CHAPTER 18 Anesthesia for General Surgery in the Neonate
Surgical procedures during the neonatal period impose significant physiologic perturbations on the infant. With increasing regularity, diseases of adult life have been linked to events in early development (in utero, newborn, infancy, or childhood). For example, intrauterine growth retardation has been linked to an increased risk for a variety of adult disorders, including coronary heart disease, stroke, hypertension, type II diabetes, and even psychiatric illness (Barker and Osmond, 1986a; Barker, 1995, 2006; Ravelli et al., 1998; Singhal et al., 2003, 2004; Barker et al., 2005; Mittendorfer-Rutz et al., 2007). In addition, early postnatal and childhood nutrition and obesity have been associated with metabolic disorders such as dyslipidemia and glucose intolerance, growth abnormalities, and cardiovascular disease in adults (Huang et al., 2007; Mikkola et al., 2007; Rakow et al., 2008). These adult disorders with pediatric foundations were initially based on Barker and Osmond’s epidemiologic study (1986b). However, subsequent research has expanded his original “thrifty-phenotype” hypothesis (i.e., survival of the undernourished fetus demands that nutrition be directed to vital organs such as the brain, resulting in insulin resistance in other tissues such as muscle and the pancreas) to include a “developmental plasticity” theory (Hales and Barker, 2001; Gluckman et al., 2005; McMillen and Robinson, 2005). These initial reports have spawned an entire focal point for research and a model termed the developmental origins of health and disease (Gluckman et al., 2005; Gillman et al., 2007; Silveira et al., 2007). Recently, the increased risk for cardiovascular and metabolic disease has been extended to include preterm infants, both those who are appropriate for gestational age (AGA) and those who are small for gestational age (SGA) (Hofman et al., 2004a, 2004b; Mikkola et al., 2007).
Anesthetic pharmacology in the neonate
Increasing evidence indicates that the physiologic response of neonates to painful stimuli is similar to that of adults (see Chapter 15, Pain Management). The response of the sympathetic nervous system to noxious stimulation includes tachycardia and hypertension, which in the setting of abnormal cerebral autoregulation predisposes the infant with a low birth weight (LBW) to intraventricular hemorrhage and possibly pulmonary hypertension. The goal during anesthesia is to avoid pain and its cardiovascular and neurologic consequences. The response of newborns to opioids and potent inhalation agents is variable, and meticulous titration is critical for neonates undergoing surgery, both to avoid cardiovascular collapse and to maintain acid-base balance and also to eliminate awareness and pain.
Pharmacokinetics and Pharmacodynamics
Pharmacokinetics and pharmacodynamics of drugs are affected by anatomic factors relating to body composition and distribution of water, as well as physiologic factors such as metabolism (i.e., hepatic biotransformation); protein binding; and pathologic factors (e.g., disease, anesthesia, and surgery) (see Chapter 7, Pharmacology of Pediatric Anesthesia). Maturational changes in distribution of total body water, tissue composition, and organ function contribute to the unique response of the newborn and young infant to various drugs. In early fetal development, water constitutes approximately 94% of body weight. As gestation continues, the total body water decreases so that at 32 weeks, 80% to 90% of body weight is water, and at term, total body water is approximately 70% to 75% of body weight. Adult proportions of fluid to body weight (55%) are reached between the ages of 9 months and 2 years. The distribution of water between the extracellular and intracellular compartments also changes during fetal growth. Extracellular water (interstitial fluid plus plasma volume) decreases from 60% of body weight at the fifth month of fetal life to approximately 45% at term. Intracellular water increases from 25% in the fifth month of fetal life to 33% at birth; therefore, the extracellular fluid compartment of the newborn is equal to or greater than the intracellular fluid space. In adults, the intracellular and extracellular fluid compartments are approximately 40% and 20% of body weight, respectively. Because the plasma component of the extracellular fluid compartment remains at approximately 5% of body weight throughout life, it is the interstitial water that is greater in infancy (40%) and declines to 10% to 15% in the adult (Friis-Hansen, 1971).
Age-dependent changes in body composition also occur. At term, fat constitutes 11% of body weight. Fat content doubles by 6 months of age and is approximately 30% at 1 year. Teenage girls remain with approximately 20% to 30% fat, whereas fat in teenage boys decreases to 10% to 15% fat. Moreover, the composition of fat tissue changes with age. Fat of the newborn may contain as much as 57% water and 35% lipids; adults have 26% water and 71% lipids (Friis-Hansen, 1971). Skeletal muscle comprises 25% of total body mass in a full-term newborn compared with 43% in an adult.
The binding of drugs to serum proteins depends on several factors, including the concentration of protein, the number of binding sites on these proteins, and the affinity of the binding sites. The concentration of total serum protein, albumin, and α1-acid glycoprotein is lower in early infancy and reaches adult levels by approximately 1 year of age (Pacifica et al., 1986). Albumin primarily binds acidic drugs; α1-acid glycoprotein binds basic drugs. The concentration of these two proteins and their binding affinities are deficient in the newborn (Piafsky and Woolner, 1982).
The primary organ for drug biotransformation is the liver, but the kidneys, intestines, lungs, and skin also have minor roles. Hepatic oxidation, reduction, and hydrolysis (nonsynthetic, phase I reactions) mature rapidly, achieving adult rates by 6 months (Niems et al., 1976). Drugs metabolized via this cytochrome P450-dependent monooxygenase system include phenobarbital and phenytoin. Conjugation reactions (synthetic, phase II reactions) convert drugs into more polar compounds to facilitate renal excretion. These systems also mature postnatally.
The renal excretion of drugs is a function of glomerular filtration rate (GFR), active secretion, and passive reabsorption. GFR and secretion increase in an age-dependent manner. Renal blood flow and GFR increase dramatically during the first postnatal week and more gradually during the next several months, and adult performance is achieved at approximately 6 to 12 months of age (Arant, 1978; Hook and Bailie, 1979). Tubular secretory and reabsorptive capacity also mature postnatally (Fetterman et al., 1965).
Inhaled Anesthetic Agents
Infants have a higher incidence of cardiovascular instability and cardiac arrest during induction of inhalational anesthesia than do older persons (Rackow et al., 1961; Friesen and Lichtor, 1982, 1983; Morray, 2000, 2002; Murat et al., 2004). This untoward effect of potent inhalational agents can be attributed to several factors, including faster equilibration, rapid myocardial uptake in infants, the increased anesthetic requirement, and sensitivity of the neonatal myocardium. Infants attain a higher concentration of inhaled anesthetic agents in the heart and brain than do adults at the same inspired concentration. Moreover, the neonatal myocardium has a smaller fraction of contractile mass, and the magnitude and velocity of fiber shortening are less than in the adult myocardium. These factors and the increased anesthetic requirement, which is inversely related to age, all produce a higher incidence of adverse cardiovascular effects in infants.
The rate of rise of the alveolar concentration of an inhaled anesthetic depends on several factors: the inspired concentration, alveolar ventilation, and uptake. The greater the alveolar ventilation, the faster is the rate of rise of the alveolar concentration. This effect of alveolar ventilation is affected by the size of the functional residual capacity (FRC). Infants and children have an FRC similar to that of the adult, 30 mL/kg per minute. In contrast, alveolar ventilation is much higher in the infant (100 to 150 mL/kg per minute) compared with the adult (60 mL/kg per minute). This difference parallels the greater oxygen consumption of the infant. Thus, in the normal term newborn who weighs 3.0 kg, the ratio of alveolar ventilation to FRC is approximately 5:1, compared with the adult, in whom the same ratio is 1.5:1. As a result of this difference, the time constant of the inhaled anesthetic equilibrium for infants is much shorter than for the adult. Consequently, changes in concentrations of inspired gas are reflected rapidly in alveolar levels. In fact, it has been demonstrated that alveolar levels of inhalational anesthetic agents reach equilibrium faster in infants that in adults (see Chapter 7, Pharmacology of Pediatric Anesthesia).
