Chapter 32 Applied Anatomy of the Thoracic and Lumbar Spine
Spine Alignment
The acquisition of upright posture and bipedal locomotion during human evolution imposed specific demands on the thoracic and lumbar spine. These demands are reflected in its overall structure (Fig. 32-1). Upright posture requires added load-bearing capacity; along the rostral-caudal axis, there is a substantial increase in the robustness not only of the vertebral bodies, but also of other stabilizing structures, such as the articular processes and spinous processes. Bipedalism requires spinal balance; this is reflected in normal spinal curvature. Unlike our quadruped ancestors, who possessed a single, broad spinal curvature, humans exhibit a combination of thoracic kyphosis and lumbar lordosis. These curves are formed by relatively small variations in intervertebral disc and vertebral body morphology. In the absence of spine deformity, the thoracic and lumbar curvatures are balanced, and the midportion of the C7 vertebral body sits atop the L5-S1 pivot point, located at the dorsal aspect of the L5-S1 intervertebral disc. With normal spinal balance, the degree of thoracic and lumbar curvature may vary considerably without adverse effect. However, deviations from normal sagittal alignment are mechanically unfavorable, imposing stress on axial musculature and resulting in pain and acceleration of the degenerative process.
Vertebrae and Ligaments
Vertebral Body
Sagittal vertebral morphology also changes along the rostral-caudal axis (Fig. 32-2). Thoracic vertebral bodies are wedge shaped, ventral height being shorter than dorsal height. This results in the normal thoracic kyphosis. In the lumbar spine, ventral and dorsal heights are generally comparable, and it is the disc shape, rather than vertebral body shape, that is the principal contributor to lumbar lordosis. At L4 and L5, some reverse wedging of the vertebral body may occur, with increased ventral height further contributing to the lower lumbar lordosis. The vertebral body gradually increases in cross-sectional area and height from the thoracic spine to the midlumbar spine (Figs. 32-3 and 32-4). From L2 to L5, vertebral height is usually stable and may decrease slightly. Changes in cross-sectional area are reflected in compression strength (Fig. 32-5).
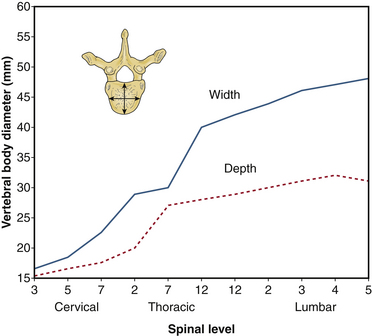
FIGURE 32-3 Vertebral body diameter by level.
(Benzel EC: Biomechanics of spine stabilization, New York, 2001, Thieme, p 1, reprinted with permission.)
Intervertebral Disc and Vertebral End Plate
The general function of the intervertebral disc is twofold: (1) It deforms to accommodate compressive loads, a role assumed by the nucleus pulposus, and (2) it resists tensile and torsional stresses, a role assumed by the anulus fibrosus (Fig. 32-6). At a microscopic level, the nucleus pulposus consists of a semifluid, gel-like substance embedded in a fine meshwork of fibrous strands. This structure results in a viscoelastic property that allows the disc to withstand and absorb axial stress. The anulus fibrosus has a lamellated boundary of intersecting fibrous strands. Anular fibers known as Sharpey fibers penetrate the dense cortical bone that makes up the outer ring of the vertebral end plate. The outermost fibers blend with overlying periosteum and longitudinal ligaments. Viewed in histologic cross section, the transition from nucleus pulposus to anulus fibrosus is a gradual one.
The bony vertebral end plate is a concave depression. At its central portion, the cancellous bone of the vertebral body is directly apposed to a cartilaginous plate, which fills the depression up to the level of the apophyseal ring, or marginal ring. The apophyseal ring is composed of cortical bone and is more resistant to compression failure than is the central end plate. Biomechanical studies have shown the strongest region of the lumbar end plate to be the dorsal, lateral aspect of the marginal ring, adjacent to the pedicle.1
Posterior Longitudinal Ligament
The posterior longitudinal ligament (PLL) also spans the full rostral-caudal axis of the spine but is less substantial than the ALL. It is located along the dorsal surface of the vertebral bodies, within the spinal canal (Fig. 32-7). At the midbody level, it is relatively narrow, but it widens considerably at the level of the disc before narrowing again as it transitions to the level below. It is adherent at the level of the end plate and anulus but elevated from the concave dorsal surface of the midvertebral body. Along the lateral margins of the PLL, there are often areas of adhesion with the underlying dura. Although the PLL’s contribution to spine stability is modest, it serves to direct disc herniations dorsolaterally, away from the central portion of the spinal canal.
Pedicle
Throughout the thoracic spine, the rostral surface of the pedicle is typically flush with the apophyseal ring of the superior end plate. In the upper and midthoracic regions, the caudal pedicle surface inserts at approximately the midplane of the vertebral body. The intervertebral foramen at these levels is formed almost exclusively by the inferior vertebral notch; the superior vertebral notch is small or nonexistent. By the lower thoracic spine, a relative increase in pedicle height has resulted in the caudal pedicle surface inserting somewhat lower, in plane with the lower one third of the vertebral body. In the lumbar spine, the pedicle is positioned progressively lower on the vertebral body and the superior vertebral notch is more pronounced.
Transverse pedicle width, rather than pedicle height, is the dimension that limits the size of a pedicle screw that can be placed at a given level. In the thoracic spine, pedicle height is commonly double that of pedicle width. Pedicle width is smallest in the region of T4-6, but increases only minimally until one arrives at the thoracolumbar junction. A graphical depiction of changes in these two dimensions across the neuraxis is shown in Figures 32-8 and 32-9.
