Chapter 8 Antigen Presentation
• T cells survey proteins derived from intracellular or extracellular pathogens by recognizing peptide fragments that have been processed and become bound to major histocompatibility complex (MHC) class I or II molecules, respectively. These MHC–antigen complexes are presented at the cell surface.
• MHC class I molecules associate with endogenously synthesized peptides, produced by degradation of the cells’ internal molecules. This type of antigen processing is carried out by proteasomes, which cleave the proteins and transporters, which take the fragments to the endoplasmic reticulum (ER).
• MHC class II molecules bind to peptides produced following the breakdown of proteins that the cell has endocytosed. The peptides produced by degradation of these external antigens are loaded onto MHC class II molecules in a specialized endosomal compartment called MIIC.
• The highly ordered area of contact between the T cell and APC is an immunological synapse. TCRs and costimulatory receptors occupy the center of the synapse. Adhesion molecules are found in the periphery.
• Costimulatory molecules are essential for T cell activation. Molecules such as B7 (CD80/86) on the APC bind to CD28 on the T cell to cause activation. Antigens presented without costimulation usually induce T cell anergy. Intercellular adhesion molecules also contribute to the interaction between a T cell and an antigen-presenting cell (APC). Interactions between intercellular cell adhesion molecule-1 (ICAM-1) and leukocyte functional antigen-1 (LFA-1) and between CD2 and its ligands extend the interaction between T cells and APCs.
• CD4 binds to MHC class II and CD8 to MHC class I molecules. These interactions increase the affinity of T cell binding to the appropriate MHC–antigen complex and bring kinases to the TCR complex.
• Binding of CTLA-4 or PD-1 on the T cell limits activation. Both of these ligands inhibit the costimulatory signal that the T cell receives from CD28.
• T cell activation induces enzyme cascades, leading to the production of interleukin-2 (IL-2) and the high-affinity IL-2 receptor on the T cell. IL-2 is required to drive T cell division.
• Antigen presentation affects the subsequent course of an immune response. The immune system responds to clues that an infection has taken place before responding strongly to antigens.
Antigen presenting cells
T cells only recognize antigen peptides bound to MHC-encoded molecules. Endogenous peptides, derived from intracellular sources such as replicating viruses, are presented on MHC class-I molecules to CD8+ T cells, while exogenous peptides, derived from extracellular sources such as microbes, are presented on MHC class-II molecules to CD4+ T cells. Before peptides can associate with the MHC molecules they are generated by partial proteolysis from the original protein antigen. Antigen processing refers to the degradation of antigen into peptide fragments, which may become bound to MHC class I or class II molecules (see Chapter 5). Whether a peptide binds to an MHC molecule depends on the amino-acid sequence of the peptide and on whether a suitable binding MHC molecule is available, which depends on the set of MHC molecules present in the individual. Broadly speaking, a single T cell recognizes a specific peptide(s) bound in the peptide-binding groove of a specific MHC molecule. However there are instances where T cells can respond to a different MHC/peptide combination, and this is equivalent to the cross-reactivity that may occur when antibodies bind to cross-reactive antigens.
• different types of antigen-presenting cell (APC) are brought into play depending on the situation. Dendritic cells (DCs) are particularly crucial for initiating responses;
• the interaction between the TCR, and the MHC/peptide complex is highly specific;
• another level of control is exerted by costimulatory molecules on APCs, resulting in T cell activation only when appropriate, such as in an infection;
• adhesion molecules on the interacting cells also contribute to the stable binding of the cells, which promotes effective antigen presentation;
• signals from the cell surface are then transmitted by a series of signal transduction pathways that regulate gene expression including cytokine production;
• in the final stages the actions of cytokines on the lymphocytes drive cell division.
The four stages of antigen presentation are outlined in Figure 8.1. In lymphoid organs, all four stages of the process can occur, resulting in T cell proliferation. However, antigen presentation can also occur to a more limited degree in tissues, resulting in cytokine production, but with little T cell division.