Blood-gas partition coefficients of isoflurane, halothane, and sevoflurane did not differ in preterm infants compared with full-term infants but were lower than in adults. Only serum cholesterol correlated with the blood-gas partition coefficients (Malviya and Lerman, 1990). The blood-gas partition coefficient is an important determinant of solubility and therefore the rate of rise of the alveolar concentration of an inhaled agent.
The effect of age on the solubility of the inhaled agents in tissue is also important in determining the rate of rise of the alveolar concentration of the agent—the rate of anesthetic induction. Data by Lerman et al. (1986) are consistent with earlier work documenting that anesthetic solubility in brain, heart, liver, and muscle increases with age. An increase in solubility may prolong uptake, delay equilibration of the tissue partial pressure of anesthetic, and prolong the time of induction. Lerman and others found that the rate of increase in tissue anesthetic partial pressure, and therefore alveolar anesthetic partial pressure, is approximately 30% more rapid in newborns than in adults.
Minimal alveolar concentration (MAC) is an estimate of anesthetic requirement and changes with age (Fig. 18-1) (Katoh and Ikeda, 1987, 1992; LeDez and Lerman, 1987). In the original study, Gregory et al. (1969) reported that infants in the first 6 months of life had the highest MAC. In a later study, newborns were noted to require approximately 25% less halothane at MAC compared with infants who are between 1 and 6 months of age (Lerman et al., 1983) (see Chapter 7, Pharmacology of Pediatric Anesthesia).
Intravenous Anesthetics and Analgesics
Several studies have shown an increased sensitivity to and more prolonged effects of barbiturates and morphine in the neonate and young infant (Kupferberg and Way, 1963; Way et al., 1965). These features have been attributed in part to the immaturity of the blood-brain barrier, allowing faster and greater penetration and therefore higher concentration of these drugs in the brain.
In 1981, Robinson and Gregory reported that after a 10 mL/kg bolus of lactated Ringer’s solution, 30 to 50 mcg/kg of fentanyl was a safe anesthetic for premature infants undergoing ligation of a patent ductus arteriosus (PDA). Several years later, evidence was presented that infants who received fentanyl in combination with d-tubocurarine and nitrous oxide in oxygen had an improved perioperative course compared with those infants who did not receive analgesics (Anand et al., 1987).
Plasma levels of fentanyl are lower in infants vs. children vs. adults (newborns were not studied) after similar intravenous doses (Singleton et al., 1987). Gauntlett and others (1988) noted that clearance of fentanyl in newborns increased during the first few weeks of life. Elimination half-life and volume of distribution did not change. In a study of newborns who were administered continuous fentanyl infusions, Saarenmaa and others (2000) noted that plasma clearance correlated with maturity (gestational age) and weight, whereas Santeiro and others (1997) noted a correlation of clearance with postnatal age (Fig. 18-2). Koehntop et al. (1986) have shown a highly variable disposition and elimination of fentanyl in neonates. In addition, infants with increased intraabdominal pressure (e.g., omphalocele, gastroschisis, or septic ileus) appeared to have a further increase in the elimination half-life compared with infants undergoing repair of a PDA or myelomeningocele. Davis and others (1989) noted that the clearance of alfentanil in newborn premature infants was markedly reduced compared with older children (Fig. 18-3).
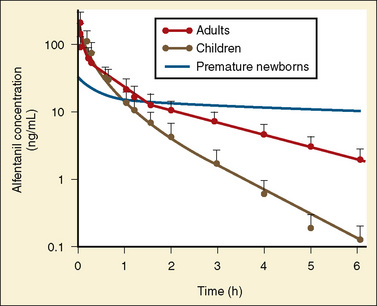
FIGURE 18-3 Age-related changes in alfentanil pharmacokinetics for premature infants, children, and adults.
(From Davis CM, Brando M: Pediatric pharmacology. In Greeley W, editor: Atlas of anesthesiology, vol 7, Philadelphia, 1999, Churchill Livingstone.)
Koren and others (1985) have shown that the elimination half-life for morphine (13.9 hours) in human neonates is markedly prolonged compared with that in older children and adults (2 hours). They also showed reduced clearance and higher serum concentration in neonates compared with those seen in older children after morphine infusion. Because of the large variability in clearance among neonates of different ages, the dose of opioids needs to be carefully titrated for each patient and each clinical setting.
Remifentanil is an ultrashort-acting opioid. Remifentanil is metabolized by plasma and tissue esterases, and its metabolism is independent of organ elimination. As a result, in neonates its pharmacokinetic profile is different from any other opioid. The remifentanil clearance rate in neonates is greater than in any other age group. Although its volume of distribution is large, its terminal elimination half-life does not change with age (Ross et al., 2001). Because remifentanil is metabolized in tissues and plasma, it does not accumulate, and consequently its context-sensitive half-time is flat (i.e., the time for 50% reduction at the effect site is independent of drug duration). Thus, remifentanil may be an ideal drug for anesthetic use in neonates.
Animal studies have shown a decreased median effective dose (ED50) for thiopental in the early weeks of life. Also, arousal in newborn rats occurred at lower brain levels of thiopental than in adult rats (Mirkin, 1975). Although this may suggest that lower doses of thiopental are needed in young neonates, studies by Jonmarker and others (1987) suggest the contrary. Similarly, ketamine requirements are greater (mg per kg of body weight) in infants than in older children (Lockhart and Nelson, 1974). Ketamine has been shown to produce apnea in infants with increased intracranial pressure (Lockhart and Jenkins, 1972). Ketamine produces hypertension and tachycardia, which some anesthesiologists have taken advantage of in caring for infants and children with congenital heart disease, cardiovascular instability, or both.
Propofol is commonly administered to infants. Propofol clearance is dependent on hepatic blood flow (high extraction coefficient) with subsequent metabolism and glucuronidation. In population pharmacokinetic analysis, Allegaert et al. (2007) noted that postmenstrual age and postnatal age were predictive covariants for clearance and that developmental maturation occurs within the first two weeks of life (Fig. 18-4).
Midazolam is an extensively used drug in both full-term and preterm infants and is metabolized by the P450 3A subfamily. Midazolam clearance in preterm infants is less than in children and older infants, and it may reflect the pattern of CYP3A4 ontogeny (Fig. 18-5). DeWildt et al. (2001), in a study of 24 preterm infants, found no relationship between age (postconceptual, gestational, or postnatal) and midazolam clearance. Of note was that in infants exposed postnatally to indomethacin, plasma clearance was higher and the volume of distribution was larger than in those infants not exposed to indomethacin. Because CYP3A4 expression is actuated during the first week after birth regardless of gestational age at birth, the decrease in midazolam clearance probably represents a decrease in CYP3A activity.
Muscle Relaxants
Developmental pharmacologic changes influence the requirements for muscle relaxants in infants and older children. Synaptic transmission is slow at birth, the rate at which acetylcholine is released during repetitive stimulation is limited, and neuromuscular reserve is reduced (Fig. 18-6). In addition, the reported sensitivity of infants to the effects of neuromuscular blocking agents has differed depending on whether drug administration was indexed to body weight or to body surface area. Because most neuromuscular blocking agents are distributed in the extracellular space and the extracellular space is related to the body surface area, dosage requirements for neuromuscular blocking agents often correlate with surface area rather than with body weight. Neonates and infants have a higher sensitivity (lower ED50 and ED95) for most nondepolarizing blocking agents.