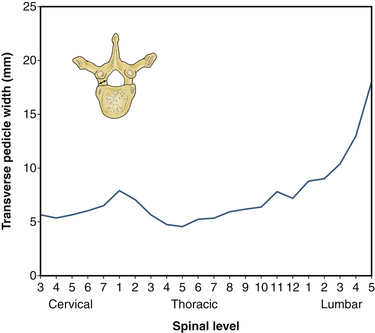
FIGURE 32-8 Transverse pedicle width by level.
(Benzel EC: Biomechanics of spine stabilization, New York, 2001, Thieme, p 6, reprinted with permission.)
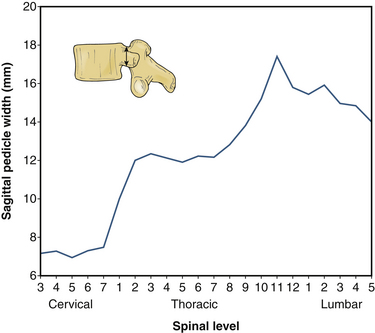
FIGURE 32-9 Sagittal pedicle width by level.
(Benzel EC: Biomechanics of spine stabilization, New York, 2001, Thieme, p 6, reprinted with permission.)
For the same reason that pedicle width constrains screw placement more than pedicle height does, transverse pedicle angle is a more relevant anatomic variable than is sagittal angle in the placement of thoracic pedicle screws. If a screw’s medial-lateral trajectory differs from that of the pedicle by even a relatively small amount, medial or lateral breach may result. Transverse pedicle angle declines fairly steadily as one proceeds down the thoracic spine until a nearly “straight-ahead” trajectory is encountered in the lower thoracic vertebrae; it then increases steeply across the lumbar levels, such that the L5 pedicle has a transverse angle of 25 to 30 degrees (Fig. 32-10).
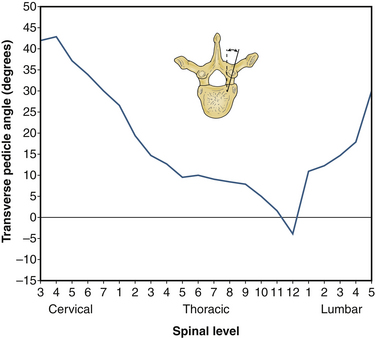
FIGURE 32-10 Transverse pedicle angle by level.
(Benzel EC: Biomechanics of spine stabilization, New York, 2001, Thieme, p 6, reprinted with permission.)
Transpedicular instrumentation in the thoracic spine is more challenging than that in the lumbar spine because of the presence of the adjacent spinal cord, the smaller pedicle diameter, and the relative proximity to neural structures (see Figs. 32-8, 32-9, and 32-14). In the lumbar spine, there is approximately 1.5 mm of epidural space between the medial pedicle wall and the thecal sac.2 In the thoracic spine, the medial pedicle border is contiguous with the edge of the thecal sac.3 In the lumbar spine, the distance from the upper edge of the pedicle to the nerve root above is approximately 5 mm, and the distance from the lower edge of the pedicle to the nerve root below, approximately 1.5 mm.2 In the thoracic spine, the distance from the upper edge of the pedicle to the nerve root above is approximately 2 to 4 mm and the distance from the lower edge of the thoracic pedicle to the nerve root below is approximately 2 to 3 mm.3
Facet and Pars Interarticularis
The facet joint, or zygapophyseal joint, is formed by an inferior articulating process emanating from the level above and a superior articulating process emanating from the level below. The facet joint is a synovial joint, possessing a true joint capsule. This capsule has two layers: an outer layer, composed of parallel bundles of collagenous fibers, and an inner elastic layer, similar in composition to the ligamentum flavum.4 Gliding articulation at the facet joint is limited by the capsular fibers, which are relatively lax in the cervical region but increase in tautness along the rostral caudal axis.
The facet joint functions primarily as a motion-limiting structure; only in extension does it function in an axial load-bearing capacity. The way in which a facet joint constrains motion depends on the alignment of its articulating surfaces (see Fig. 32-2). Lumbar facets occupy a plane that is intermediate between the sagittal and coronal planes. In the lumbar spine, there is a progression from relatively sagittal (25 degrees from sagittal) at L1-2 to relatively coronal (50 degrees from sagittal) at L5-S1. To the surgeon approaching the dorsal lumbar spine, the entry into the facet joint will be found progressively more laterally as one descends toward the lumbosacral junction. This more coronal orientation at the lumbosacral junction is an important element in preventing spondylolisthesis. Thoracic facets have an alignment that is oblique to all three cardinal planes; the articulating surface faces dorsally and slightly superolaterally. Thus, the superior articulating process is positioned relatively medial to the inferior articulating process; this is in contrast to the lumbar spine, where the superior articulating process occupies a lateral position. The transition from thoracic facet orientation to upper lumbar orientation occurs abruptly at the thoracolumbar junction.
Transverse Process
The transverse processes of the thoracic spine and the lumbar spine are relatively thin, consisting of both cortical and cancellous bone. In the thoracic spine, the transverse process projects dorsolaterally and articulates at its tip with the like-numbered rib. In the lumbar spine, the transverse processes project straight laterally and are easily fractured during wide dorsal exposure for dorsolateral fusion. The transverse processes of T11 and T12 are hypoplastic relative to their neighbors and do not articulate with their corresponding ribs. Commonly, the T11 transverse process is a dorsolaterally directed bony stump. In lieu of a T12 transverse process, there are only three small tubercles: a superior tubercle, which is equivalent to the lumbar mammillary process; an inferior tubercle, which is equivalent to the lumbar accessory process; and a lateral tubercle, which represents a very small equivalent of a transverse process. Variation in transverse process morphology can be seen in Figure 32-2.
