularization, edema, wound closure tension, and infection. Prior to the first incision, the flap skin is fully vascularized and viable using the definition of normal skin. Once the flap is raised, it is always ischemic since the normal vessels supplying that skin are cut and the flap now depends on decreased circulation from the collateral vessels. A flap is always initially viable since the skin can survive up to 12 to 13 hours of avascularity at 37°F.26–28 An ischemic flap can survive even longer since the blood flow needed to sustain skin is only 2 to 8 cc per 100 gm per minute, and normal flow to the skin is ten times greater than this minimum.16,26 Thus, Meyers26 was correct when he commented that a fresh flap is always ischemic, but viable. Once incised and relocated, the nascent flap receives its nutrients from both the pedicle and the base of the primary defect. Sufficient blood flow through the base of the flap is essential in the initial 24 to 48 hours following the initial flap creation. In both axial and random flaps, blood flow immediately drops as the flap is elevated. For axial flaps, microvascular flow actually increases to a level greater than the preoperative state within 5 hours.15,29 Flow in random pattern flaps, however, starts to improve differen-tially for up to four weeks. Marks utilized the rat model to show that flow improved on a gradient; flow increased within 14–16 hours to the skin closest to the pedicle, within 24–48 hours to the skin 1 cm distal to the pedicle, and 96 hours 3 cm distal to the pedicle.15 All sites recovered approximately 30% of their blood flow per day, with the proximal most portion recovering full blood flow by the end of 7 days and the most distal tip by the end of 14 days. Until 14 days, recovery of blood flow occurred on a gradient that depended on the distance of the skin from the base of the flap. Microvascular flow grew to higher than preoperative levels from 14 to 21 days and then gradually returned to baseline during the fourth week. The opening of collateral vessels appears to allow this sequential recovery of blood flow. However, there appears to be a limit on how fast these collaterals can open.15
This time-dependent opening of collateral vessels may be partially explained by arteriovenous (AV) shunts. These shunts control blood supply to the capillary network that supplies the flap. There are pre-AV shunt sphincters under the control of the sympathetic nervous system. When the flap is incised and undermined, local sympathetic nerve fibers are disrupted and release catecholamines. As a result, there is local vasoconstriction for up to 48 hours, by which time the nerve’s supply of norepinephrine is exhausted.26,30 Once sympathetic tone is relaxed, the blood flow to the capillary collaterals is increased to help supply the flap with nutrients.14 However, this effective sympathectomy cannot fully explain why random flaps have a graded flow recovery. Upon incision, the entire flap should have equal catecholamine release and subsequent equal flow recovery.31 Other humoral factors such as prostaglandin release may come into play.32
Local tissue conditions resulting from surgical trauma also decrease flap perfusion and subsequent survival. Following any local injury, the inflammatory cascade releases the powerful vasoconstrictor thromboxane A2.33,34 In addition, free radicals are released, causing direct injury to the flap.35,36 Finally, the edema inevitably associated with surgical trauma causes further capillary vessel resistance by increasing manual compression on the skin’s smaller caliber perfusion sources.37 All of these negative factors decrease perfusion to the flap.
Conversely, the flap may also benefit from surgical trauma. Relative local hypoxemia and increased levels of metabolic by-products induce opening of pre-capillary sphincters, thereby promoting increased local blood flow.26,30,38 Moreover, adhesion molecules, such as E-selectin, are activated following exposure to released coagulation cascade molecules such as endotoxin, interleukin-1, and tumor necrosis factor alpha.39 These adhesion molecules recruit molecules including neutrophils to the flap to clear debris and anabolic waste products. Finally, ischemic tissue attracts endothelial progenitor cells, which allow for the ingrowth of new vascular channels to supply the flap.43 Flap survival is dependent on the balance of all these factors ultimately influencing pedicle blood flow.
The nascent flap not only receives nutrients from the pedicle, but it also gains nutrition from the base of the primary defect through angiogenesis, revascularization, and neovascularization.40–43 Within the first two days of flap placement, a fibrin layer develops below the flap and provides a suitable environment for angiogenesis.35 Endothelial cells and macrophages release angiogenic cell factors important in neovascularization, the local growth of new blood vessels into the surgically manipulated skin.43–46 Neovascularization is seen as early as 3 days in the rat model47 and at 4 days in rabbit48 and pig49 models. In staged, pedicled flaps in humans, revascularization adequate for division of the flap pedicle has been demonstrated by the seventh postoperative day.49–51 This new vascular growth works in conjunction with the pre-existing collateral vessels to nourish the flap.
Tissue edema, wound closure tension, and infection also negatively affect flap blood supply and survival. Although none of these factors can solely lead to necrosis of a well-vascularized flap, each can contribute to further ischemia in a marginally perfused flap. Postoperative tissue edema places external force on small capillaries,37 resulting in increased capillary resistance. Thus, there must be greater perfusion pressure at the pedicle to counter this resistance and ensure flap tip survival. Recent studies in the rat model revealed that significant postoperative edema will not solely cause flap necrosis.52 However, it can be an additive factor along with high wound closure tensions and/or infection.