Interactions with antigen-presenting cells direct T cell activation
Dendritic cells are crucial for priming T cells
The majority of dendritic cells enter lymph nodes via afferent lymphatics. Originally it was thought that these cells were mostly derived from Langerhans’ cells in the skin, but it now appears that a substantial proportion of the early migrating DCs in afferent lymph are dermal dendritic cells (they do not express langerin (CD207) a marker of Langerhans’ cells). Moreover DCs derived from Langerhans’ cells tend to localize in the paracortex of the lymph node, whereas dermal DCs remain near lymphoid follicles. Dendritic cells arriving from the periphery of the body transport antigen to the lymph node and process it for presentation to T cells. A minor proportion of the DCs in lymph nodes, arrive from the blood across the HEV, using the same route as T cells and B cells (see Fig. 6.15), however these cells have not acquired antigen in the periphery and they can only acquire it from lymph or transfer from other cells. As they mature, dendritic cells express CCR7 which allows them to localize to the lymphoid tissues. There is also some evidence that DCs from skin and the gut have distinctive chemokine receptors, which allow them to selectively recirculate to their own lymphoid organs. As they mature, DCs also increase expression of key costimulatory molecules, including CD40, CD80 and CD86 (B7-1 and B7-2).
Macrophages and B cells present antigen to primed T cells
• bind to a specific antigen through surface IgM or IgD;
• then degrade it into peptides, which associate with MHC class II molecules.
The properties and functions of some APCs are summarized in Figures 8.3 and 8.4.
Antigen processing
Antigen processing involves degrading the antigen into peptide fragments. The vast majority of epitopes recognized by T cells are fragments from a peptide chain. Only a minority of peptide fragments from a protein antigen are able to bind to a particular MHC molecule. Furthermore, different MHC molecules bind different sets of peptides (see Chapter 5). For example, the great majority of the immune response in humans against the HIV matrix protein is directed against a single immunodominant region, i.e. one which is recognized by a large number of T cells. However, exactly which part of this region is recognized, depends on the MHC haplotypes of the individual (Fig. 8.5).
MHC class I pathway
• influenza virus hemagglutinin (HA), a glycoprotein associated with the membrane of an infected host cell, normally elicits only a weak CTL response, but influenza virus HA can be generated in the cytoplasm by deleting that part of its sequence that encodes the N terminal signal peptide (required for translation across the membrane of the endoplasmic reticulum [ER]) and, when this is done, there is a strong CTL response to HA;
• the introduction of ovalbumin into the cytoplasm of a target cell (using an osmotic shock technique) generates CTLs recognizing ovalbumin, whereas the addition of exogenous ovalbumin generates an exclusively TH cell response.
Proteasomes are cytoplasmic organelles that degrade cytoplasmic proteins
Although the assembly of MHC class I molecules occurs in the ER of the cell, peptides destined to be presented by MHC class I molecules are generated from cytosolic proteins. The initial step in this process involves an organelle called the proteasome – a multi-protein complex which forms a barrel-like structure (Fig. 8.6).
Two genes, PSMB8 and PSMB9 located in the class II region of the MHC (Fig. 8.7), encode proteasome components that subtly modify the range of peptides produced by proteasomes. The expression of these genes is induced by interferon-γ (IFNγ). The proteins displace constitutive subunits of the proteasome and along with a third inducible proteasome component (PSMB10 encoded on a different chromosome) influence processing of peptides by creating a wider range of peptide fragments suitable for binding MHC class I molecules. Additional subunits associate with the ends of core (20 S) proteasomes and may influence antigen processing. These include interferon-inducible PA28 (proteasome-activator-28) molecules as well as a complex of proteins that result in a larger 26 S particle.
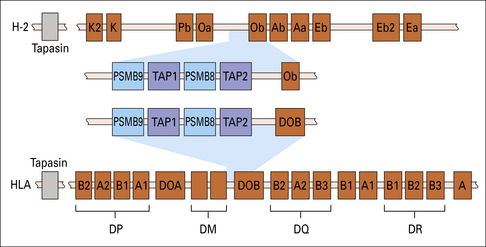
Fig. 8.7 MHC genes involved in antigen processing and presentation
Genes encoding the two subunits of the peptide transporter (TAP) and two components (PSMB8 and 9) of the multisubunit proteasome (see Fig. 8.6) are located in the murine and human class II regions. The Tapasin gene is located just centromeric of the MHC.