Rib Articulations of the Thoracic Spine
The relationship of each rib to its corresponding vertebral body changes along the rostral-caudal axis of the thoracic spine (see Fig. 32-1). The 1st rib articulates exclusively with T1 via a single, complete facet located at the lateral aspect of the vertebral body. The 2nd through 9th ribs articulate with their like-numbered vertebrae, as well as the vertebra below, via paired demifacets. These demifacets are also located on the lateral aspect of the vertebral body but in a progressively dorsal position. By the lower thoracic spine (T10-12), two important changes have occurred, First, the pattern of articulation has returned to that of a single, complete, unpaired facet; second, the gradual dorsal movement of the articulation has resulted in the joint being located on the lateral surface of the pedicle, not the vertebral body. However, the size of the rib head and its joint capsule are such that ventrolateral access to the disc space or neural foramen inevitably requires removal of some or all of the adjacent rib head, regardless of level (Fig. 32-11). Because the parietal pleura obscures much of the relevant anatomy, palpation of the rib head is an important orienting maneuver during transthoracic approaches to the spine; often the only recognizable structure that is visible through the pleura is the sympathetic chain, coursing over the rib heads (Fig. 32-12).
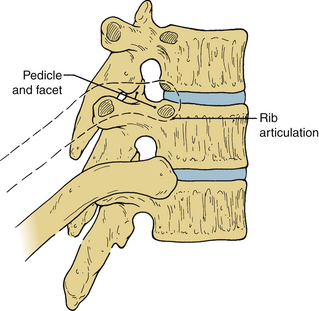
FIGURE 32-11 Rib removal is required for access to the thoracic neural foramen and intervertebral disc.
Lamina and Spinous Process
In general, the laminae are arranged in a shingle-like array, in which the lamina above overlaps the one below (see Fig. 32-1). During laminectomy, the deeper position of the more rostral portion of the lamina is readily appreciated. In the thoracic spine and the lumbar spine, the pars interarticularis consists of the lateralmost portion of the lamina. This is the portion of lamina that resides between, and functionally connects, the superior articulating process and the inferior articulating process. The pars interarticularis is important for spine stability; without it, the stabilizing effect of the facet joint below is lost.
Ligamentum Flavum
The ligamentum flavum is a thick, yellow ligament that goes from the sacrum to C2. Its characteristic appearance and consistency result from a relatively increased ratio of elastic to collagen fibers. The ligamentum flavum is discontinuous, emanating from the rostral surface of the lamina below, spanning the interlaminar space, and inserting on the ventral surface of the lamina above (Fig. 32-13). It is absent along the rostral half of the ventral laminar surface. This has practical significance for the surgeon who is relying on the ligamentum flavum to help prevent incidental durotomy during laminectomy for lumbar stenosis. The ligamentum flavum has two layers, between which exists a virtual, “gliding” space; the outer layer is discontinuous in the midline.5 Even in cases of spondylosis, in which the ligamentum flavum has assumed an exuberant or redundant posture, careful inspection will reveal a midline cleavage plane. This can be a useful point of entry in attempting the safe division of this structure without violation of the dura below.
Interspinous and Supraspinous Ligaments
The interspinous and supraspinous ligaments form a single sheet of soft tissue support in the midline (see Fig. 32-13). The interspinous ligaments span one motion segment; they originate along the superior ridge of the spinous process below and insert at the base of the spinous process above. The supraspinous ligament occupies a position superficial to the tips of the spinous processes. It has two fiber layers: a deep layer that spans one or two motion segments and a superficial layer that may span several segments. Because of their distance from the instantaneous axis of rotation, the interspinous and supraspinous ligaments serve a valuable role as a tension band. In surgeries in which complete laminectomy is not performed, preservation of these ligaments can reduce the likelihood of iatrogenic postoperative kyphotic deformity.
Neural Anatomy
Spinal Segmentation
During fetal development and childhood, the vertebral column grows faster than the spinal cord, resulting in ascension of the conus medullaris within the spinal canal. In the normal adult, the conus typically terminates at L1, though it may occupy positions ranging from T11-12 to the upper vertebral body of L3.6 Fig. 32-15 depicts the normal discordance between spinal level (the level defined by spinal rootlets’ origin) and vertebral level (the level of the neural foramen). A fracture-dislocation in the upper and midthoracic spine will typically cause cord injury at the level of injury or one level below. A fracture-dislocation in the lower thoracic spine would be expected to cause cord injury two or more levels caudal to the bony injury. In both cases, concomitant injury to descending nerve roots may result in a relative concordance between bony injury and its associated neurologic syndrome.
Spinal Cord
The gray matter of the spinal cord is divided into a ventral motor portion and a dorsal sensory portion. The ventral horns contain the cell bodies of lower motor neurons, while the dorsal horns contain the cell bodies of second-order sensory neurons; interneurons can be found in both. Major white matter tracts are depicted in Figure 32-16. Somatotopy of white matter tracts is usually such that fibers to rostral segments are positioned medial to fibers to more caudal segments; an important exception is the dorsal columns, in which fibers to more caudal segments are positioned medially.
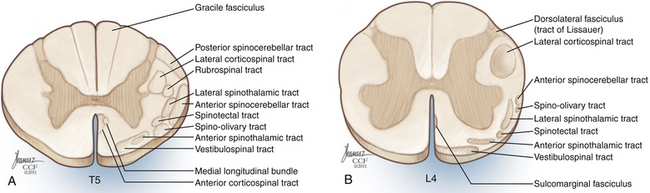
FIGURE 32-16 Spinal cord tracts. A, Thoracic cross section; B, lumbar cross section.
(Copyright Cleveland Clinic Foundation.)
