CHAPTER 103 ANESTHESIA IN THE SURGICAL INTENSIVE CARE UNIT—BEYOND THE AIRWAY: NEUROMUSCULAR PARALYSIS AND PAIN MANAGEMENT
MUSCLE RELAXANTS
Historic Perspective
One of the first documented uses of a neuromuscular blocking agent (NMBA) to aid in surgical closure of the abdomen occurred in 1912 in Germany.1 In the 1940s, the use of NMBAs during surgery started to become commonplace. Since the 1940s, the safety profile of NMBAs has significantly improved, and their use in the operating room (OR) has become routine. The introduction of mechanical ventilation in the intensive care unit (ICU) was closely followed by the use of NMBAs in the ICU. Most of our clinical experience with the use of NMBAs comes from the care of relatively healthy patients for a finite period of time in the OR. In contrast to patients in the OR, patients in the ICU are typically critically ill with multiple organ systems failing, require prolonged neuromuscular paralysis, and are receiving a large number of concomitant medications. Clinical studies have only recently started to examine indications for and complications of NMBAs in the ICU.
Current Epidemiology
Retrospective surveys reported by intensivists in 1991 and 1992 revealed that 10 patients per ICU per month required prolonged neuromuscular blockade.2 However, a study by Murray et al.3 in 1993 showed the probability of receiving a NMBA ranged from 0% in the neurosurgical ICU to 14% in the neonatal ICU. Several different situations have been identified that require short- or long-term neuromuscular paralysis.
Indications
Despite adequate sedation, some patients will not be able to tolerate mechanical ventilation, which can lead to unsafe peak inspiratory airway pressures. In a retrospective survey, Klessing4 reported that 89% of the cases requiring the use of NMBAs were related to the facilitation of mechanical ventilation, making it the most common reason for the use of neuromuscular paralysis. Other indications for NMBA use are the facilitation of endotracheal intubation, control of increased intracranial pressure (ICP), decrease of high muscle tone in certain medical conditions, and facilitation of needed medical procedures or diagnostic studies5 (Table 1). Short-acting NMBAs such as succinylcholine can be used to quickly secure an unprotected airway. However, many critically ill patients can have an airway secured by endotracheal intubation without the use of NMBAs. In patients with increased ICP, agitation or coughing caused by tracheobronchial suctioning can cause dangerous increases in ICP. Werba et al.6 demonstrated that pretreatment with vecuronium attenuated transient increases in ICP during suctioning.
Table 1 Indications for Use of Neuromuscular Blocking Agents in Intensive Care Unit
Concerns Regarding Overuse of Paralysis
Nevertheless, a retrospective study of 514 patients with severe head injuries demonstrated that the use of NMBAs resulted in longer ICU stays and increased morbidities.7 Symptomatic muscle rigidity found in tetanus, neuroleptic malignant syndrome, and status epilepticus can be ameliorated with NMBAs; however, treating the underlying cause of the symptoms is paramount. In addition, NMBAs can be used to facilitate minor bedside surgical procedures or diagnostic studies when immobility is critical for success, in particular in situations where adequate sedation levels to prevent movement cannot be achieved with analgesics and anesthetics alone, perhaps due to hemodynamic instability.
The long-term use of NMBAs has continued to increase over the last several years.8 New agents with improved safety profiles have been developed. A better understanding of the differences between surgical patients who are generally in good health and the critically ill patients found in the ICU is currently under active investigation. Appropriate medication selection will require a comprehensive understanding of the pharmacodynamics and pharmacokinetics of the various NMBAs as well as the physiologic status of the patient. Adequate sedation and analgesia are paramount because these patients are unable to communicate their distress. Sedation and analgesia and their monitoring are discussed further.
Mode of Action
In general, NMBAs function by competing with acetylcholine (ACh) at the nicotinic cholinergic receptor (nAChR) at the neuromuscular motor end plate (Figure 1). NMBAs are classified as either depolarizing or nondepolarizing agents. The nondepolarizing agents are further divided into short, intermediate, and long acting, and classified according to their chemical structure as aminosteroidal or benzyl-isoquinoline compounds.9 The ideal muscle relaxant would have rapid onset, with predictable and controllable duration of action, and lack adverse effects on hemodynamics or significant toxicity. Its elimination should be independent of liver and renal function without accumulation over time. The ideal paralytic agent would have no interactions with other medications, as well as being cost effective with a long shelf life.