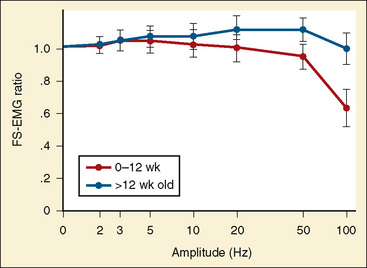
FIGURE 18-6 Tracings of the frequency sweep electromyogram (FS-EMG).
(From Crumrine RS, Yodlowski EH: Assessment of neuromuscular function in infants, Anesthesiology 54:29, 1981.)
Fisher et al. (1982) studied infants’ sensitivity to nondepolarizing muscle relaxants using the pharmacodynamic and pharmacokinetic properties of d-tubocurarine. These investigators determined the steady-state plasma concentration associated with 50% neuromuscular blockade (CPss50) and noted that infants had a lower CPss50 than older children. Because the volume of distribution of d-tubocurarine in infants is significantly larger than that in older children, the dose (mg per kg of body weight) required to achieve the same degree of neuromuscular blockade appeared the same for infants and older children. Although the pharmacokinetic data reveal similar clearance values for infants and older children, the infant’s larger volume of distribution and consequently longer elimination half-life suggest that infants need less frequent and smaller supplemental doses for continued neuromuscular relaxation. Although these data are specific for d-tubocurarine, the general principles can be extrapolated to other hydrophilic compounds that are primarily distributed to the “central compartment” (i.e., small volume of distribution).
Studies of rocuronium in infants and children have shown that onset time in small infants is faster, and the occurrence of 100% block at lower doses compared with older children suggests a greater potency in infants. In addition, rocuronium has an age-dependent difference in duration of action and in recovery following 0.45 mg/kg and 0.6 mg/kg doses (Fig. 18-7) (Driessen et al., 2000; Rapp et al., 2004).
General approach to intraoperative monitoring of the neonate
Thermal Protection
As already described, the newborn infant loses thermal protection after birth, so measures must be taken to protect against heat loss during surgery (Box 18-1):
Monitoring in the Operating Room
Circulatory Monitoring
A precordial stethoscope is a simple and effective means to assess the quality of heart sounds, rate, and rhythm, as well as breath sounds. A change in the intensity of heart sounds indicates a decrease in blood pressure and possibly cardiac output. Depending on the surgical procedure and the method of airway management (mask vs. laryngeal mask airway [LMA] vs. endotracheal tube), an esophageal stethoscope is an alternative monitor for noninvasive beat-to-beat cardiovascular monitoring. Although the sensitivity of the stethoscope has been discussed, the simplicity and accuracy of the precordial and esophageal devices to monitor heart tones and breath sounds during surgery involving pediatric patients cannot be denied (Hubmayr, 2004).
In most cases, the blood pressure can be accurately monitored with an automated device based on oscillometry (e.g., Dinamap) or ultrasonic flow (e.g., Arteriosonde) if the appropriate-sized cuff is available. Cuff inflation of the automatic devices should cycle no more frequently than every 3 to 4 minutes to avoid ischemia to the arm (Waugh and Johnson, 1984). Systolic blood pressure measurements correlate with the circulating blood volume and therefore are essential to monitor and guide fluid and blood replacement. Another alternative system is a Doppler ultrasonic transducer, which has a characteristic sound that decreases in intensity with a decrease in blood pressure.
An indwelling arterial cannula allows repeated blood sampling for cardiopulmonary and biochemical evaluation. A 22- or 24-gauge cannula can be inserted percutaneously or by cutdown into a variety of sites, including the radial, dorsalis pedis, or posterior tibial arteries. Before the insertion of the catheter, the adequacy of circulation to the hand should be assessed by applying a modified Allen’s test (Brodsky, 1975). The axillary artery is rarely cannulated. In general, the umbilical artery can be cannulated in the first 4 to 7 days of life, but this is usually avoided after the first 2 days of life because of the risks of infection and vascular emboli. The umbilical catheter tip can be placed high (T10 to T12) or low, above the bifurcation of the aorta and below the level of the renal arteries (L4, L5). The placement of the catheter must be confirmed by a radiograph (Fig. 18-8).
The risk for retinopathy of prematurity necessitates meticulous monitoring of oxygen saturation in the neonate, especially the very low–birth-weight (VLBW) infant. Most neonatologists recommend adjusting the inspired oxygen to maintain oxygen saturation between 90% and 95%, depending on the underlying medical status, gestational age, hemoglobin, and postnatal age (i.e., quantity of HgF). Of importance, if blood is shunting right-to-left through a PDA, the oxygen saturation measured in the lower extremities or umbilical artery (postductal site) does not reflect the oxygen saturation in the retinal vessels (preductal site). To enable simultaneous monitoring of both preductal and postductal oxygen saturation, two pulse oximeters are placed—one on the right hand (preductal) and one on a lower extremity (postductal). During right-to-left shunting through the PDA, the preductal oxygen saturation is higher than the postductal value, and the difference depends on the amount of shunting. If blood is shunting right-to-left only via the foramen ovale or other intracardiac sites (e.g., ventriculoseptal defect), the preductal and postductal oxygen saturation are equal (see Chapters 34, and 23, Respiratory Physiology in Infants and Children, Cardiovascular Physiology, and Anesthesia for General Abdominal, Thoracic, Urologic, and Bariatric Surgery).
A central venous catheter may be indicated to administer blood, fluid, total parenteral nutrition (TPN) and medications, and to monitor central venous pressure (CVP). Using the Seldinger technique, a catheter can be inserted percutaneously into the subclavian, internal jugular, external jugular, or femoral veins. In emergencies or situations of difficult venous access, the umbilical catheter can be inserted and passed into the right atrium (Fig. 18-8). Its location must be verified by x-ray or by a CVP tracing. Umbilical vein catheters have been associated with portal vein thrombosis. All central venous catheters are associated with significant morbidity, including thrombosis, emboli, and infection. Central venous catheters in the LBW infant have additional risks, from malpositioning and from the disruption of venous flow (ratio of the size of vessel to the size of the catheter is low).
Ventilatory Monitoring
Manual ventilation has been proposed as a technique to allow the anesthesiologist to continuously sense changes in compliance of the chest and airways. However, Spears and others (1991) noted that manual ventilation can be extremely unreliable for sensing changes in airway compliance. In addition to monitoring heart sounds, the precordial or esophageal stethoscope is a simple system to monitor ventilation and quality of breath sounds. Peak airway and end-expiratory pressures should also be measured. End-tidal carbon dioxide devices (mass spectrometers or infrared analyzers) are now the “standard of care” to continuously monitor the adequacy of respiratory exchange. These devices provide a breath-to-breath level of carbon dioxide tension, and the waveform of this measurement can provide information about rebreathing, ventilator disconnection, suspected air embolism, and hypermetabolic states (see Chapter 10, Equipment).
The pulse oximeter provides a precise, continuous readout of the hemoglobin oxygen saturation. During the first 1 to 2 weeks of life and without transfusion of autologous blood, the oxygen dissociation curve of HgF is shifted to the left of the adult curve so that hemoglobin saturation of 95% to 97% corresponds to an arterial oxygen tension (Pao2) of 52 to 77 mm Hg, assuming a Pao2 at 50% hemoglobin saturation (P50) of 19 mm Hg. The hemoglobin saturation should be correlated with an arterial partial pressure of oxygen (Po2) measurement to ensure valid interpretation of oxygen saturation data in the OR (see Chapter 3, Respiratory Physiology in Infants and Children).