The diameter of the spinal cord increases in two regions, known as the cervical enlargement and the lumbosacral enlargement (see Fig. 32-15). The cervical enlargement results from the increased number of cell bodies present in gray matter innervating the upper extremities; it is found at vertebral levels C4-T1. The lumbosacral enlargement results from the expansion of gray matter at levels innervating the lower extremities and is found at vertebral levels T9-12.
Lateral Recess, Neural Foramen, and Intraforaminal Root
At the lateral border of the thecal sac, each root becomes ensheathed in dura. In the lumbar spine, this shoulder angles obliquely downward to pass below the like-numbered pedicle; in so doing, it crosses the plane of the intervertebral disc. In the cervical spine, by contrast, the nerve takes a relatively horizontal course to its corresponding foramen (above the like-numbered pedicle). This has significance during dorsal operations for dorsolateral disc herniation. During cervical foraminotomy/discectomy, the disc fragment is typically located within the root axilla and can be found with gentle elevation of the nerve root, while during lumbar laminotomy/discectomy, the disc fragment is usually located at the shoulder and is uncovered with medially directed traction on the shoulder. In the upper and midthoracic spine, the course of the ensheathed nerve root is more complicated; anatomic studies have shown downward angulation of the intradural root, followed by upward angulation after the root enters the dural sleeve, followed again by downward angulation once the root has left the neural foramen.7
Before entering the neural foramen, a nerve root traverses the lateral recess. Though it is been variously defined by different authors,8–10 the most intuitive explanation of the lateral recess is a space defined medially by the edge of the thecal sac and laterally by the medial pedicular plane at the level of the midvertebral and lower vertebral body (Fig. 32-17). In the lumbar spine, it is located ventral to the ligamentum flavum and facet complex, dorsal to the vertebral body, and just rostral and medial to the neural foramen. Lateral recess stenosis results from spondylotic redundancy of the ligamentum flavum and facet hypertrophy.
The neural foramen is a space defined rostrally by the pedicle, caudally by the pedicle, ventrally by the inferior portion of the vertebral body and the intervertebral disc, and dorsally by the superior articular process of the level below, with its capsular covering (Fig. 32-18; see also Fig. 32-17). The dimensions of the neural foramen are greater on the rostral-caudal axis than on the ventral-dorsal axis. In the lumbar spine, the neural foramen is approximately 12 to 19 mm high but only 6 to 8 mm wide in the sagittal plane. Thus, the nerve is particularly susceptible to compression from in front (the intervertebral disc) and behind (the superior articular process of the level below). Symptomatic foraminal stenosis commonly results from loss of disc height with secondary impaction of the superior articular process into the neural foramen. Since the height of the neural foramen is commonly two or three times its width, neural compression is usually the result of narrowing in the dorsal-ventral axis rather than the rostral-caudal axis. Restoration of disc height may alleviate symptoms, but this is more commonly the result of an associated increase in foraminal sagittal width than foraminal height per se.
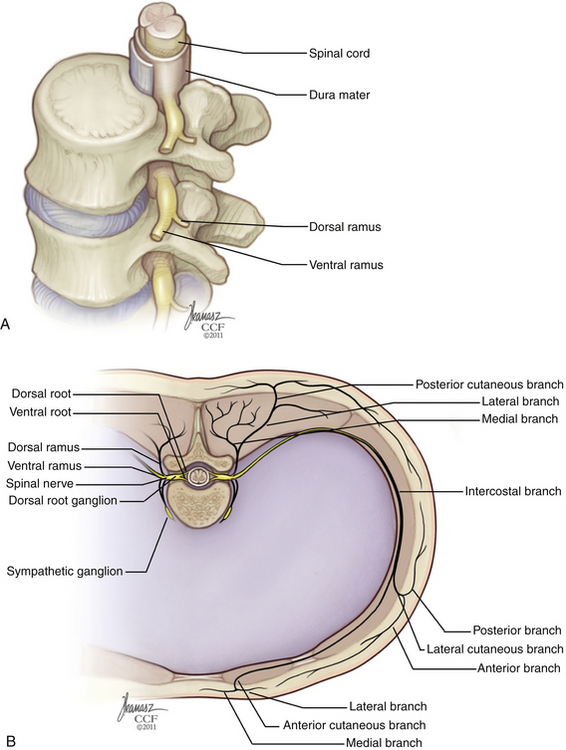
FIGURE 32-18 A, Lateral view of lumbar neural foramen. B, Thoracic cross section showing distal nerve branches.
(Copyright Cleveland Clinic Foundation.)
The origin of the spinal nerve is defined by the union of the dorsal and ventral roots. This usually occurs within the neural foramen, after the roots have entered a common dural root sleeve. The dorsal root ganglion is located distally on the dorsal root and usually occupies a foraminal position. Variation is common, however, and a significant percentage of ganglia are located proximal or distal to the neural foramen.
Extraforaminal Spinal Nerve
After leaving the neural foramen, the spinal nerve bifurcates into ventral and dorsal primary rami (Fig. 32-19). In the thoracic spine, the ventral primary ramus is called the posterior intercostal nerve. This nerve travels laterally and joins the intercostal neurovascular bundle of the like-named rib. Thus, the ninth thoracic spinal nerve exits through the T9-10 foramen and gives rise to the ninth posterior intercostal nerve, which joins the intercostal bundle beneath the 9th rib. In the lumbar spine, the spinal nerve travels obliquely caudally and laterally between the transverse processes, ventral to the intertransverse muscles and ligaments. Bifurcation into ventral and dorsal primary rami occurs at the level of the intervertebral disc. The lumbar ventral primary rami continue along the oblique course of the spinal nerve and make up the lumbar and lumbosacral plexi.