Depolarizing Neuromuscular Blocking Agents
The only commercially available depolarizing NMBA is succinylcholine. Succinylcholine results in a lasting depolarization of the motor end plate, leading to an initial uncoordinated contraction of the muscle fibers, observed as “fasciculations,” but preventing subsequent activation. Through the continued stimulation of the nAChRs, repolarization of the receptors and subsequent muscle contractions are inhibited.9 The rapid onset and short duration of succinylcholine make it ideal for rapid-sequence intubations (dose 1–1.5 mg/kg IV bolus)9 (Table 2). But the concomitant potassium release as a result of the initial depolarization can be dangerous even in normal patients with borderline elevated potassium levels, but in particular in stroke, paralysis, burn, and spinal trauma patients 24 hours after the initial insult. This is because extrajunctional ACh receptors will have developed at denervated muscle fibers; they respond to succinylcholine by opening their potassium channels. Adverse effects of succinylcholine include the previously mentioned hyperkalemia, cardiac arrhythmias (in particular, bradycardia in children), myalgias, myoglobinemia, increased ICP, intraocular and gastric pressure, allergic reaction, and prolonged paralysis in patients with acquired or genetic plasma-cholinesterase deficiencies. Succinylcholine is a triggering agent for malignant hyperthermia in susceptible patients.
Nondepolarizing Neuromuscular Blocking Agents
Unlike succinylcholine, nondepolarizing NMBAs prevent muscle contraction by competitively inhibiting the binding of ACh to nAChRs. Structurally, the nondepolarizing NMBAs are classified as aminosteroidal compounds (vecurnonium and pancuronium) or as benzyl-isoquinolinium compounds (atracurium and cis-atracurium). Because of similarities in their chemical structure, various commonly used medications can imitate or alter the action of NMBAs or interfere with their elimination (see Table 2). Clinically the nondepolarizing NMBAs are classified by duration of action. ACh receptors are present in both the central nervous system (CNS) and the peripheral nervous system. Because nondepolarizing NMBAs are large charged molecules, they are unable to cross the brain–blood barrier into the CNS or enter across the placenta into the fetal circulation. Hence, ACh binds to receptors in the nerve ganglia and at the neuromuscular junction. NMBAs bind preferentially to the ACh receptor in the neuromuscular junction, but minimal interactions with the ACh receptors found at the autonomic ganglia help explain some side effects like the mild tachycardia observed with vecuronium and pancuronium or the bradycardia associated with the use of succinylcholine.
Pancuronium
Pancuronium (Pavulon®) is the principal long-acting aminosteroidal nondepolarizing NMBA used in the ICU. The chief advantages of pancuronium are its relatively low cost per dose and its comparatively long duration of action allowing for intermittent bolus dosing.8 Pancuronium is partially metabolized in the liver and excreted as the parent compound or as a metabolite by the kidneys. Significant renal or hepatic dysfunction may lead to prolonged paralysis. Other complications of pancuronium result from its vagolytic effect, which can result in tachycardia and hypertension.9
Doxacurium
Doxacurium (Nuromax®) is a relatively new long-acting benzyl-isoquinoline compound that causes little to no histamine release or cardiovascular side effects. In addition, duration of effect does not seem to accumulate with repeated dosing. Elimination appears to be independent of renal function.10
Vecuronium
Vecuronium (Norcuron®) is an intermediate-acting aminosteroid NMBA that is structurally related to pancuronium. The loss of a methyl group considerably decreases its vagolytic effect. Vecuronium is metabolized by the liver with three known metabolites retaining paralytic activity. The parent compound and its metabolites are excreted by the kidneys. Vecuronium can be administered either as intermittent IV boluses or as a continuous infusion.9
Atracurium
Atracurium (Tacrium®) and its isomer cis-atracurium (Nimbex®) are benzyl-isoquinolinium NMBAs. The advantage of these compounds is their elimination via Hoffmann degradation independent of both hepatic and renal function. Essentially, the drug spontaneously disintegrates in the warm physiochemical environment of blood (hence, unlike other NMBAs, atracurium needs to be cooled for storage). A metabolite common to both compounds is laudanosine, which in vitro has been shown to cause seizures. However, there are currently no documented occurrences of a seizure in a human receiving either of these medications. Cisatracurium is the cis-isomer of atracurium9 and as a result lacks the histamine release, the major side effect of atracurium. Both compounds can be administered as intermittent boluses or as a continuous infusion.