Minimal-access surgery in the neonate
The development and refinement of endoscopic tools, optical technology, and surgical skills have allowed even the most complex neonatal procedures, such as congenital diaphragmatic hernia (CDH), tracheoesophageal fistula (TEF), congenital cystic adenomatoid malformation, and lobar emphysema, to be routinely accomplished with minimal-access surgery (Georgeson, 2003; Al-Qahtani and Almaramhi, 2006; Ponsky and Rothenberg, 2008). The field of minimal-access surgery continues to evolve and expand into other arenas such as robotic techniques, “stealth surgery” (or “scarless surgery”), and natural orifice transluminal endoscopic surgery (NOTES) (Dutta and Albanese 2008; Dutta et al., 2008; Isaza et al., 2008).
Endoscopic surgical techniques improve surgical conditions and produce better outcomes for patients. Improved technology has created endoscopic tools and video equipment that produce a magnified image and consequently better visualization of the surgical field. In patients with TEF the thoracoscopic approach improves the surgeon’s view, because the fistula is seen perpendicular to its connection to the membranous trachea; consequently the surgeon may more easily identify the exact site for ligation (Fig. 18-9) (Holcomb et al., 2005; Rothenberg, 2005b).
In addition to better visualization, minimally invasive surgery has also been associated with shorter postoperative recovery periods and decreased pain, inflammation, and scarring (Fujimoto et al., 1999). With less trauma to tissues, fluid therapy may be reduced as well. In some specific procedures, such as repair of a TEF, the avoidance of a posterior-lateral thoracotomy may prevent thoracic nerve damage, winged scapula, shoulder girdle weakness, fused ribs, chest wall asymmetry and muscular/soft tissue developmental abnormalities, and scoliosis (Jaureguizar et al., 1985; Rothenberg, 2005a;). In abdominal surgery, the use of minimally invasive techniques may decrease the incidence of adhesive injury after laparoscopy. This may be especially relevant in the newborn, in whom adhesive bowel obstruction after laparotomy ranges between 1% to 2% per year (Ponsky and Rothenberg, 2008).
Minimal-access surgery in neonates also presents challenges and limitations. The small working space and small size of the newborn, coupled with the unique aspects of neonatal physiology, make thoracoscopic surgery and one-lung ventilation difficult. There is a learning curve to minimally invasive surgery. Procedure times, operative morbidity, and conversion rates to open procedures vary among centers and surgeons (Ponsky and Rothenberg, 2008).
Efficient and safe endoscopic surgery requires both surgical and biotechnical expertise, as well as highly skilled OR and anesthesia teams (Kalfa et al., 2007). Because of these technical challenges, the OR times in the early learning phase may be markedly prolonged compared with operating times for the open procedures (Rothenberg, 2005a; Tsao and Lee, 2005; Nguyen et al., 2006; Kalfa et al., 2007).
Laparoscopy Background
These hemodynamic changes from insufflation, positioning, and hypercarbia are of minimal significance in a euvolemic, healthy adult, but the risks may become significant in patients with underlying cardiac disease (Grabowski and Talamini, 2009). Data documenting cardiorespiratory responses to laparoscopy in infants have been reported, but only about studies involving the use of noninvasive tools, and they differ from some of the results reported in healthy adults.
Using a transesophageal ultrasonic probe inserted after induction of general anesthesia in 12 healthy boys (23 ± 5 months of age), Gueugniaud et al. (1998) noted that pneumoperitoneum (10 mm Hg) decreased aortic blood flow and stroke volume and increased systemic vascular resistance. No effects on mean arterial pressure or end-tidal CO2 were noted. Of importance, these patients remained supine (no reverse Trendelenberg positioning).
Manner et al. (1998) reported the pulmonary effects of pneumoperitoneum in 10 patients between the ages of 1 and 15 years who were undergoing a variety of laparoscopic procedures (intraperitoneal pressure, 12 mm Hg). In this study, the patients’ pulmonary compliance decreased (17% with the reverse Trendelenberg position, 28% with the reverse Trendelenberg position plus insufflation); peak inspiratory pressure increased (25% with the reverse Trendelenberg position, 30% with the reverse Trendelenberg position plus insufflation) and end-tidal CO2 tension (Petco2) concentration increased from 33 to 42 mm Hg at maximum insufflation pressure. In 17 infants (younger than 10 months) who were undergoing a variety of procedures that required at least 30 minutes of laparoscopy but experienced no change in position, Bannister et al. (2003) reported similar findings. Patients were divided into two groups: those weighing less than 5 kg (intraabdominal pressure less than 12 mm Hg), and those weighing more than 5 kg (intraabdominal pressure, less than 15 mm Hg). Again, peak inspiratory pressure increased (18%) and correlated with insufflation pressure, and dynamic compliance decreased (48%). Tidal volume decreased 33% and oxygen saturation fell 2% to 11% in 41% of the patients. Ventilation was adjusted to maintain Petco2 within 10% of baseline, and 20 adjustments were reported. Neither heart rate nor blood pressure changed with insufflation or ventilatory adjustments, and significant changes from baseline status were noted at insufflation pressures of 10 mm Hg and greater but not at pressures of 5 mm Hg. Similar minimal hemodynamic effects of low-pressure pneumoperitoneum have been documented in young children elsewhere (DeWaal and Kalkman, 2003).
Of note, inducing a pneumoperitoneum in the Tredelenburg position during gynecologic surgery in adults decreases the distance between the endotracheal tip and the carina as well as the tracheal length (Kim et al., 2007). Because the distance between the larynx and the carina is markedly less in the infant, this phenomenon may be of critical importance in the newborn undergoing laparoscopy.
Renal function is affected by pneumoperitoneum. However, data have primarily been acquired in the adult population. In adults, decreased urine production has been attributed to the decreased glomerular filtration rate and renal perfusion from the direct effects of pneumoperitoneum (e.g., compression of the renal artery, the inferior vena cava, or the parenchyma) or from secondary effects (e.g., decreased cardiac output and release of mediators) (Rosin et al., 2002). Renal blood flow and renal function decrease during pneumoperitoneum; the effects on blood flow are pressure dependent and exacerbated in the head-up position, but they return to normal after deflation (Demyttenaere et al., 2007).
The effect of pneumoperitoneum on intracranial pressure has been studied in adults. Although the newborn’s cerebrovascular physiology and autoregulatory responses differ from those of the adult, newborns with limited autoregulatory responses may have more profound cerebral vascular responses from increased intraabdominal pressure. In adult animals, intraabdominal pressures of 15 and 25 mm Hg—but not 5 mm Hg—affected intracranial pressure, similar to findings by Rosenthal et al. (1998) (Rosin et al., 2002). Bloomfield et al. (1997) created models that linked intraabdominal, intrathoracic, and intracranial pressures. As with other studies, pleural, peak inspiratory, right atrial, and pulmonary arterial pressures increased as intraabdominal pressure increased above 10 mm Hg. The increases became especially significant at intraabdominal pressures of 25 mm Hg. At the same time cardiac index decreased, but systemic vascular resistance increased and blood pressure remained stable. Increased intracranial pressure accompanied the rise in intraabdominal pressure; however, these changes reversed when the abdomen was decompressed. Of significance, the increase in pleural and intracranial pressures with increased intraabdominal pressure was prevented in a group of swine with the chest opened (e.g., median sternotomy and pleuropericardotomy). However, in spite of the open chest, cardiac index decreased and systemic vascular resistance increased. The investigators suggested that the acuity of the increase in intrathoracic pressure secondary to an abrupt rise in intraabdominal pressure acutely increased central venous pressure, preventing cerebral venous outflow. When they prevented the effect of intraabdominal pressure on the pleural pressure (open chest), venous and intracranial pressures did not change.