Closing wounds under large amounts of tension can place undue vascular stress on the wound edges and tip of the flap. It is typically recommended that one undermine 2 to 4 cm,53 or 50 to 100% of the defect width,108 beyond the wound edge to decrease wound tension. Undermining beyond this distance may be detrimental since there may be unnecessary vascular compromise, more bleeding, and greater dead space, all of which can lead to surgical complications. High closure tension leads to dehiscence and wound edge necrosis, but it does not usually lead to entire flap necrosis.54–56
Wound infection can cause partial or complete flap necrosis. With local infection, there is release of toxic free radicals and greater tissue edema.57 Infection can also lead to vessel thrombosis.58 In addition to local tissue destruction and vascular compromise, collagen production and deposition are hindered.59 Therefore, flap adhesion to the wound bed and overall tensile strength are affected.
FLAP BIOMECHANICS
In addition to understanding the skin’s vascular supply, the surgeon must also appreciate the unique biomechanical properties of skin if flap surgery is to be successful. All materials have characteristic biomechanical properties: stress, strain, creep, and stress relaxation. In regard to skin, stress is the force applied per cross-sectional area, and strain is the change in length divided by the original length of the given tissue to which a given force is applied. The stress–strain relationship of skin shows that skin, unlike some other materials, is not truly elastic (Figure 2.5). As a small amount of stress (or tension) is placed on the skin, there is a corresponding change in the skin’s length (strain). At a certain point on the stress–strain curve (zone III in Figure 2.5), even a large amount of applied force will not result in further incremental skin stretch.60 This nonelastic property of skin is mainly due to its structural constituents—collagen and elastin. In relaxed skin, collagen is randomly oriented, and elastin is loosely wrapped around and attached to multiple points on the collagen bundles.61–65 When a small amount of force is initially applied to skin, the elastin network is first deformed66,67 and the skin is easily lengthened (zone I in Figure 2.5). With sun exposure and intrinsic aging, there is a progressive decrease in the functional elastic fiber network.67 As a result, the stress–strain curve is shifted to the right so that when applied to aged or sun-damaged skin, less applied force results in greater lengthening. As continued force is applied, the collagen fibers begin to reorient parallel to the direction of the force (rapid transition of the curve, zone II in Figure 2.5).65 At the point where the elastin and collagen fibers are maximally stretched, even a large amount of force will only minimally stretch the skin (zone III in Figure 2.5). Thus, the skin is not truly elastic at all levels of applied tension.
Creep refers to the increase in strain seen when skin is under constant stress. When the skin is held at sufficient tension, elastic fibers will fragment and collagen fibers will align parallel to the applied force. As a result of this reorganization of the elastic and collagen fiber networks, interstitial fluid will be displaced and can be seen with the naked eye.68 Creep typically begins to occur within several minutes of the constant force application.
While skin demonstrates elastic properties with low loads, skin exhibits “stress relaxation” and creep when larger forces are applied for longer periods of time. Stress relaxation occurs when skin is held under constant tension. In this case the amount of force (stress) required to maintain this tension decreases with time. The skin’s relaxation under stress is closely related to its ability to increase in length when placed under a constant stress (the creep phenomenon).65
When skin is held at a constant tension for several days, stress relaxation occurs. There is a decrease in stress due to an increase in skin cellularity and the permanent stretching of skin components.65 In clinical practice, serial excision and tissue expansion utilize the principles of creep and stress relaxation. When a large lesion is partially excised and reconstructed under tension, the skin relaxes and lengthens, allowing further staged excisions.
FLAPS DEFINED BY MOVEMENT
In addition to classifying flaps by their vascularity, flaps are often also categorized by their primary movement directions. The three basic flap movements are advancement, rotation, and transposition. With advancement flaps, the major motion of the flap is largely along a single linear direction towards the primary defect (Figure 2.6). Rotation flaps are those flaps that are rotated in an arc along a pivot point to fill the adjacent primary defect without crossing any intervening skin (Figure 2.7). A transposition flap is incised and lifted over intact skin and then placed into the wound (Figure 2.8). A distinct type of transposition flap is called the interpolation flap. An interpolation flap is a flap, potentially with a predictable, axial vascular supply, that involves two steps or stages. The first stage of the flap is transposing the tissue into the wound with the pedicle overlying the intervening skin. The second stage is separating the flap from its origin and therefore dividing its pedicle.
In addition to classifying flaps by their unidirectional motion, some authors, noting the inherent limitations of such schemata, categorize flaps as sliding or lifting flaps.69,70 Sliding flaps include advancement and rotation flaps, where tissue is pushed into the primary defect. A lifting flap is the typical transposition flap, in which tissue must be lifted over normal skin to fill the wound. These more general classifications recognize that very few flaps move tissue along a single, predictable path. For example, most rotation flaps also incorporate a significant degree of tissue advancement.