Innervation of the Spine
As the medial branch of the dorsal primary ramus travels along the dorsal elements of the next caudal level, it gives off branches to the facet capsule, periosteum of the neural arch, and dorsal ligaments. Each medial branch gives off twigs to the adjacent and subadjacent facet joints. Thus, the medial branch of the L2 dorsal primary ramus supplies the L2-3 facet joint via proximal zygapophyseal nerves and the L3-4 facet joints via the distal proximal zygapophyseal nerves.11
The sinuvertebral nerve (nerve of Luschka or ramus meningeus) is a recurrent nerve that may originate from the distal margin of the dorsal root ganglion, the spinal nerve, or the rami communicantes (Fig. 32-20). It then passes, either singly or in multiple fibers, back through the rostral portion of the neural foramen to innervate the intervertebral discs above and below, as well as the ventral dura (see Fig. 32-16).12 Encapsulated as well as nonencapsulated nerve endings have been demonstrated in these terminal branches, suggesting possibly a proprioceptive function as well as a nociceptive function.
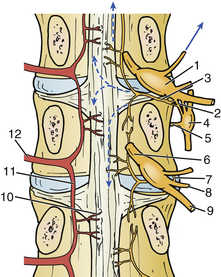
FIGURE 32-20 Sinuvertebral nerve.
1, dorsal root ganglion; 2, rami communicantes; 3, sinuvertebral nerve and its origin; 4, autonomic ganglion; 5, nerve to anterior longitundinal ligament; 6, spinal nerve roots; 7, sinuvertebral nerve arising from distal pole of ganglion; 8, dorsal primary ramus of spinal nerve; 9, ventral primary ramus of spinal nerve; 10, arteries entering basivertebral sinus to supply cancellous bone; 11, descending dorsal central branch of vertebromedullary (spinal) artery; 12, ventral branch of vertebromedullary artery. (From Herkowitz H, editor: Rothman-Simeone the spine, Philadelphia, 2011, Saunders, p 31.)
Anomalous Root Anatomy
Anomalies of nerve roots are relatively common, occurring in over 10% of nerve roots studied.13 Intradural connections, or conjoined roots, are more common than extradural anastomoses, which often have no demonstrable neural connection when viewed histologically. Additionally, roots may have anomalous relationships with their respective foramen, exiting low in the foramen or through a different foramen altogether. Figure 32-21 depicts a variety of anomalies demonstrated in one oft-cited cadaveric study.13 The possibility of anomalous anatomy should be considered when there is discordance between radiographic and clinical findings.
Vascular Anatomy
Middle Thoracic, Lower Thoracic, and Lumbar Arterial Supply: The Segmental System
The descending aorta begins on the left side of the fourth thoracic vertebra and descends ventromedially to reside on the ventral surface of the fourth lumbar vertebra. Just below the fourth lumbar vertebra, the aorta bifurcates into the common iliac arteries. The orifices of the segmental vessels are positioned on the right side of the aorta in the upper thoracic spine, but as the aorta assumes a more ventral, midline position with its caudal descent, the orifices move dorsomedially, so in the lumbar spine, they occupy a midline position on the dorsal wall (Fig. 32-22).
There is variation in the rostral-caudal course of the segmental arteries from level to level (Fig. 32-23).14 Arteries that originate in the middle thoracic spine have a steeper upward trajectory, crossing as many as two vertebral bodies before reaching their final segmental position. This angle is reduced as the aorta descends. In the lower thoracic and upper lumbar spine, segmental arteries usually ascend one level. At the third and fourth lumbar levels, they course horizontally. Each segmental vessel ultimately courses laterally over the midportion of the destined vertebral body.
The segmental arteries have three major branches (Fig. 32-24). Ventral to the neural foramen, each segmental artery branches into a dorsal (or middle) and a lateral branch. One or more spinal (or medial) branches will arise from either the parent segmental artery or the dorsal or lateral branches. The spinal branch courses into the neural foramen and gives rise to four types of vessels: (1) posterior central branches, which remain in the epidural space, supplying the dorsal vertebral body and PLL; (2) prelaminar branches, also epidural, which supply the inner surface of the lamina and ligamentum flavum; (3) dural branches, which supply the dura of the root sleeve and adjacent spinal dura; and (4) neural branches, which supply the roots and spinal cord. The foraminal spinal branch should not be confused with the anterior and posterior spinal arteries, which are major intradural arteries supplying the spinal cord.
Spinal Cord Arterial Supply
The arterial system of the spinal cord consists of a single anterior spinal artery and two paired posterior spinal arteries, all of which are variable in diameter along the length of the spinal cord. The anterior and posterior spinal arteries are supplied by radiculomedullary arteries that are variable in number and location. Branches of this arterial network may be broadly divided into two systems (Fig. 32-25). On the surface of the spinal cord, the pial arterial plexus (or corona radiata or vasa corona) participates in a pial, or periaxial, anastomotic network that connects the anterior and posterior spinal arteries, supplying peripheral white matter tracts. The gray matter of the spinal cord, including both dorsal and ventral horns, is principally supplied by central arteries (sulcal branches, or arteriae sulcocommissurales) emanating from the anterior spinal artery. Though intra-axial anastomoses do exist in the form of vertical connections between adjacent central arteries, these are small in caliber and of limited functional significance.
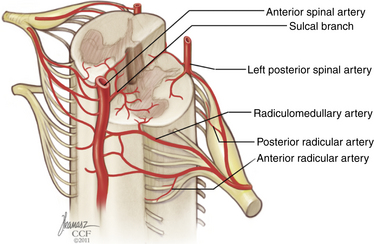
FIGURE 32-25 Arterial supply of the spinal cord and proximal nerve roots.