The absence of predictable autoregulation of cerebral perfusion in the newborn combined with the significant risk of injury secondary to oxygen and positive pressure ventilation may have unique implications for the anesthetic management of the newborn during either laparoscopy or thoracoscopy. Although indirect monitoring of cerebral blood flow is available (e.g., near-infrared spectroscopy, or NIRS), ideally a reliable, continuous method to accurately monitor cerebral perfusion pressure would be of critical benefit in this setting (Brady et al., 2007).
Laparoscopy and Thoracoscopy in the Newborn
The effects of insufflation during both laparoscopy and thoracoscopy are dramatic in the newborn. The impact of increased intrathoracic pressure and hypercarbia can profoundly influence the transitional circulation. Changes in patient oxygen saturation, hypotension, interruption of the procedure, and hypercarbia are common in the newborn (Kalfa et al., 2005, 2007; Krosnar and Baxter, 2005).
Insufflation in the newborn also decreases core body temperature. In 50% of newborns, the postoperative core temperature was less than 36° C. A long operative time (insufflation for more than 100 minutes) was associated with lower postoperative core body temperature (Kalfa et al., 2005).
Inaccurate end-tidal CO2 values are common during laparoscopy, especially in the setting of cyanotic heart disease (Laffon et al., 1998; Wulkan and Vasudevan, 2001). At the same time, absorption of CO2 from the peritoneum seems to be age-dependent (McHoney et al., 2003). The total amount of insufflated CO2 and elimination of CO2 correlated with age. Younger patients also demonstrated a short period of increased CO2 elimination after desufflation, which may be related to increased venous return after the decrease in intraabdominal pressure.
Because one-lung ventilation is challenging to achieve and at times is not tolerated by a newborn, many pediatric surgeons suggest that insufflation of the chest with low flow of CO2 (1 to 2 L/min, peak pressure of 4 to 6 mm Hg) in the 30- to 45-degree prone position induces adequate lung collapse (Rothenberg, 2002; 2005b; Holcomb et al., 2005).
In spite of the myriad of critical physiologic challenges, endoscopic procedures have been conducted successfully in high-risk infants with conditions such as hypoplastic left heart syndrome, complex cyanotic heart disease, and patients with single ventricle physiology (Mariano et al., 2005; Rice-Townsend et al., 2007; Slater et al., 2007).
Specific neonatal surgical lesions
Abdominal Wall Defects: Gastroschisis and Omphalocele
Gastroschisis and omphaloceles are the most common abdominal wall defects (ectopia cordis and cloacal and bladder extrophy are less common), but they are still rare, with an incidence of approximately 1:10,000 and 1:4000 to 7000 births (Paidas et al., 1994). Some have suggested that both ethnic and geographic origins affect the incidence, but overall the lesions seem sporadic (Mann et al., 2008).
Currently, fetal ultrasounds are routinely conducted to screen for high-risk conditions. For example, nuchal translucency and biochemical markers (free β-subunit of human chorionic gonadotropin and pregnancy-associated plasma protein A) are now measured to screen for aneuploidy or cardiac and other anomalies (Souter and Nyberg, 2001; Weiner et al., 2007). An abnormal value on screening prompts an evaluation that includes a detailed fetal ultrasound; in the case of abdominal wall defects, ultrasound confirms the presence of a lesion in approximately 95% of cases after as few as 10 to 14 weeks’ gestation (Mann et al., 2008). The in utero diagnosis allows planned delivery at a medical center with resources for high-risk obstetric, surgical and anesthetic, and neonatal care.
Although omphalocele and gastroschisis appear to be similar in gross physical appearance, these lesions are distinct from each other. An omphalocele is a central defect of the umbilical ring; the abdominal contents herniate into the intact umbilical sac unless the sac ruptures in utero (Fig. 18-10). The umbilical cord is also inserted into the sac, which contains an internal peritoneal membrane and external amniotic membrane. The lesion has a fascial defect of more than 4 cm (fewer than 4 cm is often considered an umbilical hernia) and often as large as 10 to 12 cm. The sac often contains the stomach, loops of the small and large intestines, and in about 30% to 50% of cases, the liver. A gastroschisis, on the other hand, is an abdominal wall defect that usually occurs to the right of the umbilical cord, with evisceration of bowel through the defect (Fig. 18-11). This lesion is usually 2 to 5 cm in diameter, and in most cases there are only small and large bowel present. In rare cases the liver may exit through the abdominal wall defect as well. The bowel is exposed to the intrauterine environment with no sac, causing its loops to become matted, thickened, and often covered with an inflammatory coating or peel. Whether this exudative peel is secondary to a specific inflammatory pathway or just an effect of amniotic fluid is unclear. The umbilical cord is normal and separate from the defect. When the testes exit along with the bowel, cryptorchidism occurs with gastroschisis (Lawson and de La Hunt, 2001; Weber et al., 2002).
Embryology
Although experimental models have been described, the embryologic etiology of these lesions is not completely understood (Correia-Pinto et al., 2001). Omphalocele has been described as a failure of the cephalic, lateral, and caudal folds to fuse (closure of the exocelomic space) and as abnormal fusion and differentiation of myotomes to form abdominal wall musculature (7 to 12 weeks of gestation). That is, the abdominal cavity is primarily underdeveloped. During weeks 7 to 12 of development, the midgut elongates and herniates into the umbilical cord. By week 12, the abdominal cavity is large enough for the developing gut to exit the cord and reenter the abdomen. Some believe that the simple failure of this return to the abdomen is a developmental arrest that results in an omphalocele and a small abdominal cavity. The abdominal cavity is small only because the gut remains in the umbilical sac.
Gastroschisis is considered to be an earlier embryologic event, resulting from an abnormality of the development of the right omphalomesenteric artery or right umbilical vein, which in turn results in ischemia to the right paraumbilical area. More recently, abdominal wall defects have been ascribed to an abnormal relationship between cell proliferation and planned cell death (apoptosis) at the critical embryonic folding period (Robinson and Abuhamad, 2000). Inadequate mesoderm development may contribute to dysplastic abdominal wall growth. This underdeveloped site is commonly found just to the right of the umbilicus and ruptures with increased pressure of the growing intraabdominal organs. Similar defects in other areas may produce other lesions, such as extrophy of the bladder.
Gastroschisis is usually an isolated lesion, but recent reports raise the possibility of familial occurrence (Yang et al., 1992; Torfs et al., 1994). In utero exposure to acetaminophen, aspirin, and pseudoephedrine has been associated with an increased incidence of gastroschisis (Werler et al., 2002; Baerg et al., 2003). In the case of either an omphalocele or gastroschisis, rotation of the gut is incomplete in utero, resulting in various “malrotation” phenotypes. Intestinal atresias are common, especially in patients with gastroschisis.