Monitoring of Neuromuscular Blockade
The primary use of NMBAs in the ICU is to facilitate mechanical ventilation.4 The degree of neuromuscular blockade required to reach this clinical goal will vary from patient to patient. The first set of guidelines detailing the use of NMBAs in the ICU was published in 1995 and later reevaluated in 2002. The current guidelines state that any patient receiving a NMBA should be assessed both clinically and by train-of-four (TOF) monitoring, with the clinical goal of NMBA titration to one or two twitches.11 Daily clinical assessment involves the observation of skeletal muscle movement and respiratory effort by the patient. Peripheral nerve stimulation by four equal electrical charges delivered at 0.5-second intervals can be evaluated with visual, tactile, or mechanical means (Figure 2). The TOF count is the observed number of twitches out of four. A TOF count of one out of four signifies that 90%–95% of the nAChRs are occupied. A TOF count of three out of four signifies that 75%–80% of the nAChRs are occupied. At 50% occupancy of the nAChRs, the patient can be safely extubated.12 This translates clinically to a sustained head lift for 10 seconds or to a train of four of four plus sustained tetanus to a 5-second stimulation with 100 Hz. Complete resolution of neuromuscular blockade is important not only to ensure adequate respiratory muscle power to sustain the work of breathing without ventilator support, but even more for the patient to protect the airway by unimpaired activity the bulbar muscles. Ulnar nerve innervation of the adductor pollicis muscle of the thumb is the most frequently used site to monitor TOF. The electrical stimulus should flow through the nerve, reach the neuromuscular junction, and release ACh, leading to a muscle contraction. If the electrodes are too close to the muscle tested, the current may directly cause a false-positive contraction. Hence any nerve/muscle pair that is at a certain distance apart can be used, such as the facialis/orbicularis oculi or the peroneus nerve/tibialis anterior. The monitoring of the degree of neuromuscular blockade may allow for the lowest NMBA dose and may minimize the adverse effects of prolonged NMBA use.11
Complications of Prolonged Neuromuscular Blockade
Complications related to the prolonged use of NMBAs include prolonged recovery from NMBAs, critical illness myopathy, and critical illness polyneuropathy.11 Prolonged recovery from NMBAs is defined as the time to recovery requiring 50%–100% more than predicted by pharmacologic parameters, and is most likely due to the accumulation of NMBAs or their metabolites (American Society of Anesthesiologists [ASA] guidelines). As detailed previously, the steroid-based NMBAs undergo hepatic metabolism yielding active metabolites. Interaction of the NMBAs or their metabolites with other concurrent medications may also explain the prolonged blockade (see Table 1).