When discussing the motion of these flaps, the surgeon must understand the tension vector created by the flap’s closure. This vector depends on the primary and secondary motion of the flap. The primary motion of the flap is the tension placed on the flap tissue as it is moved to fill the wound (primary defect) (Figure 2.9). The secondary motion, created by the movement of the flap into the defect, is the force placed on the tissue surrounding the primary defect (Figure 2.9). The combined force created by the primary and secondary motions causes a new tension vector that must be anticipated by the surgeon when deciding which flap can best be utilized for repair. This final tension vector varies, depending on the flap chosen, and may be appreciated when placing the key stitch. The key stitch is the stitch placed to initially move the flap and accurately place it into the primary defect.71 If not properly planned, the flap’s final tension vector may cause undesirable pulling on free margins such as the eyelid, alar rim, and mouth.
Advancement Flap
The advancement flap has long been used in facial reconstruction. Its first description has been attributed to Celsus of ancient Rome, who used the flap for the reconstruction of the cheek and ears.72,73 In the 1800s, French surgeons advocated the use of “lambeau par glissement” (sliding flaps) for facial reconstruction.74 In 1885, Burow modified and further described the advantages of this flap in reconstruction.75
The unilateral, bilateral, and V to Y advancement flaps are the most commonly used advancement flaps in dermatologic surgery. The primary and secondary motions, and thus the resulting tension vectors of these flaps, are usually in a simple linear line along the direction of the flap movement75 (Figure 2.6). The key stitch typically brings the leading edge of the flap to the opposite wound edge to close the primary defect. As the tissue is advanced and sutured into place, a standing cone (or cones) develop(s). These standing cone deformities, or dog ears, are caused by the pouching of excess, compressed skin near the flap’s base when tissue is advanced under tension.76 These tissue protrusions can be anticipated and a triangle of skin, called a Burow’s triangle,77 can be removed from anywhere along the skin surrounding the flap (Figure 2.6). The standing cone may also be corrected either by extending the incision line to remove the excess tissue or using an M-plasty78,79 (Figure 2.10a). By truncating one end of the closure, the M-plasty flap has the advantage of providing a shorter final wound length. When using bilateral advancement flaps to correct a circular defect, the repair may be described as an A-to-T (T-plasty) or an O-to-H flap (H-plasty) (Figure 2.10b). These unilateral and bilateral advancement flaps are most commonly used in repairs on the forehead, scalp, and eyelids.
The V-to-Y advancement flap, or island pedicle flap,80 utilizes a subcutaneous pedicle that perfuses a triangular flap that advances along a single direction (Figure 2.10c). All borders of the flap are incised to the subcutaneous fat, and the resulting island of tissue, now liberated from lateral dermal attachments, is advanced to fill the defect. The pedicle is underneath rather than at the edge of the flap and may include muscle.81–83 Various modifications84,85 such as parallel release incisions of the pedicle base allow significant advancement of the flap with predictable flap survival.86 Because the flap’s muscular-containing, thick pedicle ensures liberal blood flow, this flap is the most likely of all flaps to survive in patients with tobacco use which can result in decreased perfusion. The flap can be used anywhere on the face, but the authors find this flap to be particularly useful for repairs of the upper cutaneous lip, the alar–cheek sulcus, and the lateral eyebrow.
Rotation Flaps
In 1842, Pancoast described using rotation flaps to close facial wounds.87 Like most random pattern flaps, rotation flaps recruit tissue adjacent to the primary defect. An arc or semicircle flap is incised and pivoted into the wound (Figure 2.11). Thus, the primary motion is rotation. Similar to advancement flaps, the secondary motion of the flap causes a standing cone deformity at the base of the flap. This Burow’s triangle may be excised from anywhere along the arc of the flap (Figure 2.11a). Another similarity between rotation and advancement flaps is the placement of the key stitch. This first stitch closes the primary defect.
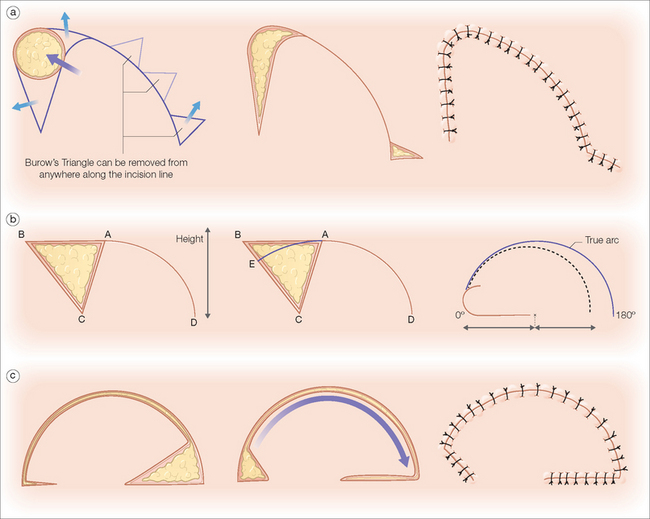
Figure 2.11 Rotation flaps. (a) Rotation flaps utilize tissue adjacent to the primary defect. The semicircular flap is incised and pivoted along one axis into the wound. The Burow’s triangle may be excised from anywhere along the tissue arc of the flap to prevent a standing cone deformity and allow easier flap movement into the defect. (b) When the rotation flap AD is rotated along its pivot point C, it will only reach point E and fill the triangle ACE without tension. The effective length of any flap is shortened when rotating on a fixed point (seen on right). To compensate for this functional length loss, Dzubow recommends that the planned height actually be greater than the defect height.89 (c) To increase the forward rotation of the flap, a back-cut may be employed. Although there is improved movement, there is increased risk of distal flap ischemia since the back-cut decreases the size of the vascular pedicle.