Although neither gastroschisis nor omphalocele is considered to be familial, 50% to 75% of infants with an omphalocele have other anomalies, and 20% to 30% have chromosomal abnormalities (Robinson and Abuhamad, 2000; Weber et al., 2002; Mann et al., 2008). Only 15% to 20% of infants with gastroschisis have associated malformations, and only rarely is there a complex pattern of malformation (Stoll et al., 2008). For example, Beckwith-Wiedemann, Rieger’s, and prune belly (Eagle-Barrett) syndromes are associated with omphaloceles, as are trisomies 13, 15, 18, and 21. If the omphalocele contains only bowel and if oligo- or polyhydramnios are present, the likelihood of an associated chromosomal abnormality increases. Others have noted that omphaloceles that contain liver and other viscera are more likely to have cardiac, renal, or limb anomalies, whereas the smaller omphaloceles are more likely to have gastrointestinal or central nervous system malformations (Mann et al., 2008). Syndromes of midline defects often include an omphalocele, and the lesion has been noted in the nonsyndromic multiple congenital anomalies (MCA) complex.
Single-gene mutations have been identified in omphaloceles with multiple anomalies, such as the filamin A, alpha (FLNA) gene in the otopalatodigital syndrome, which is characterized by abnormalities in the axial and appendicular skeleton plus extraskeletal anomalies such as omphalocele (Robertson, 2007). One syndrome, Pentalogy of Cantrell, includes an omphalocele, diaphragmatic hernia, sternal abnormalities, an ectopic and anomalous heart, and gene abnormalities at Xq25 to Xq26.1. Another syndrome (OEIS Complex) involving the lower abdomen includes omphalocele, bladder or cloacal extrophy, imperforate anus, colonic atresia, sacrovertebral anomalies, and meningomyelocele. No environmental or teratogenic associations have been proposed as etiologic causes. Allen et al. (2006) reported an association with assisted reproductive technology. Thus, identifying an abdominal wall defect in utero demands an intense evaluation for associated anomalies, which may be isolated, part of a recognizable malformation syndrome, or chromosomal. In addition, serial ultrasounds should be conducted to evaluate fetal growth and well being, as well as amniotic fluid volume. Of note, after 20 to 32 weeks’ gestation, a higher incidence of fetal demise has been reported. The mode of delivery for infants with abdominal wall defects remains controversial, but recent studies have shown that in gastroschisis and nongiant omphaloceles (i.e., liver extracorporeal), outcome after vaginal delivery is no different than with caesarean section (Henrich et al., 2008). The controversy centers on delivery with gastroschisis because of the unprotected viscera, but no advantages of cesarean section are consistently apparent when survival and incidence of complications are evaluated (Weber et al., 2002).
Preoperative Management
Normothermia should be maintained or achieved by preventing heat loss from the exposed viscera. A bowel bag may be used for this purpose (Towne et al., 1980). Emphasis should be placed on covering the lesion with gauze, nonadherent dressing, and a plastic cover rather than moist (saline-soaked) material, as moisture tends to exacerbate heat loss (Mann et al., 2008). It is critical to avoid distorting the sac or twisting at the base. Decompressing the stomach with an orogastric or nasogastric tube minimizes regurgitation, aspiration pneumonia, and further bowel distention. Broad-spectrum antibiotics are started and intravenous fluid therapy (e.g., lactated Ringer’s solution) at as much as 3 to 4 times (150 to 300 mL/kg per day) the usual maintenance rate (80 to 100 mL/kg per day) is infused to provide adequate hydration and to compensate for a combination of peritonitis, edema, ischemia, protein loss, and significant third-space loss. Without such vigorous fluid resuscitation, hypovolemic shock, hemoconcentration, and metabolic acidosis may develop. A urine output of 1 to 2 mL/kg per hour suggests adequate hydration. Because of the large fluid requirements, acid-base status and electrolyte levels should be monitored carefully by serial arterial or venous blood-gas measurements. Rarely, severe metabolic acidosis develops in spite of aggressive fluid delivery (e.g., coexisting sepsis), and sodium bicarbonate (infusion or bolus) or sodium acetate infusion may be required to maintain the pH at greater than 7.20. In general, delivery of crystalloid and colloid provides adequate support.
Intraoperative Management
Surgical management aims to repair the abdominal wall defect and reduce the protruded viscera. If primary closure is not possible, a staged repair is planned, including the use of the silo chimney or the silastic silo prosthesis (Schuster, 1967). The “silo” consists of a silastic or Teflon mesh that is sutured to the fascia of the defect. The synthetic material used to cover the lesion and the specific mechanism for placing the organs into the abdomen (e.g., umbilical tapes or umbilical cord clamps) vary from center to center. After the silo is in place, the extraabdominal organs are gradually returned to the peritoneal cavity over 3 to 10 days (Fig. 18-12). Improved outcome using the delayed-repair approach after the nonoperative placement of a spring-loaded silo has been described by Schlatter et al. (2003). These authors state that this procedure is accomplished in the neonatal intensive care unit (NICU) or delivery room and requires no anesthesia. The prosthesis is then removed, the lesion is reduced under general anesthesia, and eventually the defect is closed. The authors also claim that the time to both first and full feeds was shorter in infants who underwent delayed closure. Similarly, Pastor et al. (2008) performed a prospective multicenter study of infants with gastroschisis. They noted that the routine use of a preformed silo was associated with similar outcomes as primary closure for infants; however, this method has not gained widespread acceptance.
Forcing the viscera into an underdeveloped abdominal cavity that cannot accommodate the herniated bowel and tight closure of the defect can restrict diaphragmatic excursion, possibly compress the lungs, and may produce abdominal compartment syndrome (ACS). ACS not only impairs respiratory function, but the high intraabdominal pressure can diminish blood flow to the kidneys and other viscera, impair venous return, and even increase intracranial pressure via effects on intrathoracic pressure (Rosenthal et al., 1998). Thus, during abdominal closure, the anesthesiologist must monitor airway pressures to identify decreased pulmonary compliance, as well as observe for evidence of decreased perfusion to the lower extremities (evidence of ACS). The surgeon and the anesthesiologist should cooperate to assess the feasibility of a primary closure. Yaster et al. (1988) noted that an increase in intragastric pressure of greater than 20 mm Hg and CVP of more than 4 mm Hg above baseline were often associated with reductions in venous return and cardiac index, requiring surgical decompression of the abdomen. The impact of ACS on hemodynamic function and its treatment with percutaneous drainage of the peritoneal cavity has been described in adults and older children, especially in the setting of trauma, and recently in a low–birth-weight infant with a sepsis and an intestinal perforation (Hunter and Damani, 2004; Hunter, 2008, Rasner et al., 2008).
An arterial catheter facilitates blood sampling and continuous monitoring of blood pressure. However, a CVP catheter might be equally valuable for evaluating changes in blood volume and the degree of visceral compression during abdominal closure, and to allow metabolic monitoring (e.g., serial intraoperative glucose levels in infants with Beckwith-Wiedemann syndrome) (Yaster et al., 1988). Intravenous fluids often consist of 5% to 10% dextrose in 0.2% saline to deliver the maintenance therapy and lactated Ringer’s solution (8 to 15 mL/kg, or more, per hour) for third-space loss. Vigilant efforts at preventing heat loss should be sustained (see Chapter 6, Thermoregulation: Physiology and Perioperative Disturbances).