Critical Illness Myopathy
Critical illness myopathy (CIM), also referred to as acute myopathy of intensive care and acute quadriplegic myopathy, is believed to be an acute primary myopathy. This myopathy results in diffuse weakness or flaccid paralysis with distal and proximal muscle groups equally affected. The muscle weakness persists long after the discontinuation of the NMBA and the elimination of the NMBA and its metabolites. The respiratory muscles are commonly involved, which prevents weaning from the ventilator.13 The majority of patients recover within 4 months; however, permanent neurologic deficits have been reported.14 An association of concurrent administration of NMBAs and corticosteroids with CIM exists.15–18 In addition, the prolonged use of both NMBAs and corticosteroids beyond 1–2 days increases the risk of myopathy (ASA guidelines). The ASA guidelines on the use of NMBAs in the ICU recommend that for patients receiving both NMBAs and corticosteroids, every effort should be made to discontinue the NMBAs as quickly as possible. The use of drug holidays may decrease the incidence of CIM.11
Critical Illness Polyneuropathy
Critical illness polyneuropathy (CIP) occurs due to diffuse axonal polyneuropathy. The mechanism is thought to involve impaired peripheral nerve perfusion, which leads to microvascular ischemia of the nerve.11,19 The electroneurographic pattern demonstrates a decrease in the amplitude of the nerve action potential with preserved normal conduction velocities. This condition is contrasted with Guillain-Barre syndrome (GBS), demyelinating polyradiculoneuritis, in which the amplitude of the nerve action potential is preserved and the conduction velocity is decreased.20 The occurrence of both CIP and CIM in critically ill patients is high; however, the occurrence of CIP has not been associated with the use of NMBAs (Table 3).
Table 3 Risk Factors for Critical Illness Myopathy and Polyneuropathy
Adapted from Latronico N, et al: Critical illness myopathy and neuropathy. Curr Opin Crit Care 11(2):126–132, 2005.
Functional Defects of Neuromuscular Junction
A last potential cause of motor dysfunction in ICU patients could be due to alterations in the neuromuscular junction. Conditions such as spinal cord injuries, cerebral vascular accidents, burns, crush injuries, and immobilization result in increased expression of abnormal nAChRs. These abnormal receptors are more sensitive to depolarizing NMBAs (succinylcholine) and more resistant to nondepolarizing NMBAs.21 The infusion of subparalytic doses of NMBAs has been shown to increase the expression of nAChRs in the absence of immobilization.22
SEDATION AND ANALGESIA IN CRITICAL CARE SETTING
Analgesic Agents and Their Advantages
Morphine and fentanyl are the most widely used and appropriate opioid analgesics for use in the critical care setting (Table 4). Both are cleared by the liver. Although morphine is less costly, it has an active metabolite, morphine-6-glucoronide, that is renally excreted and can hence accumulate leading to renal failure. Fentanyl is eliminated by the liver only, and hence is safe even for long infusions in renal failure. Fentanyl, being very lipophilic, also has a more rapid onset compared to morphine, making it very suitable for the titration of acute pain at the bedside. Caution is warranted, however, as its rapid offset after a single dose is due to redistribution, not metabolism: This means that a few single small dose of fentanyl will only last for about 30 minutes, but with a continuous infusion fentanyl will behave similarly to other long acting narcotics like morphine and may take several hours to wear off. Hydromorphine may be preferable in patients with hemodynamic compromise, because of morphine’s potential for histamine release or preload reduction. Meperidine and the mixed agonist/antagonist opioids should be avoided. The former because of its renally cleared proconvulsant metabolite, normeperidine. The latter because they can precipitate withdrawal in opioid habituated patients.
Indications and Patient-Controlled Analgesia
Epidural and Regional Analgesia
To administer analgesics only where the pain originates makes intuitive sense. Besides, local anesthetics lack the respiratory and sedative side effects of their narcotic counterparts. By blocking the conduction of nerves reporting the nociception to the CNS, superior analgesia can be achieved (Figure 3). Limitations are the need for expertise in performing regional blocks or epidural anesthesia. Currently, duration of action of local anesthetic agents is only a matter of hours, making repeated blocks necessary. While longer-acting agents in depot formulations are on the horizon, they are not yet clinically available. Leaving catheters in place for continuous infusions may work well, but is fraught with infectious risks, particularly in septic patients. In addition, in the current litigious climate many anesthesiologists are wary about performing procedures on sedated or unconscious patients who cannot give feedback during block performance. Many critically ill patients are anticoagulated or coagulopathic. In particular, in the tight epidural space a hematoma can have devastating neurological outcomes. Hence, epidural and regional blocks and catheters can only be placed in time windows when the benefits outweigh the bleeding risk or when the anticoagulation is held for a few hours. Once the catheter is in place, prophylactic anticoagulation may be resumed, and indeed may even become superfluous. This is because epidural anesthesia has intrinsic antithrombotic effects by its action on the autonomic system (and consequently blood flow), and because regional anesthesia may significantly enhance patient mobilization. In particular, for the pain of rib fractures and thoracotomies, the superior epidural analgesia in the absence of respiratory depression will convey a significant survival benefit in the elderly critically ill and in patients with respiratory comorbidities like chronic obstructive lung disease or pulmonary fibrosis.