The surgeon must pay particular attention to the size of the designed rotation flap. Because it is rotated along an arc, the functional flap length is shorter than the actual length of the flap’s incision (Figure 2.11b). Hence, the planned length must be longer than the diameter of the wound.88 Dzubow recommends that the planned height of the flap be greater than the height of the defect to account for this functional loss of length.89 One advantage of the rotation flap is that its base is typically broad and, therefore, the flap’s length-to-width ratio may be as long as 6:1.90 This makes the flap particularly useful when closing inelastic areas such as the scalp.
To increase the movement of the rotation flap, a back-cut may be employed. A back-cut is when an incision is placed into the pedicle of the flap to allow greater forward movement (Figure 2.11c). Although the back-cut can increase flap mobility, this maneuver cuts into the flap’s base, thereby increasing the risk of tip ischemia. In areas with excellent vascularity such as the gabella, the back-cut is often incorporated into the rotation flap’s design.
Rotation flaps are most often utilized for repairs of the scalp, forehead, cheek, and nose. When two rotation flaps are designed to repair the defect, the closure is called an O-to-Z repair. The Rieger flap,91 or dorsal nasal rotation flap, uses a back-cut in its design to allow closure of small- to medium-sized defects of the distal third of the nose. In 1985, Marchac and Toth modified the flap design by defining an axial pedicle for the flap based on the angular artery.92 This modification allows a greater flap length-to-pedicle base ratio because of a reliable blood supply.
Transposition Flaps
Transposition flaps were documented in the pre-Common Era. In about 2000 bc, Indians committing adultery were punished by nasal tip amputation. Although reconstructive procedures may have occurred even at that time, the Indian surgeon Sushrata Samita of the eighth century bc is credited with first describing nasal reconstruction using a cheek transposition flap.1 By approximately 1000ad Samita’s heirs introduced the “Indian flap,” a forehead flap used to cover nasal defects. By the fifteenth century, this surgical technique found its way to Europe, and the flap’s use continues today.93
Dermatologic surgeons most commonly utilize the rhombic, bilobed, and melolabial transposition flaps. The rhomboid, or Limberg,94 flap utilizes the geometry of two equilateral triangles placed base-to-base to form the shape of an equilateral parallelogram (rhombus) (Figure 2.12a). The typically circular primary defect can be modified to resemble a rhombus where all sides are equal in length, two interior angles are 60°, and two interior angles are 120°. The first flap incision is designed along the short diagonal and will equal the length of each side of the rhombus. As Figure 2.12a depicts, the angle of the flap incision will equal 60°, and four different rhombic flaps can be potentially created. A variation of the traditional Limberg flap is the Dufourmentel95 rhomboid flap, which utilizes a 30° interior flap angle (Figure 2.12b). This smaller angle at the flap’s apex can minimize the possibility of a distracting tissue bulge near the peak of the flap’s donor site. The rhombic flap is extremely versatile and can be used anywhere on the face, provided that the secondary motion at the flap’s donor site is carefully considered.
Another variant of the transposition flap is the bilobed flap, a useful flap that allows tissue mobilization in areas where closure of the secondary defect is difficult. Esser first described this flap in 1918 for nasal tip reconstruction in three case reports96 (Figure 2.13a). In the bilobed design, there are actually two flaps that share a common pedicle. The primary flap is designed to close the primary defect, and the second flap fills the secondary defect (Figure 2.13b). Because the secondary lobe’s donor site is typically located in an area of relative skin excess, this tertiary defect can be closed in a simple side-to-side manner. Although the design nuances of the bilobed flap can be initially confusing, the movement of the flap can be accurately predicted if the basic principles of transposition flaps are well understood.
A third commonly used transposition flap in dermatologic surgery is the melolabial, or nasolabial banner, flap. With this type of flap, the base may be located on the inferior or superior97 cheek, depending on the coverage needed (Figure 2.14). The base contains a highly vascular array of perforating vessels derived from the angular artery. As a result of this excellent perfusion, the melolabial flap may be designed significantly longer than the typically acceptable 3:1 length-to-base ratio and still survive. The secondary defect at the flap’s donor site is easily closed due to the laxity of medial cheek tissue.