Transporters move peptides to the ER
The products of two genes, TAP1 and TAP2, that map in the MHC (see Fig. 8.7), function as a heterodimeric transporter that translocates peptides into the lumen of the ER. TAP is a member of the large ATP-binding cassette (ABC) family of transporters localized in the ER membrane. Microsomes from cells lacking TAP1 or TAP2 could not take up peptide in experiments in vitro. Using a similar system it was shown that the most efficient transport occurred with peptide substrates of 8–15 amino acids. Although this size is close to the length preference of MHC class I molecule binding sites, it suggests that some additional trimming may be required by enzymes in the lumen of the ER, particularly ERAAP (ER-associated aminopeptidase).
A multi-component complex loads peptides onto MHC class-I molecules
MHC class-I α-chains are initially associated with the chaperone, calnexin. Once released from calnexin they bind to β2-microglobulin to form a complete MHC class-I molecule, which is incorporated into a peptide-loading complex associated with the TAP transporters (Fig. 8.8). MHC class I molecule complexes lacking peptide are unstable, ensuring that only functionally useful complexes are available for interaction with TCRs.
Antigen processing affects which peptides are presented
• the efficiency of the proteasome in generating different peptides, which varies if the proteasome contains interferon-inducible components;
• the efficiency of the transporters in taking peptides from the cytosol to the ER;
Each one of these factors also depend on the amino-acid sequence of the original protein (Fig. 8.9), and to some extent on genetic variations in molecules involved in antigen processing (Fig. 8.10). All of these considerations are important in developing vaccines, where the aim is to identify an immunodominant region of a pathogen to stimulate T cells; but it is not enough for a peptide to bind to MHC molecules, it must also be processed properly if it is to be immunogenic.
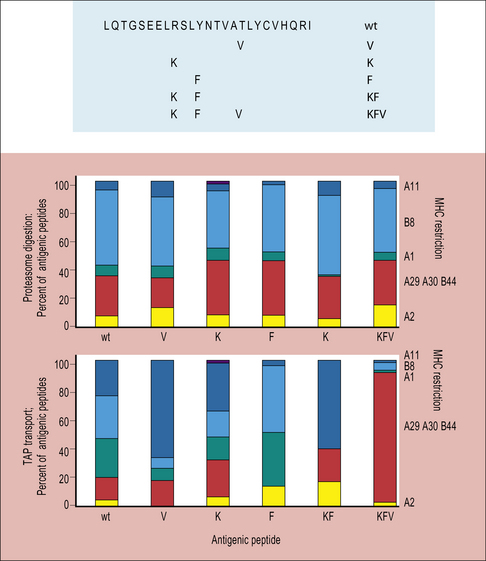
Fig 8.9 Production of antigenic peptides
(Based on data from Tenzer et al., 2009, Nature Immunology, 10, 636–646.)
Some class I-like molecules can present limited sets of antigens
HLA-E-signal peptide complex interacts with the NKG2A inhibitory receptor on NK cells
The HLA-E–signal peptide complex interacts with the NKG2A inhibitory receptor on NK cells (see Fig. 10.6). A cell that expresses HLA-E is therefore not killed by NK cells.
Why are antigen-processing genes located in the MHC?
The finding of a cassette of antigen-processing genes such as PSMBs and TAPs in the class II region of the MHC is striking. There is some evidence, especially from studies in rats, that particular alleles of TAP are genetically linked with alleles of class I genes that are most suited to receive the kind of peptides preferentially transported by the products of that TAP allele (see Fig. 8.10). The clustering of antigen processing and presenting genes in the MHC of most vertebrate species may not be fortuitous. It may help to coordinate co-evolution of some molecules as well as facilitating exchange of sequences between loci.