Sedatives
Benzodiazepines and propofol have anxiolytic, sedative, and anticonvulsive properties, but no analgesic properties. Indeed, good pain control will significantly reduce, if not eliminate, the need for sedation. The sedative agent is chosen based on the clinical need for short-term reversible or prolonged sedation, taking into account comorbidities such as renal failure that might prolong the agent’s duration of action (Table 5).
Haloperidol is the drug of choice for treating ICU psychosis. It is a neuroleptic with strong antidopaminergic activity, and hence patients need to be monitored for extrapyramidal side effects, neuroleptic malignant syndrome, and QT prolongation on the electrocardiogram. Neuroleptic malignant syndrome (NMS) is a rare potentially fatal central neural disorder characterized by hyperthermia, rigidity, autonomic instability, and altered mental status, mediated by central and postganglionic dopamine agonism. NMS is different from the anesthesia-related muscular disorder malignant hyperthermia that is triggered exclusively by succinylcholine and volatile anesthetics such as halothane.
Dexmedetomidine is a new centrally active alpha-2 agonist, available for intravenous administration (Figure 4). Unlike all other sedative agents discussed previously, it has analgesic properties, yet unlike opioids it does not depress the respiratory drive. Unlike most other sedative and analgesic medications, it can be continued during trials of spontaneous ventilation and even during the actual extubation itself. Many self-extubations occur precisely when the analgesic and anxiolytic coverage is reduced to assess the patient’s suitability for extubation. On the other hand, dexmedetomidine is sometimes by itself not strong enough in trauma patients to control pain, or in patients habituated to ethanol, benzodiazepine, or other recreational drugs, to achieve adequate sedation, and hence supplementation may be necessary (Figure 5). Theoretically, dexmedetomidine might be a suitable drug for analgesia and sedation by nonanesthesia personal for minor procedures in patients with an unsecured airway like dressing changes in burn patients. However, dexmedetomidine is not approved by the Food and Drug Administration (FDA) for this indication, nor can this approach be recommended as long as there is no more evidence in the literature supporting its safety profile under these circumstances. Dexmedetomidine is started as an infusion with or without initial bolus. Depending on the dose, dexmedetomidine can cause hypertension as well as hypotension, and may lead to bradycardia. The latter is often a desired effect in patients with a history of coronary artery disease, as this prolongs the time of myocardial perfusion during diastole and reduces myocardial oxygen demand, but it can reduce cardiac output in patients depending on heart rate for cardiac output.
BISPECTRAL INDEX MONITORING
The bispectral index (BIS) is a neurophysiologic monitor that was designed with the intention of measuring unconsciousness under what would otherwise be an uncomfortable perioperative experience. It received FDA clearance in 1996 with studies correlating the BIS index to response to verbal command and to a lesser extent, movement upon surgical incision with various modes of anesthesia. Since then, it has become ubiquitous with prevention of perioperative awareness, and has found a niche among monitoring equipment in ORs across the country. While the popularity of this instrument has become synonymous with outpatient procedures, a new frontier has slowly developed, that is, the use of the BIS monitor in the critical care setting (Figure 6).
Benefits of Bispectral Index in Critical Care Setting
Sleep disruption in critically ill patients is a well-documented phenomenon. It has been shown to increase the state of confusion, more eloquently termed ICU psychosis, in addition to contributing to respiratory dysfunction. A review by Eveloff23 and Gabor et al.24 concluded that sleep disruption in the ICU can cause increased respiratory muscle fatigue and decreased ventilatory responsiveness to hypercapnia, which could prolong mechanical ventilation. Another study by Rundshagen and colleagues25 found that recall of nightmares and hallucinations in sedated and ventilated patients discharged from the ICU approached 9.3% and 6.6%, respectively. Sleep disruption with decreased slow wave patterns has also been suggested to predispose for post-traumatic stress disorder. The common element in these investigations is the value of attaining near normal sleep patterns, while preventing awareness of stressful stimuli during the ICU period, both of which could be assessed by the BIS monitor.