PRACTICAL POINTS
The most suitable color and texture match in facial reconstruction is obtained when a flap is taken from the same facial aesthetic unit as the adjacent defect. Gonzalez-Ulloa first described the aesthetic facial units98 (Figure 2.15). Skin varies in color, texture, and appendageal characteristics depending on the location on the face. Areas of the face that share similar skin characteristics and that are commonly bordered by visually prominent, naturally occurring anatomic landmarks are considered to be within a single aesthetic unit.99–101 The two most complicated areas for reconstruction on the face are the nose and the lip. On the nose, there are many different small subunits divided by the skin’s color, texture, and mobility.102,103 Robinson recently published a 10-year prospective study confirming improved cosmetic results when wounds were reconstructed within aesthetic units.104 She emphasized that when a primary defect involves multiple aesthetic units, each unit should be reconstructed individually with sutures placed in the borders between units. If a wound involves more than 50% of the subunit, the authors often remove the unit’s remaining tissue and reconstruct the unit as a whole. Although some normal tissue is sacrificed, the final cosmetic result is superior since scars are least conspicuous when they are properly placed at the junction of aesthetic units.
When designing any closure, the surgeon must identify tissue reservoirs using the “smart finger.” Tissue surrounding the wound should be palpated, pushed, and pinched to evaluate tissue laxity and favorable relaxed skin tension lines (RSTLs),105,106 which are those curvilinear lines seen at rest in elderly patients. In young patients, pinching the skin will identify these furrows. The lateral and inferior face usually has greater tissue reservoirs than the central zones of the face.107 Tissue may be borrowed from these areas, but incisions should be planned to be parallel to the RSTLs in order to minimize scar visibility (Figure 2.16). When an incision is placed within an RSTL, the wound’s tension vectors parallel the lines of maximal extensibility, and there is less tension on the wound edge. Probing fingers will locate the best reservoir to allow wound closure with minimal tension and to produce the best functional and cosmetic results.
FINAL THOUGHTS
Surgical technique and knowledge of anatomy must be refined. There is no substitution for understanding the anatomy of any area to be reconstructed. This familiarity will help one avoid transecting major cutaneous arteries and important motor nerves. Skin hooks and fine-tooth forceps should be utilized to avoid blunt trauma to tissues. Wide undermining should be utilized to prevent flap elevation (pin-cushioning) during healing.108 Meticulous hemostasis must be obtained prior to final suturing. Moreover, any dead space beneath the flap should be eliminated with the conservative use of tacking sutures.
1 Wallace AF. History of plastic surgery. J Royal Soc Med. 1978;71:834-838.
2 Cutting C, Ballantyne D, Shaw W, Converse JM. Critical closing pressure, local perfusion pressure, and the failing skin flap. Ann Plast Surg. 1982;8:504-509.
3 Milton SH. Pedicled skin flaps: the fallacy of the length:width ratio. Brit J Surg. 1970;57:502-508.
4 Daniel RK, Williams HB. The free transfer of skin flaps by microvascular anastomoses. An experimental study and a reappraisal. Plast Reconstr Surg. 1973;52:16-31.
5 Stell PM. The pig as an experimental model for skin flap behaviour: a reappraisal of previous studies. Brit J Plast Surg. 1977;30:1-8.
6 Patterson TJ. The survival of skin flaps in the pig. Br J Plast Surg. 1968;21:113-117.
7 Patterson TJ. Study of the blood-supply of skin-flaps by close-up thermography. Br J Surg. 1969;56:381.
8 Dzubow LM. Tissue movement—a microbiomechanical approach. J Dermatol Surg Oncol. 1989;15:389-399.
9 Baran NK, Horton CE. Growth of skin grafts, flaps, and scars in young minipigs. Plast Reconstr Surg. 1972;50:487-496.
10 Kernahan DA, Zingg W, Kay CW. The effect of hyperbaric oxygen on the survival of experimental skin flaps. Plast Reconstr Surg. 1965;36:19-25.
11 Donovan WE. Experimental models in skin flap research. In: Grabb WC, Myers MB, editors. Skin flaps. Boston: Little, Brown; 1975:11-20.
12 Daniel RK. The anatomy and hemodynamics of the cutaneous circulation and their influence on skin flap design. In: Grabb WC, Myers MB, editors. Skin flaps. Boston: Little, Brown; 1975:111-131.
13 Neligan P, Pang CY, Nakatsuka T, Lindsay WK, Thomson HG. Pharmacologic action of isoxsuprine in cutaneous and myocutaneous flaps. Plast Reconstr Surg. 1985;75:363-374.
14 Pearl RM. A unifying theory of the delay phenomenon—recovery from the hyperadrenergic state. Ann Plast Surg. 1981;7:102-112.
15 Marks NJ. Quantitative analysis of skin flap blood flow in the rat using laser Doppler velocimetry. J R Soc Med. 1985;78:308-314.
16 Guyton AC. Textbook of medical physiology, 6th edn., Philadelphia: WB Saunders; 1981:344-356.
17 Pearl RM, Johnson D. The vascular supply to the skin: an anatomical and physiological reappraisal-Part I. Ann Plast Surg. 1983;11:99-105.