MHC class II pathway
Class II molecules are loaded with exogenous peptides
MHC class II molecules are produced in the ER, complexed to a polypeptide called the invariant chain (Ii) (encoded outside the MHC), which stabilizes the complex and prevents the inappropriate binding of antigen. The αβ–Ii complex is transported from the Golgi to an antigen processing compartment which appears as a multivesicular body (also called the MIIC compartment), specialized for the transport and loading of MHC class II molecules. The compartment has characteristics of both endosomes and lysosomes with an onion-skin appearance under the electron microscope, comprising multiple membrane structures (Fig. 8.11). The αβ complex spends 1–3 hours in this compartment before reaching the cell surface (Fig. 8.12).
Exogenous antigens reach the MIIC compartment from acidic endosomes, where they have been partly degraded by the actions of proteases and chaperone proteins. The Ii chain is cleaved by cathepsins into small fragments, one of which, termed CLIP (class II-associated invariant peptide), is located in the groove of the class II molecule until replaced by peptides destined for presentation. The exchange of CLIP for other peptides is orchestrated by HLA-DM, an MHC class-II-like chaperone protein, consisting of α and β chains both encoded within the MHC class II region, but which does not itself have a peptide binding site (Fig. 8.13). HLA-DM binds to the αβ-CLIP complex to stabilize it until it has bound a suitable antigenic peptide. In cell lines lacking HLA-DM, the class II molecules are unstable and the cells no longer process and present proteins. Their class II molecules end up at the cell surface occupied by CLIP fragments of the invariant chain.
A further MHC-encoded molecule, HLA-DO (see Fig. 8.7), which associates with DM, regulates peptide loading. Like conventional MHC class II molecules, HLA-DO is a heterodimer, consisting of the DOA and DOB chains.
T cell interaction with APCS
When the T cell encounters the appropriate MHC/peptide, a conformational change in LFA-1 on the T cell, signaled via the TCR, results in tighter binding to ICAM-1 and prolonged cell–cell contact. (Fig. 8.14) The joined cells can exist as a pair for up to 12 hours, and this marks the second phase of interaction. At this stage an ‘immunological synapse’ forms and the T cell may be activated (Fig. 8.15).
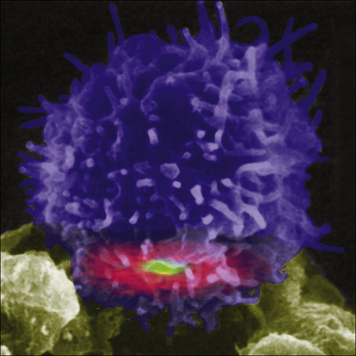
Fig. 8.14 Color-enhanced reconstruction of an immunological synapse
(Courtesy of Dr Mike Dustin and Science.)
The immunological synapse is a highly ordered signaling structure
The interactions between APCs and T cells have been studied extensively in vitro, where the cells form a ‘bulls-eye’ structure at the point of contact (see Fig. 8.14). This ‘immunological synapse’ is thought to reflect, in idealized conditions, the events that occur within a lymph node when T cells and dendritic cells interact; in vitro high doses of antigen may be used, and it is known that the size of the synapse relates to the amount of antigen present.
Costimulation by B7 binding to CD28 is essential for T cell activation
• prolongs and augments the production of IL-2 and other cytokines; and
• is probably important in preventing the induction of tolerance, a condition where the T cell is not activated and is put into a state of anergy, i.e. it is unable to respond subsequently.
Many cells in the tissues can be induced to express MHC class-II molecules and present antigenic peptides to CD4+ T cells, however, they are mostly ineffective in inducing T cell activation and proliferation, because they lack the necessary costimulatory molecules (Fig. 8.16).
Ligation of CTLA-4 inhibits T cell activation
CTLA-4 (CD152) is an alternative ligand for B7, with higher affinity than CD28. It is an inhibitory receptor limiting T cell activation, resulting in less IL-2 production. CTLA-4 appears to act by reducing the time for interaction between the APC and the T cell, so a stronger activation signal is needed otherwise incomplete signaling will occur. As a T cell matures, it expresses higher levels of CTLA-4 and hence requires stronger activation stimuli to continue division (Fig. 8.17).