Minimization of drug withdrawal symptoms is another unforeseen benefit of the BIS index in the ICU. In a study by Cammarano and colleagues,26 9 of 28 patients mechanically ventilated for more than 7 days were found to exhibit withdrawal reactions including insomnia and sleep disturbances. This association was attributed to high-dose opioids and benzodiazepines. The implementation of sedation scores theoretically should minimize the risk of excessive doses; however, the major drawback to the traditional scoring systems is their reliance on the subjectivity of the practitioner. Several studies have shown that nurses rely on previous experiences with sedation for their population of patients, which is further complicated by a lack of scientific evidence for a particular sedative practice. In addition, most cues used by critical care nurses to judge sedation are inadequate. The importance of objective data is therefore paramount to the optimal management of sedation and convalescence in the critically ill. With the BIS monitor, an objective method for the titration of sedation transforms the realm of abstract theory to tangible and practical clinical application (Table 6).
Table 6 BIS Values and EEG Patterns Correlated to Hypnotic States at Administration of Sedative Agents
Clinical State | BIS Value | EEG Pattern |
---|---|---|
Awake | 100 | Alpha wave predominance |
Moderate hypnotic state | 60 | Decreased alpha/increased beta wave |
Deep hypnotic state | 40 | Predominance of delta and theta waves |
Cortical silence, coma, brain death | 0 | Isoelectric EEG, burst suppression |
BIS, Bispectral index; EEG, electroencephalograph.
Computing the Bispectral Index
The BIS is derived empirically based on a statistical model; it is not a physiologic parameter. It was attained by analyzing a large database of EEGs from healthy volunteers who had received one or more of the most commonly used hypnotic agents (Figure 7). EEG features were identified that characterized some portion of the EEG spectrum that changed as the subjects went from the awake state to anesthetized (Figure 8). Multivariate statistical models were then used to derive the optimum combination of these features, which were then transformed into a dimensionless 0–100 linear scale. Details of the algorithm and statistical model are proprietary information. However, a brief four-step model of the process is described in the following (Figure 9):
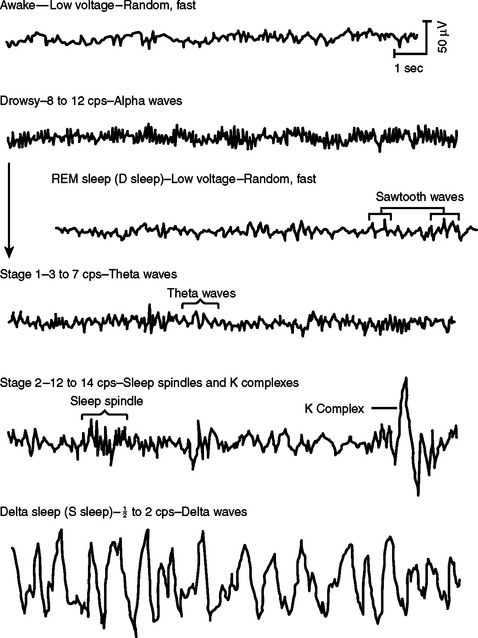
Figure 8 Illustration of electroencephalogram patterns from waking state to sleep sleep.
(Modified from Hauri P: The sleep disorders. Kalamazoo, MI, 1977.)
With a BIS number designation, an assessment can then be made on the hypnotic state of the patient.
Limitations
The BIS monitor, although novel in its approach on quantifying the level of sedation, is not impervious to the setbacks of derivation and statistical models (Table 7). Namely, the instrument’s ability to assess a given level of sedation is only as good as the database with which it compares the filtered EEG. Recalling the aforementioned development process, it is reasonable to presume that the differences between healthy volunteers and critically ill patients undergoing sedation may increase the margin of error, especially when considering that a portion of these patients have neurologic dysfunction or injury. Comparing these EEGs to a database of healthy patients does not seem to be an ideal scenario for assessing the hypnotic state in these patients.