18 Pearl RM, Johnson D. The vascular supply to the skin: an anatomical and physiological reappraisal-Part II. Ann Plast Surg. 1983;11:196-205.
19 Midy D, Mauruc B, Vergnes P, Caliot P. A contribution to the study of the facial artery, its branches and anastomoses: application to the anatomic vascular basis of facial flaps. Surg Radiol Anat. 1986;8:99-107.
20 Cutting C. Critical closing and perfusion pressures in flap survival. Ann Plast Surg. 1982;9:524.
21 Mathes SJ, Naha F. The reconstructive triangle: a paradigm for surgical decision making. In: Reconstructive surgery: principles, anatomy, and technique. New York: Churchill Livingston; 1997:9-36. 37–160.
22 Fazio MJ, Zitelli JA. Flaps. In: Ratz JL, Geronemus RC, Goldman MP, et al, editors. Textbook of dermatologic surgery. Philadelphia: Lippincott-Raven; 1998:225-227.
23 Heniford BW, Bailin PL, Marsico RE. Field guide to local flaps. Dermatol Clin. 1998:65-74.
24 Stranc MF, Sanders R. Abdominal wall skin flaps. In: Grabb WC, Myers MB, editors. Skin flaps. Boston: Little, Brown; 1975:419-426.
25 Hartwell SWJr. Local flaps of the leg and foot. In: Grabb WC, Myers MB, editors. Skin flaps. Boston: Little, Brown; 1975:497-506.
26 Meyers B. Understanding flap necrosis. Plast Reconstr Surg. 1986;78:813-814.
27 Kerrigan C, Daniel R. Critical ischemia time and the failing skin flap. Plast Reconstr Surg. 1982;69:986-989.
28 Zelt RG, Olding M, Kerrigan CL, Daniel RK. Primary and secondary critical ischemia times of myocutaneous flaps. Plast Reconstr Surg. 1986;78:498-503.
29 Guba AM, Zinner MJ, Hobson RW. Regional hemodynamics of a pedicle flap: evaluation by distribution of radioactive microspheres. J Surg Res. 1978;25:274-279.
30 Kerrigan CL, Zelt RG, Daniel RK. Secondary critical ischemia time of experimental skin flaps. Plast Reconstr Surg. 1984;74:522-526.
31 Gottrup F, Oredsson S, Price DC, Mathes SJ, Hohn DC. A comparative study of skin blood flow in musculocutaneous and random pattern flaps. J Surg Res. 1984;37:443-447.
32 Sasaki GH, Pang CY. Experimental evidence for involvement of prostaglandins in viability and acute skin flaps: effects on viability and mode of action. Plast Reconstr Surg. 1981;67:335-340.
33 Kerrigan CL, Stotland MA. Ischemia reperfusion injury: a review. Microsurgery. 1993;14:165-175.
34 Kay S, Green C. The effect of a novel thromboxane synthetase inhibitor dazmegrel (UK38485) on random pattern skin flaps in the rat. Br J Plast Surg. 1986;39:361-363.
35 Goding GSJr, Horn DB. Skin flap physiology. In: Baker SR, Swanson NA, editors. Local flaps in facial reconstruction. St Louis, MO: Mosby; 1995:15-30.
36 Angel MF, Narayanan K, Swartz WM, et al. The etiologic role of free radicals in hematoma-induced flap necrosis. Plast Reconstr Surg. 1986;77:795-803.
37 Mellow CG, Knight KR, Angel MF, Coe SA, O’Brien BM. The biochemical basis of secondary ischemia. J Surg Res. 1992;52:226-232.
38 Kerrigan CL, Daniel RK. Skin flap research: a candid view. Ann Plast Surg. 1984;13:383-387.
39 Wang C, Kerrigan CL, Stotland MA. Kinetics of E-selectin expression in surgical flaps. Plast Reconstr Surg. 1997;100:1482-1488.
40 Tsur H, Daniller A, Strauch B. Neovascularization of skin flaps: route and timing. Plast Reconstr Surg. 1980;66:85-93.
41 Pickett BP, Burgess LP, Livermore GH, Tzikas TL, Vossoughi J. Wound healing. Arch Otolaryngol Head Neck Surg. 1996;122:565-568.
42 Jonsson K, Hunt TK, Brennan SS, Mathes SJ. Tissue oxygen measurements in delayed skin flaps: a reconsideration of the mechanisms of the delay phenomenon. Plast Reconstr Surg. 1988;82:328-336.
43 Park S, Tepper OM, Galiano RD, et al. Selective recruitment of endothelial progenitor cells to ischemic tissues with increased neovascularization. Plast Reconstr Surg. 2004;113:284-293.
44 Jonasson K, Hunt TK, Brennan S, Mathes SJ. Tissue oxygen measurements in delayed skin flaps: a reconsideration of the mechanisms of the delay phenomenon. Plast Reconstr Surg. 1988;82:328-336.