(Copyright Cleveland Clinic Foundation.)
Three regions of the spine may be distinguished on the basis of the richness of arterial supply and anastomosis. The superior or cervicothoracic area encompasses the cervical and upper thoracic spinal cord and thus includes the cervical enlargement. It is supplied by multiple branches of the vertebral arteries, the deep cervical artery, and the ascending cervical artery. The anterior spinal artery is usually fairly robust in size. The intermediate, or midthoracic, zone (T4-8) has a sparser arterial supply. It is supplied by radiculomedullary vessels from the segmental system, which are inconsistent in number and size; often only one major radiculomedullary vessel supplies this zone. The anterior spinal artery is usually of a smaller diameter and may be absent. The lower, or thoracolumbar, zone (T9 to conus) includes the lumbar enlargement and benefits from a more robust segmental supply compared to the midthoracic zone. The principal radiculomedullary artery to this zone is known as the artery of Adamkiewicz; its inadvertent occlusion may result in paraplegia. The anterior spinal artery is relatively large in the lower zone, and it participates in a constant circumferential arterial anastomosis with the posterior spinal arteries at the level of the conus medullaris.15
The richness of anastomotic connections within each of the three zones is also variable. Compared with the upper and lower zones, the midthoracic region has a dearth of periaxial and intra-axial anastomoses. The central arteries emanating from the anterior spinal artery are fewer in number and smaller in diameter.15 Thus, the midthoracic region is vulnerable as a watershed zone during prolonged periods of global ischemia.
Artery of Adamkiewicz
The artery of Adamkiewicz (AA) is the largest radiculomedullary artery, possessing a diameter of 0.5 to 0.8 mm, which is comparable to that of the anterior spinal artery. It anastomoses with the anterior spinal artery, following a characteristic hairpin loop that is visible on an anteroposterior projection during spine angiography. Ligation or occlusion of the AA will commonly result in spinal cord infarction. The artery typically arises at a variable level between the ninth intercostal artery and the second lumbar artery (in 85% of cases), most commonly on the left side (in approximately 75% of cases)16,17; however, its origin is sufficiently variable that individual radiologic investigation is necessary when its course must be determined preoperatively. Within the foramen, the AA is found in the rostral or middle portion, ventral to the dorsal root ganglion–ventral root complex, where it pierces the dura before joining the ventral root.16
Nerve Root and Cauda Equina Arterial Supply
The arterial supply of nerve roots follows a common scheme. Proximal radicular arteries emanate from the spinal cord’s arterial system and supply the portion of the root closest to the cord; dorsal proximal radicular arteries course along the dorsal roots and are usually direct branches of the posterior spinal artery, and ventral proximal radicular arteries are derived from the pial plexus and course along the ventral roots. The root’s distal portion is perfused by distal radicular arteries emanating from the segmental arterial system. The intervening middle portion of each root relies on a series of progressively smaller anastomotic vessels between these two systems. Radiculomedullary vessels, such as the AA, that traverse the entire length of the root and anastomose directly with the anterior or posterior spinal arteries are usually not significant contributors to root perfusion and often travel in a separate pial investment from the radicular arteries.18
Roots that make up the cauda equina are considerably longer than their rostral counterparts. As a result, there is a longer region in the midportion of each root with a less robust vascular supply. This may have relevance in the clinical entities of neurogenic claudication and cauda equina syndrome. In both, the time dependency of symptoms suggests reversible, or potentially reversible, ischemia of nerve roots. Some authors have suggested that the smaller vessels that predominate in the roots’ middle portions may be more vulnerable to traction or compression.18,19
Venous Drainage of the Spinal Cord and Cauda Equina
The vertebral column is drained by a single large venous plexus. The veins are valveless, permitting bidirectional flow within the system; the assignation of different names to different “plexi” within the system belies its true nature as a single functional unit. The term Batson plexus, which alludes only to the epidural component, is somewhat misleading. Nonetheless, the use of a terminology based on anatomic location is useful. The venous drainage of the spine can be subdivided into an anterior external venous plexus, located along the ventral surface of the vertebral body; the anterior interior venous plexus, located in the epidural space ventral to the thecal sac; the posterior internal venous plexus, located in the epidural space dorsal to the thecal sac; and the posterior external venous plexus, located along the dorsal surfaces of the laminae, facets, and spinous processes. Basivertebral veins radiate through the vertebral body, connecting the anterior internal and anterior external plexi. Dorsally, venous channels traverse the laminae as well. The anterior and posterior internal vertebral venous plexi both resemble ladders, with laterally positioned longitudinal veins connected by transverse anastomoses (Fig. 32-26). In the anterior internal vertebral venous plexus, these anastomoses occur at the level of the midbody and include a connection with the corresponding basivertebral vein. In the posterior internal vertebral venous plexus, transverse anastomoses are found at the level of the lamina. Robust anastomoses connect the vertebral plexus with multiple other venous elements, including the inferior vena cava and segmental veins, the azygous system, the sacral and pelvic plexi, the prostatic plexus, and the intracranial dural sinuses.
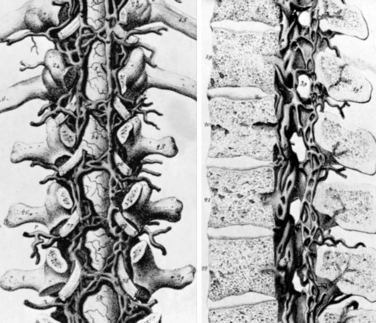
FIGURE 32-26 Anterior and posterior internal epidural venous plexi.
(From Herkowitz H, editor: Rothman-Simeone the spine, Philadelphia, 2011, Saunders, p 41. Permission granted by Dr. Rothman.)