Circumstance(s) | BIS Reading | Description |
---|---|---|
Electromyographic activity | Falsely increased | EMG artifact misinterpreted as cerebral activity. |
Neuromuscular blockade | Falsely decreased | Removal of EMG artifact. |
Electronic devices | Falsely increased | Electromagnetic fields, vibrations, and electric interference interpreted as increased cerebral activity. |
Cerebral injury | Falsely decreased | Decreased cerebral perfusion, neurological damage, and abnormal mental function leads to lower EEG activity. |
Alzheimer’s | Falsely decreased | Decreased beta waves equate to overall decreased collection of EEG data. |
Lack of stimulation | Fails to predict movement upon stimulation | BIS readings are retrospective but not predictive. They only assess adequacy of sedation at current level of stimulation. |
Various combination of sedatives and opioids in critically ill patients | BIS value studied mostly for healthy volunteers, general anesthesia, and pure drug regimes | BIS values change for a given clinical endpoint depending on medication combination used. |
Note: This table organizes the most common reasons for erroneous BIS-Monitor readings. Included are the potential root causes and a brief description of why they occur.
BIS, Bispectral index; EEG, electroencephalograph; EMG, electromyogram.
Prospective Uses
The BIS monitor, an instrument designed for the quantification of sedation, has prospective uses as both an endpoint determinant of pentobarbital therapy and as a diagnostic tool. Traumatic head injury, cerebral hemorrhagic events, and neoplasms are significant causes of increased intracranial pressures. Pentobarbital-induced coma is a second-line treatment for intracranial hypertension refractory to osmotic or diuretic agents and cerebrospinal fluid drainage. Up to one-third of medical centers within the United States use barbiturates as a treatment modality for ICP. Pentobarbital-induced coma can be confirmed by an isoelectric pattern with intermittent “bursts” of activity—burst suppression—on the EEG. This pattern serves as a guide to therapy that minimizes barbiturate dosage and the likelihood of toxicity. The BIS monitor has the capacity to collect raw EEG data and display burst suppression ratios as a percentage of isoelectric episodes over a 63-second period. The size of the monitor and its ability to express burst suppression ratios as a numerical value make it an ideal bedside monitor in the ICU. Riker and colleagues’27 prospective study of 12 patients comparing EEG monitoring values to BIS values found that BIS values correlated well with the standard EEG-based method used to titrate barbiturate therapy. With the BIS monitor, titration of a pentobarbital infusion could occur on a minute-to-minute basis without the use of special technicians, bulky equipment, or interpretation of the EEG by a neurologist hours later. This could significantly reduce overall cost and improve care of patients with increased ICP.
SUMMARY
The care of critically ill patients in the ICU at times may require the use of NMBAs for any number of reasons. As with any medication, the risk:benefit ratio of using NMBAs must be calculated on a patient-by-patient basis. Using the lowest dose possible for the shortest time period required can minimize the complications of using NMBAs. In addition, it must be remembered that NMBAs do not provide analgesia or amnesia, and require the concurrent use of sufficient dosages of pain medication and sedation. EEG monitoring is now commercially available for assessment of adequate sedation, and also for burst suppression in pentobarbital coma, even though its use in the ICU is still being developed. Good analgesia, including regional techniques where indicated, will reduce the need for sedation and improve outcomes most if individualized and include the use of patient-controlled administration of analgesics. Adequate sedation that is adjusted with frequent patient assessment or participation and daily wake-up tests will help prevent excess morbidity from oversedation as well as recall of unpleasant experiences and post–intensive care, traumatic stress disorders.
1 Lawen A. Ueber die Verbindung der Lokalanasthesie mit der Narkose, uber hohe Extraduralanasthesie and epidurale Injektionen Anasthesia render Losungen bei tabischen Magenfrisen. Beitr Klin Chir. 1912;80:168-189.