45 Liu PY, Wang XT, Badiavas E, Rieger-Christ K, Tang JB, Summerhayes I. Enhancement of ischemic flap survival by prefabrication with transfer of exogenous PDGF gene. J Reconstr Microsurg. 2005;21:273-279.
46 Simman R, Craft C, McKinney B. Improved survival of ischemic random skin flaps through the use of bone marrow nonhematopoietic stem cells and angiogenic growth factors. Ann Plast Surg. 2005;54:546-552.
47 Gatti JE, LaRossa D, Brousseau DA, Silverman DG. Assessment of neovascularization and timing of flap division. Plast Reconstr Surg. 1984;73:396-402.
48 Serafin D, Shearin C, Georgiade NG. The vascularization of free flaps: a clinical and experimental correlation. Plast Reconstr Surg. 1977;60:233-241.
49 Tsur H, Daniller A, Strauch B. Neovascularization of skin flaps: route and timing. Plast Reconst Surg. 1980;66:85-90.
50 Klingenstrom P, Nylen B. Timing of transfer of tubed pedicles and cross flaps. Plast Reconstr Surg. 1966;37:1-12.
51 Cummings C, Trachy R. Measurement of alternative blood flow in the porcine panniculus carnosus myocutaneous flap. Arch Otolaryngol. 1985;111:598-600.
52 Demirseren ME, Yenidunya MO, Yenidunya S. Island rat groin flaps with twisted pedicles. Plast Reconstr Surg. 2004;114:1190-1194.
53 Leach J. Proper handling of soft tissue in the acute phase. Facial Plast Surg. 2001;17:227-238.
54 Myers MB. Wound tension and vascularity in the etiology and prevention of skin sloughs. Surgery. 1964;56:945-949.
55 Myers MB, Combs B, Cohen G. Wound tension and wound sloughs–a negative correlation. Am J Surg. 1965;109:711-714.
56 Larrabee WF. Design of local skin flaps. Otolaryngol Clin North Am. 1990;23:899-923.
57 Salasche SJ. Acute surgical complications: cause, prevention, and treatment. J Am Acad Dermatol. 1986;15:1163-1185.
58 Myers MB. Investigation of skin flap necrosis. In: Grabb WC, Myers MB, editors. Skin flaps. Boston: Little, Brown; 1975:3-10.
59 Salasche SJ, Grabski WJ. Complications of flaps. J Dermatol Surg Oncol. 1991;17:132-140.
60 Larrabee WFJr. Immediate repair of facial defects. Dermatol Clinic. 1989;7:661-676.
61 Lanir Y. A structural theory for the homogeneous biaxial stress-strain relationships in flat collagenous tissues. J Biomech. 1979;12:423-436.
62 Daly CH, Odland GF. Age-related changes in the mechanical properties of human skin. J Invest Dermatol. 1979;73:84-87.
63 Larrabee WFJr, Galt JA. A finite element model for the design of local skin flaps. Otolaryngol Clin North Am. 1986;19:807-824.
64 Lanir Y, Walsh J, Soutas-Little RW. Histological staining as a measure of stress in collagen fibers. J Biomech Eng. 1984;106:174-176.
65 Ridenour BD, Larrabee WF. Biomechanics of skin flaps. In: Baker SR, Swanson NA, editors. Local flaps in facial reconstruction. St. Louis, MO: Mosby; 1995:31-38.
66 Daly CH, Odland GF. Age-related changes in the mechanical properties of human skin. J Invest Dermatol. 1979;73:84-87.
67 Larrabee WF, Sutton D. The biomechanics of advancement and rotation flaps. Laryngoscope. 1981;91:726-734.
68 Gibson T, Kenedi RM. Biomechanical properties of skin. Surg Clin North Am. 1967;47:279-294.
69 Swanson NA. Classifications, definitions, and concepts in flap surgery. In: Baker SR, Swanson NA, editors. Local flaps in facial reconstruction. St. Louis, MO: Mosby; 1995:63-74.
70 Johnson TM, Swanson N, Baker SR. Concepts of sliding and lifting tissue movement in flap reconstruction. Dermatol Surg. 2000;26:274-278.
71 Robinson JK. Placement of the tension-bearing suture in repairing the alar facial junction. J Am Acad Dermatol. 1997;36:440-443.
72 Marmelzat WL. Medicine in history—Celsus (25ad), plastic surgeon: on the repair of defects of the ears, lips, and nose. J Dermatol Surg Oncol. 1982;8:1012-1014.
73 Zelac NE, Swanson N, Simpson M, Greenway HT. The history of dermatologic surgical reconstruction. Dermatol Surg. 2000;26:983-990.
74 Hale EK. The history of flaps. J Drugs Dermatol. 2002;1:293-296.
75 Burow A. Beschreibung einer neuen Transplantations-methode (Methode der seitlichen Dreiecke) zum Wiederersatz verlorengegangener Teile des Gesichts J. Berlin: Nauck, 1855.