Intracellular signaling pathways activate transcription factors
TCR-binding activates tyrosine kinases
TCR α and β chains are associated with the CD3γ, δ, and ε molecules, the ζ and η chains and the enzyme Lck (p56lck), which is attached to the intracellular portions of CD4 or CD8 (Fig. 8.w1). (The label p56lck signifies a lymphocyte-specific tyrosine kinase of 56 kDa.) The first steps involve tyrosine kinases of the Src family, particularly Lck and Fyn, which phosphorylate target sequences found in the TCR ζ chain termed immunoreceptor tyrosine-based activation motifs (ITAMs).
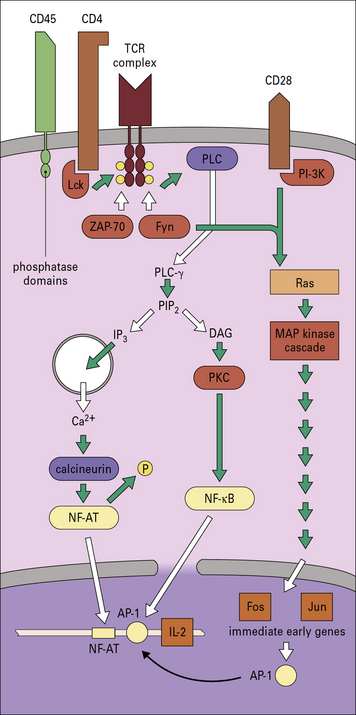
Fig. 8.w1 Intracellular signaling in T cell activation
T cell activation involves the transduction of signals from both the TCR and CD28. Clustering of surface receptors such as TCR, CD4, CD28, and CD45 results in activation of tyrosine kinases Fyn and Lck. CD4, which is associated with the TCR complex, binds to the kinase, Lck. Such kinases become activated by dephosphorylation, possibly by phosphatase domains on CD45 (leukocyte common antigen). Lck can now phosphorylate the ITAM domains on the γ chains of CD3 (see Figs 5.3 and 5.15), which allows them to associate with other kinases including Fyn and ZAP-70. Fyn activates phospholipase C (PLCγ), which leads to two pathways by the cleavage of phosphatidylinositol bisphosphate (PIP2) into diacylglycerol (DAG) and inositol trisphosphate (IP3). IP3 releases Ca2+ from intracellular (ER) stores to activate calcium-dependent enzymes such as calcineurin. Calcineurin removes phosphate from the transcription factor NF-AT (nuclear factor of activation of T cells), which causes its translocation to the nucleus. DAG activates protein kinase C (PKC), which then activates the transcription factor NF-κB. Meanwhile, ZAP-70, Fyn, and PI-3 kinase (PI-3 K) (associated with CD28) integrate signals via kinase cascades in the cytoplasm that activate specific transcription factors. Adapter proteins are used to link the various receptors to the common intracellular signaling components. GTP-binding proteins (G proteins) are involved in activating a set of protein kinases in the MAP kinase cascade. The transcription factors translocate to the nucleus to activate genes, including ‘immediate early genes’ Fos and Jun for cell division and the promoter AP-1, which acts with NF-AT on the IL-2 gene.
Phosphorylation of the ITAMs initiates a series of steps (see Fig. 8.w1), that lead to the activation of transcription factors including NFAT, and NFκB, and their translocation to the nucleus. The IL-2 enhancer contains a binding site for a nuclear factor, NF-AT (nuclear factor of activation of T cells), which also interacts with Fos and Jun, to induce IL-2 gene expression of the IL-2 receptor (IL-2R) gene.
In summary, TCR stimulation results in activation of a variety of tyrosine kinases and downstream effectors that regulate cellular responses, resulting in the regulation of IL-2 gene expression, which is largely responsible for activating the T cell and inducing division. An understanding of the requirements for T cell division has allowed researchers to generate long-lived T cell lines, which are used in many areas of immunological research (Method box 8.1).