The clinical significance of this system is threefold. First, it can be a source of considerable troublesome bleeding, particularly in the setting of raised intrathoracic or intra-abdominal pressure. Second, because of its low resistance, its relative proximity to the central venous system, and its distensibility, the spinal epidural venous system contributes to the normal ebb and flow of cerebrospinal fluid between the cranial and spinal compartments. With a Valsalva maneuver, the spinal epidural veins become distended, raising cerebrospinal fluid pressure in the spinal compartment and forcing the fluid cranially. In the presence of obstruction at the foramen magnum, this may contribute to the development of syringomyelia.20 Third, because of its valveless connection with other venous systems, the vertebral plexus is a common source of hematologic spread of malignancy and probably accounts for the relatively high incidence of vertebral metastasis.
The intradural venous drainage of the spinal cord, while in continuity with the vertebral plexus via segmental radiculomedullary veins, should be considered as a separate functional unit (Fig. 32-27). Like radiculomedullary arteries, the radiculomedullary veins are inconsistent from level to level, reducing the capacitance of flow between the intradural and epidural systems. Although these radiculomedullary veins are valveless, there are multiple functional obstructions to venous reflux from the epidural system to the intradural system. These include intradural venous folds, a meandering configuration, stenosis at the point of dural penetration, and increased smooth muscle fiber within the radicular veins, which may have autoregulatory function.21 The spinal cord is thus protected from the deleterious effects of venous hypertension except in severe circumstances.
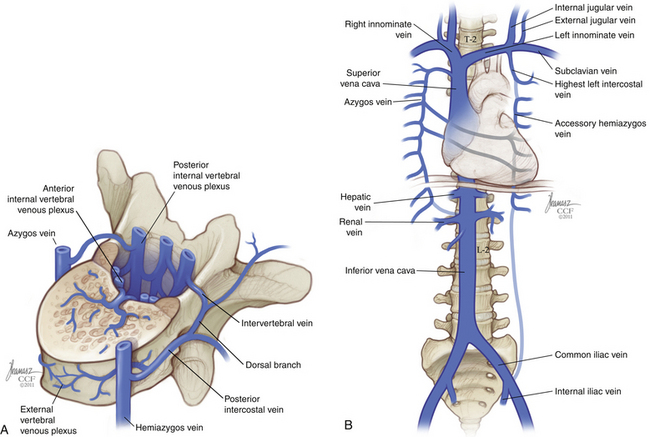
FIGURE 32-27 A, Internal and external vertebral venous plexi. B, Major venous structures and their relationship to the spine.
(Copyright Cleveland Clinic Foundation.)
Venous drainage of the spinal cord occurs via a radially arranged system, which transmits venous blood from the central portion of the cord into a pial venous plexus on the cord’s surface. An anterior spinal vein and a posterior vein are located near the midline of the ventral and dorsal surfaces of the spinal cord, respectively. The pial plexus and anterior and posterior spinal veins drain along radiculomedullary veins located in the dorsal or ventral roots. While a radicular or radiculomedullary artery travels on the root’s surface, the radiculomedullary vein resides within the root, nestled between nerve fasciculi. The radiculomedullary or distal radicular vein usually pierces the dura in concert with the spinal nerve, though a separate aperture may be found in as many as 40% of cases; the transdural segment of vein may be as long as 1 cm.22
Understanding the specific vascular anatomy of the nerve root is of particular importance in the surgical management of spinal dural arteriovenous fistula, or type 1 spinal arteriovenous malformation. Dural arteriovenous fistulae form between a dural artery and a radicular or radiculomedullary vein, resulting in venous hypertension in the pial plexus with secondary myelopathy. Microanatomic study has shown that the dural artery will usually send multiple small branches into the dura of the root sleeve at the junction of the radicular dura and the spinal dura. These will then coalesce again in the outer dural layer to form a single arterial branch that traverses the inner dural layer to fistulize with a radiculomedullary vein at the inner surface of the dura.23 Thus, the lesion is usually intradural and represents a fistulization between an extradural artery and an intradural vein. Because multiple dural arteries may participate in such a fistula, treatment is usually directed at surgical or endovascular occlusion of the final common pathway: the intradural radiculomedullary vein.
Anatomic Variation and Surgical Localization
Surgery in the thoracic spine is often based on counting ribs on an anteroposterior or posteroanterior radiograph. Confusion may arise when a patient has more than the normal number of ribs, fewer than the normal number of ribs, or elongated transverse processes that are erroneously counted as ribs. Cervical ribs are estimated to occur with a prevalence of 0.05%24 to 6%.25 Elongated transverse processes are estimated to occur with a prevalence of 2.2%.24 The prevalence of thoracic rib aplasia is approximately 6%, and the prevalence of lumbar rib is approximately 1%.25 The overall prevalence of rib number abnormality is estimated to be approximately 8%26; disparate results reported in the literature may reflect differences in incidence among different ethnic populations.
Lumbosacral transitional vertebrae occur when lumbar segments are “sacralized” (incorporated into the sacrum as nonmobile segments) or when sacral segments are “lumbarized” (resulting in more than five mobile vertebrae between the sacrum and the thoracic spine). Transitional vertebrae are relatively common. A recent study of 750 consecutive patients undergoing whole-spine MRI found that 20% exhibited transitional vertebrae: 14.5% had six lumbar-type vertebrae, 5.3% had four lumbar-type vertebrae, and 0.13% had three lumbar-type vertebrae.27 There is an association between the presence of a cervical rib and a lumbosacral transitional vertebra.28
Alleyne C.H.Jr., Cawley C.M., Shengelaia G.G., Barrow D.L. Microsurgical anatomy of the artery of Adamkiewicz and its segmental artery. J Neurosurg. 1998;89:791-795.