76 Gormley DE. The dog-ear: causes, prevention, and correction. J Dermatol Surg Oncol. 1977;3:194-198.
77 Gormley DE. A brief analysis of the Burow’s wedge/triangle principle. J Dermatol Surg Oncol. 1985;11:121-123.
78 Webster RC, Davidson TM, Smith RC, et al. M-plasty techniques. J Dermatol Surg. 1976;2:393-396.
79 Salasche SJ, Roberts LC. Dog-ear correction by M-plasty. J Dermatol Surg Oncol. 1984;10:478-482.
80 Barron JN, Emmett AJ. Subcutaneous pedicled flaps. Br J Plast Surg. 1965;18:51-78.
81 Tomich JM, Wentzell JM, Grande DJ. Subcutaneous island pedicle flaps. Arch Dermatol. 1987;123:514-518.
82 Papadopoulous DJ, Trinei FA. Superiorly based nasalis myocutaneous island pedicle flap with bilevel undermining for nasal tip and supratip reconstruction. Dermatol Surg. 1999;25:530-536.
83 Krishnan R, Garman M, Nunez-Gussman J, Orengo I. Advancement flaps: a basic theme with many variations. Dermatol Surg. 2005;31:986-994.
84 Hairston BR, Nguyen H. Innovations in the island pedicle flap for cutaneous facial reconstruction. Dermatol Surg. 2003;29:378-385.
85 Vandeput J. The new bilaterally pedicled V-Y advancement flap for face reconstruction. Plast Reconstr Surg. 2003;111:1363-1364.
86 Skaria AM. Refinement of the island pedicle flap: Parallel placed release incisions to increase translation movement. Dermatol Surg. 2004;30:1595-1598.
87 Pancoast J. New operation for the relief of persistent facial neuralgia. Medical Times of Philadelphia. 1872;2:85-87.
88 Konz B. Use of skin flaps in dermatologic surgery of the face. J Dermatol Surg. 1975;1:25-30.
89 Dzubow LM. The dynamics of flap movement: effect of pivotal restraint on flap rotation and transposition. J Dermatol Surg Oncol. 1987;13:1348-1353.
90 Crow ML, Crow FJ. Resurfacing large cheek defects with rotation flaps from the neck. Plast Reconstr Surg. 1976;58:196-200.
91 Rieger RA. A local flap for repair of the nasal tip. Plast Reconstr Surg. 1967;40:147-149.
92 Marchac D, Toth B. The axial frontonasal flap revisited. Plast Reconstr Surg. 1985;76:686-694.
93 Chase RA. Introduction: The history of vascularized composite-tissue transfers. In Strauch B, Vasconez LO, Hall-Findlay E, editors: Grabb’s encyclopedia of flaps, 2nd edn., New York: Lippincott-Raven, 1988.
94 Limberg AA. Modern trends in plastic surgery. Design of local flaps. Mod Trends Plast Surg. 1966;2:38-61.
95 Dufourmental C. Closure of limited loss of cutaneous substance. So-called “LLL” diamond-shaped L rotation-flap. Ann Chir Plast. 1962;7:60-66.
96 Gestielite lokale Nasemplastik mit zweizipfligem Lappen, Deckung des sekundaren Defektes vom ersten Zipfel durch den zweiten. Dtsch Z Chir. 1918;143:385-390.
97 Pharis DB, Papadopoulos DJ. Superiorly based nasolabial interpolation flap for repair of complex nasal tip defects. Dermatol Surg. 2000;26:19-24.
98 Gonzalez-Ulloa M. Restoration of the face covering by means of selected skin in regional aesthetic units. Br J Plast Surg. 1956;9:212-221.
99 Webster RC, Smith RC. Cosmetic priniciples in surgery on the face. J Dermatol Surg Oncol. 1978;4:397-402.
100 Dzubow LM, Zack L. The principle of cosmetic junctions as applied to reconstruction of defects following Mohs surgery. J Dermatol Surg Oncol. 1990;16:353-355.
101 Burget GC. Modification of the subunit principle. Arch Facial Plast Surg. 1999;1:16-18.
102 Burget GC, Menick FJ. The subunit principle in nasal reconstruction. Plast Reconstr Surg. 1985;76:239-247.
103 Burget GC. Aesthetic reconstruction of the tip of the nose. Dermatol Surg. 1995;21:419-429.
104 Robinson JK. Segmental reconstruction of the face. Dermatol Surg. 2004;30:67-74.
105 Stegman SJ. Guidelines for placement of elective incisions. Cutis. 1976;18:723-726.
106 Summers BK, Siegle RJ. Facial cutaneous reconstructive surgery: general aesthetic principles. J Am Acad Dermatol. 1993;29:669-681.
107 Summers BK, Siegle RJ. Facial cutaneous reconstructive surgery: facial flaps. J Am Acad Dermatol. 1993;29:917-941.
108 Boyer JD, Zitelli JA, Brodland DG. Undermining in cutaneous surgery. Dermatol Surg. 2001;27:75-78.