Method box 8.1 T cell lines
To test the response of lymphocytes to a specific antigen that is being presented, the lymphocyte stimulation test (Fig. MB8.1.1) may be used. This test measures the response of the T cells to antigen as indicated by their entering the cell cycle and incorporating precursors of DNA synthesis.
Interleukin-2 drives T cell division
On resting T cells, the IL-2R is predominantly present as a low-affinity form consisting of two polypeptide chains, a β chain (p75) that binds IL-2 and a common γc chain that signals to the cell. When the T cell is activated, it produces an α chain (CD25), which contributes to IL-2 binding and, together with the β and γc chains, forms the high-affinity receptor (Fig. 8.18). IL-2 is internalized within 10–20 minutes and the β and γc chains are degraded in lysosomes while the α chain is recycled to the cell surface. Sustained signaling by IL-2 over several hours is needed to drive T-cell division.
In view of the importance of IL-2 in T cell division, it was surprising that the rare patients who lack CD25 (and IL-2 receptor knockout mice) develop an immunoproliferative condition. These observations lead to an awareness that IL-2 also has a regulatory function in T cell development – regulatory T cells (Tregs) are characterized by high CD25 expression, and IL-2 is required for their generation in the thymus and maintenance in the periphery (see Chapter 11).
Types of immune response
• release cytokines such as IFNγ and granulocyte–macrophage colony stimulating factor (GM–CSF);
• express surface molecules such as CD154 which binds to the costimulatory molecule CD40 on antigen presenting cells.
Activation of lymphocytes leads to two partially competing processes:
Danger signals enhance antigen presentation
In other words, foreign substances may be innocuous or invisible to the immune system unless accompanied by danger signals, such as infection. These danger signals are provided by receptors for microbial products on APCs, such as the Toll-like receptors (TLRs, see Fig. 6.21).
Critical thinking: Antigen processing and presentation (see p. 435 for explanations)
1. Why are macrophages used as APCs in this experiment? Would you get the same results if you used infected fibroblasts?
2. Why does the live influenza virus stimulate both clones whereas the inactivated virus stimulates only the MHC class II-restricted clone?
3. Why does emetine prevent the macrophages from presenting antigen to the MHC class I-restricted T cells whereas chloroquine prevents them from presenting to MHC class II-restricted cells?
4. One of these clones expresses CD4 and the other CD8. Which way round is it?
Ackerman A.L., Cresswell P. Cellular mechanisms governing cross-presentation of exogenous antigens. Nat Immunol. 2004;5:678–684.
Bell D., Young J.W., Banchereau J. Dendritic cells. Annu Rev Immunol. 1999;17:255–305.
Berger A.C., Roche P.A. MHC class II transport at a glance. J Cell Sci. 2009;122:1–4.
Boes M., Ploegh H.L. Translating cell biology in vitro to immunity in vivo. Nature. 2004;430:264–271.
Brocke P., Garbi N., Momburg F., Hammerling G.J. HLA-DM, HLA-DO and tapasin: functional similarities and differences. Curr Opin Immunol. 2002;14:22–29.
Cresswell P, Ackerman AL, Giodin A, et al. Mechanisms of MHC class-I restricted antigen processing and cross presentation. Imm Revs 205;207:145–157
Fooksman D.R., Vardhana S., Vasiliver-Shamis G., et al. Functional anatomy of T cell activation and synapse formation. Ann Revs Immunol. 2010;28:79–106.
Kloetzel P.M. Generation of MHC class I antigens: functional interplay between proteasomes and TPPII. Nat Immunol. 2004;5:661–669.
Okazaki T., Honjo T. PD-1 and PD-1 ligands: from discovery to clinical application. Int Immunol. 2007;19:813–824.
Randolph G., Orchando J., Partida-Sanchez S. Migration of dendritic cell subsets and their precursors. Ann Revs Immunol. 2008;26:293–316.
Tenzer S., Wee E., Burgevin A., et al. Antigen processing influences HIV-specific cytotoxic lymphocyte immunodominance. Nat Immunol. 2009;10:636–646.
Watts C., Powis S. Pathways of antigen processing and presentation. Rev Immunogenet. 1999;1:60–74.