After decompression of the stomach, anesthesia may be induced with inhalation or intravenous agents. Because it distends the bowel, nitrous oxide is avoided. In most cases, neuromuscular blockade facilitates the abdominal decompression or closure. Ventilation with an air (or nitrogen) and oxygen mixture with low concentrations of an inhalation anesthetic plus intravenous opioids are titrated in response to the hemodynamic status. In neonates who have received transfusions of adult blood, the inspired oxygen concentration must be adjusted to maintain oxygen saturation between 85% and 95% and lower in the preterm infant (See Chapter 17, Neonatology for Anesthesiologists). In infants who have not received adult hemoglobin transfusions, the oxygen saturation should be maintained at 90% to 97%, and in preterm infants it should be kept at between 90% and 95%. In the infant who has had no transfusion, this assumes a P50 of 19 mm Hg, and an O2 saturation between 90% and 97% results in a Pao2 of 40 to 64 mm Hg. In the preterm infant, an O2 saturation of 90% to 95% results in a Pao2 of 40 to 52 mm Hg (See Chapter 3, Respiratory Physiology, Table 3-3). Vane et al. (1994) demonstrated that spinal anesthesia can be an effective anesthetic for the repair of gastroschisis in selected patients. Evaluating for associated intraabdominal anomalies (e.g., atresia) is worthwhile, but especially in the setting of gastroschisis the “matted” bowel is difficult to examine thoroughly for other malformations.
Postoperative Care
Postoperatively, after an uncomplicated primary closure of an abdominal wall defect, mechanical ventilation is usually required for 24 to 48 hours or longer; thereafter, respiratory compliance usually improves dramatically (Nakayama et al., 1991, 1992). Clearly, infants undergoing a gradual reduction after placement of a silo or similar device should be mechanically ventilated during this process. Infants with a small defect can sometimes be extubated at the conclusion of surgery. All patients must be carefully monitored for respiratory and infectious complications in an intensive care unit after closure of an abdominal wall defect. Inferior vena caval compression (evident by blueish lower limbs) or bowel ischemia (necrotizing enterocolitis) can occur as a result of increased abdominal pressure and may require surgical decompression.
The onset of peristalsis after repair of omphalocele or gastroschisis is usually delayed, and the resulting ileus may be prolonged so that total parenteral nutrition is generally required for days to weeks postoperatively (O’Neil and Grosfeld, 1974). Bowel hypomotility is most common in infants with gastroschisis. In anticipation of this, most infants with large lesions and especially gastroschisis should have appropriate intravenous access established in the OR to facilitate early postoperative nutritional support, which is essential for healing and recovery. Prolonged feeding intolerance should precipitate an evaluation for an associated intestinal atresia or other intestinal lesion.
Outcome
Survival of infants born with anterior abdominal wall defects has improved dramatically as a result of prenatal diagnosis; improved surgical, anesthetic, and perioperative intensive care; and nutritional support. The advent of accurate prenatal imaging has allowed termination of a pregnancy when a fetus with multiple anomalies, chromosomal lesions, or a huge defect is identified. When the defect exists in isolation from other anomalies or malformations, 95% to 97% survival is expected. Mortality and long-term outcome are related to associated anomalies (e.g., cardiac malfunction or intestinal atresia); complications of treatment (e.g., bowel perforation, necrotizing enterocolitis, sepsis, or short bowel syndrome); and the side effects of intravenous alimentation (e.g., liver failure or sepsis). In a single institutional report by Phillips et al. (2008), of the patients with gastroschisis and intestinal atresia about one third have significant intestinal dysmotility without short bowel syndrome or obstruction. Although specific data have been collected to stratify and predict outcomes of patients with abdominal wall defects, an obvious observation is that infants with a simple defect (i.e., no atresias, no extraintestinal anomalies, and defect smaller than 5 or 6 cm) and no postoperative complications (e.g., obstruction, necrotizing enterocolitis, or short gut syndrome) have a better prognosis than those with a complex lesion or difficult postoperative course (Molik et al., 2001; Henrich et al., 2008). Of course, outcomes in the setting of a giant omphalocele (i.e., a liver-containing lesion through an abdominal defect wider than 5 cm) are overshadowed by a significant incidence of long-term respiratory insufficiency that may be related to sequelae of abnormal chest wall development (Tsakayannis et al., 1996; Biard et al., 2004).
In a follow-up study of 23 infants born with gastroschisis between 1972 and 1984 and who survived more than 1 year (older than 16 years of age at the time of study), Davies and Stringer (1997) reported that 22 of these 23 infants were in good health and that their overall growth was normal. About one third of the patients with gastroschisis had undergone additional surgery for adhesions, bowel obstruction, or scar complications related to their defect. In a 30-year review of morbidity related to adhesions after an abdominal wall defect in the neonatal period, van Eijck et al. (2008) reported an incidence of small bowel obstruction of 25% in the gastroschisis group, but only 13% in the patients who had had an omphalocele. Of all the patients, 88% required a laparotomy, and 85% occurred in the first year of life. A history of sepsis or wound dehiscence was a factor for predicting the development of a small bowel obstruction. The authors suggest that pediatric surgeons should evaluate the relevance of adhesion-prevention techniques and materials at the time of the initial surgical treatment of abdominal wall defects (e.g., component separation technique or hyaluronate-based barrier) (van Eijck et al., 2008).
Of note, in 25% to 60% of patients with anterior wall defects, the absence of an umbilicus was a distressing physical sign, especially during adolescence (Tunell et al., 1995; Davies and Stringer, 1997). Studies involving shorter follow-up periods have noted patients with chronic abdominal pain and gastroesophageal reflux (Fasching et al., 1996). Recently, economic analysis of care of infants with gastroschisis emphasized that the cost of care is high and that on average 47 days of hospitalization were needed in order to establish full feeds (Sydorak et al., 2002).
Congenital Diaphragmatic Hernia
Congenital diaphragmatic hernia (CDH) is a defect in the diaphragm that develops early in gestation and is associated with extrusion of intraabdominal organs into the thoracic cavity (Fig. 18-13). The incidence of CDH ranges between 1:2500 and 1:3000 live births. CDH may be an isolated lesion, or it may be associated with other anomalies. Although most cases are considered sporadic rather than part of a genetic syndrome, the nonisolated CDH has been linked to specific chromosomal anomalies including duplication of 1q24 to q31.2 and deletion of a portion of 6q, 8p23.1, and 15q26 (Holder et al., 2007; Clugston et al., 2008). In addition, CDH is a key feature of syndromes such as Beckwith-Wiedemann; coloboma, heart, atresia choanae, retardation, genital, and ear (CHARGE); Cornelia de Lange’s; and Denys-Drash. Trisomies 13, 18, and 21 and 45 are the most common aneuploidies associated with CDH. Multiple examples of familial cases have been reported, but recurrence rates are low.
The most common defect is posterolateral (Bochdalek’s hernia), occurring in 90% of cases, of which 75% are left sided. Morgagni (anteromedial) and paraesophageal hernias and eventrations make up the remainder (Fig. 18-14). This anomaly is much more than a hole in the diaphragm. CDH is also associated with the following conditions:
Since the 1980s, the survival of neonates with CDH has improved dramatically from 40% to 60% in the 1980s to 70% to 80% in the 1990s. The drastic improvement in the operative mortality rate of CDH has apparently resulted from the new strategy of delaying surgery, neonatal stabilization, and the adaptation of new a lung-protective, or “gentle ventilator,” strategy with a small tidal volume, a higher and adequate positive end-expiratory pressure (PEEP) to keep the airways open, and the acceptance of the resultant rise in Pco2 (permissive hypercapnea) (Nakayama et al., 1991; Boloker et al., 2002; Harrison et al., 2003). The development of the lung-protective approach is based on the findings that the traditional ventilatory strategy of maintaining “normal” Pco2 levels with large tidal volumes results in further damage to the lung tissues and exacerbates acute respiratory distress syndrome (ARDS) (Wada et al., 1997; Björklund et al., 1997) (see Timing of Surgery, p. 570).