Jenis L.G., An H.S. Spine update. Lumbar foraminal stenosis. Spine (Phila Pa 1976). 2000;25:389-394.
Lazorthes G., Gouaze A., Zadeh J.O., et al. Arterial vascularization of the spinal cord. J Neurosurg. 1971;35:253-262.
Parke W.W., Gammell K., Rothman R.H. Arterial vascularization of the cauda equina. J Bone Joint Surg [Am]. 1981;63:53-62.
van der Kuip M., Hoogland P.V., Groen R.J. Human radicular veins: regulation of venous reflux in the absence of valves. Anat Rec. 1999;254:173-180.
1. Hou Y, Luo Z. A study on the structural properties of the lumbar endplate: histological structure, the effect of bone density, and spinal level. Spine (Phila Pa 1976). 2009;34:E427-E433.
2. Ebraheim NA, Xu R, Darwich M, Yeasting RA. Anatomic relations between the lumbar pedicle and the adjacent neural structures. Spine (Phila Pa 1976). 1997;22:2338-2341.
3. Ebraheim NA, Jabaly G, Xu R, Yeasting RA. Anatomic relations of the thoracic pedicle to the adjacent neural structures. Spine (Phila Pa 1976). 1997;22:1553-1556. discussion 1557
4. Yamashita T, Minaki Y, Ozaktay AC, et al. A morphological study of the fibrous capsule of the human lumbar facet joint. Spine (Phila Pa 1976). 1996;21:538-543.
5. Viejo-Fuertes D, Liguoro D, Rivel J, et al. Morphologic and histologic study of the ligamentum flavum in the thoraco-lumbar region. Surg Radiol Anat. 1998;20:171-176.
6. Soleiman J, Demaerel P, Rocher S, et al. Magnetic resonance imaging study of the level of termination of the conus medullaris and the thecal sac: influence of age and gender. Spine (Phila Pa 1976). 2005;30:1875-1880.
7. Nathan H, Feuerstein M. Angulated course of spinal nerve roots. J Neurosurg. 1970;32:349-352.
8. Crock HV. Normal and pathological anatomy of the lumbar spinal nerve root canals. J Bone Joint Surg [Br]. 1981;63:487-490.
9. Lee CK, Rauschning W, Glenn W. Lateral lumbar spinal canal stenosis: classification, pathologic anatomy and surgical decompression. Spine (Phila Pa 1976). 1988;13:313-320.
10. Jenis LG, An HS. Spine update. Lumbar foraminal stenosis. Spine (Phila Pa 1976). 2000;25:389-394.
11. Bogduk N, Long DM. The anatomy of the so-called “articular nerves” and their relationship to facet denervation in the treatment of low-back pain. J Neurosurg. 1979;51:172-177.
12. Bogduk N, Tynan W, Wilson AS. The nerve supply to the human lumbar intervertebral discs. J Anat. 1981;132:39-56.
13. Kadish LJ, Simmons EH. Anomalies of the lumbosacral nerve roots. An anatomical investigation and myelographic study. J Bone Joint Surg [Br]. 1984;66:411-416.
14. Shimizu S, Tanaka R, Kan S, et al. Origins of the segmental arteries in the aorta: an anatomic study for selective catheterization with spinal arteriography. AJNR Am J Neuroradiol. 2005;26:922-928.
15. Lazorthes G, Gouaze A, Zadeh JO, et al. Arterial vascularization of the spinal cord. J Neurosurg. 1971;35:253-262.
16. Alleyne CHJr., Cawley CM, Shengelaia GG, Barrow DL. Microsurgical anatomy of the artery of Adamkiewicz and its segmental artery. J Neurosurg. 1998;89:791-795.
17. Thron AK. Vascular anatomy of the spinal cord: neuroradiological investigations and clinical syndromes. New York: Springer-Verlag; 1988.
18. Parke WW, Gammell K, Rothman RH. Arterial vascularization of the cauda equina. J Bone Joint Surg [Am]. 1981;63:53-62.
19. Murphy RW. Nerve roots and spinal nerves in degenerative disk disease. Clin Orthop Relat Res. 1977;719:46-60.
20. Williams B. The distending force in the production of communicating syringomyelia. Lancet. 1969;2:696.
21. van der Kuip M, Hoogland PV, Groen RJ. Human radicular veins: regulation of venous reflux in the absence of valves. Anat Rec. 1999;254:173-180.
22. Lasjaunias P, Berenstein A. Surgical neuroangiography: functional vascular anatomy of brain, spinal cord, and spine, vol 3. Berlin: Springer-Verlag; 1990.
23. McCutcheon IE, Doppman JL, Oldfield EH. Microvascular anatomy of dural arteriovenous abnormalities of the spine: a microangiographic study. J Neurosurg. 1996;84:215-220.
24. Brewin J, Hill M, Ellis H. The prevalence of cervical ribs in a London population. Clin Anat. 2009;22:331-336.
25. Merks JH, Smets AM, Van Rijn RR, et al. Prevalence of rib anomalies in normal Caucasian children and childhood cancer patients. Eur J Med Genet. 2005;48:113-129.
26. Loder RT, Huffman G, Toney E, et al. Abnormal rib number in childhood malignancy: implications for the scoliosis surgeon. Spine (Phila Pa 1976). 2007;32:904-910.
27. Hanson EH, Mishra RK, Chang DS, et al. Sagittal whole-spine magnetic resonance imaging in 750 consecutive outpatients: accurate determination of the number of lumbar vertebral bodies. J Neurosurg Spine. 2010;12:47-55.
28. Erken E, Ozer HT, Gulek B, Durgun B. The association between cervical rib and sacralization. Spine (Phila Pa 1976). 2002;27:1659-1664.