2 Helliwell TR, et al. Necrotizing myopathies in critically ill patients. J Pathol. 1991;164:307-314.
3 Murray MJ, et al. The use of neuromuscular blocking drugs in the intensive care unit: a U.S. perspective. Intensive Care Med. 1993;19:S40-S44.
4 Klessing HT, et al. A national survey on the practice patterns of anaesthesiologist intensivists in the use of muscle relaxants. Crit Care Med. 1992;20:1341-1345.
5 Murphy GS, Vender JS. Neuromuscular-blocking drugs: use and misuse in the intensive care unit. Crit Care Clin. 2001;17:925-942.
6 Werba A, et al. Vecuronium prevents increases in intracranial pressure during routine tracheobronchial suctioning in neurosurgical patients. Anaesthesist. 1986;40:328-331.
7 Hsiang JK, et al. Early, routine paralysis for intracranial pressure control in severe head injury: is it necessary? Crit Care Med. 1994;22:1471-1476.
8 Ohlinger MJ, Rhoney DH. Neuromuscular blocking agents in the neurosurgical intensive care unit. Surg Neurol. 1998;49:217-221.
9 Stoelting RK. Pharmacology and Physiology in Anesthetic Practice, 3rd ed. Philadelphia: Lippincott-Raven, 1999.
10 Murray MJ, et al. Double-blind, randomized, multicenter study of doxacurium vs. pancuronium in intensive care unit patients who require neuromuscular blocking agents. Crit Care Med. 1995;23:450-458.
11 Murray MJ, et al. Clinical guidelines for sustained neuromuscular blockade in the adult critically ill patient. Crit Care Med. 2002;30:142-156.
12 Frankel H, et al. The impact of implementation of neuromuscular blockade monitoring standards in a surgical intensive care unit. Am Surg. 1996;62:503-506.
13 Douglass DA, et al. Myopathy in severe asthma. Am Rev Respir Dis. 1992;146:517-519.
14 Kordas M. The effect of procaine on neuromuscular transmission. J Physiol. 1970;209:689-696.
15 Road J, et al. Reversible paralysis with status asthmaticus, steroids, and pancuronium: clinical electophysiological correlates. Muscle Nerve. 1997;20:1587-1590.
16 David W, et al. EMG findings in acute myopathy with status asthmaticus, steroids, and patalytics: clinical and electrophysiologic correlation. Electromyogr Clin Neurophysiol. 1998;38:371-376.
17 Zochodne DW, et al. Acute necrotizing myopathy of intensive care: electrophysiologic studies. Muscle Nerve. 1994;17:285-292.
18 Marik PE. Doxacurium-corticosteroid acute myopathy: another piece to the puzzle. Crit Care Med. 1996;24:1266-1267.
19 Bolton CF. Sepsis and the systemic inflammatory response syndrome: neuromuscular manifestations. Crit Care Med. 1996;24:1408-1416.
20 Latronico N, et al. Critical illness myopathy and neuropathy. Curr Opin Crit Care. 2005;11:126-132.
21 Yanaz P, Martyn JAJ. Prolonged D-tubocurarine infusion and/or immobilization cause upregulation of acetylcholine receptors and hyperkalemia to succinylcholine in rats. Anesthesiology. 1996;84:384-391.
22 Hogue CW, et al. Tolerance and upregulation of acetylcholine receptors following chronic infusions of d-tubocurarine. J Appl Physiol. 1992;72:1326-1331.
23 Eveloff SE. The disruptive ICU. An issue to lose sleep over? Chest. 1995;107:809-818.
24 Gabor JY, et al. Sleep disruption in the intensive care unit. Curr Opin Crit Care. 2001;7:21-27.
25 Rundshagen I, et al. Incidence of recall, nightmares, and hallucinations during analgosedation in intensive care. Intensive Care Med. 2002;28:38-43.
26 Cammarano WB, et al. Acute withdrawal syndrome related to the administration of analgesic and sedative medications in adult intensive care unit patients. Crit Care Med. 1998;26:676-684.
27 Riker RR, et al. Comparing the bispectral index and suppression ratio with burst suppression of the electroencephalogram during pentobarbital infusions in adult intensive care patients. Pharmocotherapy. 2003;23:1087-1093.