CDH persists as a lesion with many unanswered questions; however, the impact of pulmonary hypoplasia and pulmonary hypertension on pathophysiology and clinical outcome is well recognized. For example, Dillon et al. (2004) correlated pulmonary artery pressure (measured via echocardiogram) with survival in a cohort of 47 full-term infants treated with delayed surgery and gentle ventilatory approaches such as high-frequency oscillatory ventilation (HFOV), permissive hypercarbia, nitric oxide (NO), or extracorporeal membrane oxygenation (ECMO). All 23 infants with estimated normal pulmonary artery pressure within the first 3 weeks of life (49% of study) survived. In contrast, none of the 8 patients with persistent systemic (or higher) pulmonary artery pressure survived. Of the remaining 16 patients with pulmonary artery pressure between these two extremes, 12 (75% of the 16) survived. Both in utero and postnatal optimum management strategies for these infants remains unclear (de Buys Roessingh and Dinh-Xuan, 2009).
Survival of inborn patients is lower (62.6%) than outborn patients (83.3%), suggesting that surviving transport to a tertiary care center occurs at a higher rate for those with less severe lesions. Since 2000, survival rates of patients who receive care at a NICU are at least 60% to 70% (Migliazza et al., 2007). Thus, institution-based reports that only include data about live-born infants cared for in an NICU may underestimate the total mortality of CDH and the incidence and severity of associated anomalies. Survival rates associated with an isolated congenital diaphragmatic hernia approaches 80% in those who do not require ECMO (Sigalet et al., 1995; Reickert et al., 1998). Harrison first noted in the 1970s that mortality may be “hidden” when in utero deaths and early postnatal deaths are not included in calculating mortality associated with CDH (Harrison et al., 1978, 1994).
A population-based study of CDH in western Australia that included complete outcome data (i.e., miscarriages, stillbirths, and terminations of pregnancies) noted that of the 116 CDH cases studied, 61% of infants were born alive (Colvin et al., 2005). Of those born alive, 35% died before transport, and 52% survived beyond the age of 1 year. However, if the denominator includes all cases, only 32% survived past 1 year. Of these infants, only 16% of all prenatally-diagnosed and 33% of live-born, prenatally-diagnosed infants with CDH survived beyond the age of 1 year, whereas 92% of infants who had surgery and 80% of those who reached the referral center survived beyond 1 year of age. A major anomaly was identified in 47% of this cohort, and mortality reached 78% in this subgroup. In those pregnancies that were terminated, 71% had a major anomaly in addition to CDH. Similar to other reports, 53% of CDH cases were identified in utero; 49% of these were terminated. Of the 47% diagnosed postnatally, 74% were liveborn. Most deaths occurred in the first week of life. Eighty-eight percent of those with a preoperative pneumothorax died. Low birth weight and low Apgar scores at 1 and 5 minutes were also predictive of increased mortality. Thus, high mortality associated with this lesion persists, and prenatal termination dramatically confounds the accurate overall estimate of the outcome of CDH.
Similar findings have been reported from the United Kingdom’s northern health region on all cases of CDH from 1991 to 2001, which included antenatal diagnoses and termination, associated anomalies, therapy, and outcome. Stege et al. (2003) claimed that in the era of gentle ventilation, various newer therapies (e.g., ECMO, HFOV, and NO) have had minimal impact on mortality when complete case ascertainment is included. The authors noted that the two factors that distort postnatal mortality include the rate of termination and the presence of other anomalies. Stege also reported that the mortality rate before ECMO was available was 68% total (57% of live births) and after ECMO was available, the mortality rate was 58% total (45% of live births). However, since discontinuing ECMO as a therapeutic option, mortality has remained at 58% overall (40% of live births). Similar patterns evolved for data about NO and HFOV. That is, changes in ventilator supportive care (i.e., gentle ventilation) of infants with CDH probably account for the decreased mortality and survival for CDH. The authors emphasize that only about 50% of CDH cases are identified on antenatal surveillance, leading to persistence of births of infants with this life-threatening lesion who are without the advantages of a tertiary level nursery care that has pediatric surgical and medical expertise.
Stillborn babies with CDH have a 95% incidence of other anomalies. A severe cardiac malformation combined with CDH implies a lethal outcome (Fauza and Wilson, 1994; Migliazza et al., 2007). Although various series’ rank the incidence of associated malformations differently, Stege et al. (2003) reported that cardiovascular lesions were most common, followed by chromosomal, skeletal, facial dysmorphism (cleft palate, ear deformity), gastrointestinal, and syndromal. Rarely, CDH is a part of a complex set of anomalies called Pentalogy of Cantrell, which consists of omphalocele, sternal cleft, ectopia cordis, and an intracardiac defect (ventricular septal defect or diverticulum of the left ventricle) (Wesselhoeft and DeLuca, 1984). Although uncommon, CDH has been associated with several other syndromes including, Fryns’ syndrome, Goldenhar’s syndrome, Brachmann-deLange syndrome, and Beckwith-Weidemann syndrome (Thornburn et al., 1970; Fryns et al., 1979; Rollnick and Kaye, 1983; Jelsema et al., 1993).
Embryology
The diaphragm, lungs, and gastrointestinal tract develop synchronously. The lungs begin as a ventral bud of the foregut. Airway development and branching begin between the fourth and fifth weeks of gestation and progress until the terminal bronchioles are formed by the seventeenth week. The ventral (membranous) component of the diaphragm is formed between the third and fourth weeks of gestation. At about the eighth week of gestation, this portion envelops the esophagus, inferior vena cava, and aorta and fuses with the foregut mesentery to form the posterior and medial (membranous) portions of the diaphragm. The lateral margins of the diaphragm are considered to be derived from the muscular components of the body wall (recent investigations in rats have questioned the contribution of the body wall). The pleuroperitoneal canals close when all the membranous portions of the diaphragm fuse together, and by the ninth week of gestation, diaphragmatic closure is usually complete (Wells, 1954). If the closure (obliteration) of the pleuroperitoneal canals is delayed beyond the ninth to tenth weeks of development, or if the normal rotation and settling of the midgut occur before the tenth week or before the obliteration of the pleuroperitoneal canals, the midgut (abdominal viscera) herniates into the thoracic (pleural) cavity.
In the past, this defect has been attributed to an abnormal closure of the pleuroperitoneal canal, but investigations using the nitrofen-induced CDH model in the rat have used an innovative approach to reexamining the etiology of this lesion. CDH seems to be linked to a disordered formation of the pleuroperitoneal fold—a much earlier event (fourth week of gestation). An abnormal formation of the framework of mesenchyme, which inhibits muscular development of the diaphragm, has been linked to abnormalities in the retinoid signaling pathway. This suggests that vitamin A may play a role in the pathophysiology of CDH (Greer et al., 2003; Holder et al., 2007). CDH was found in 20% to 40% of offspring of rodents (dams) deficient in vitamin A, suggesting a critical role of the retinoid pathway. In addition, exposure to nitrofen (inhibitor of a key enzyme responsible for conversion of retinal to retinoic acid) was associated with development of CDH.
Preoperative Management
Pathophysiology
In the most common presentation, the herniated abdominal viscera (which includes the midgut but may also include the stomach, parts of the descending colon, the left kidney, and the left lobe of the liver) occupies the left thoracic cavity and interferes with the development of the lungs. In most cases, depending on the severity of the hernia, some degree of pulmonary hypoplasia exists, the severity of which depends on when the herniation and compression occurred during fetal development. The herniation of abdominal contents shifts the mediastinum to the right, compresses the contralateral lung and, in part, contributes to abnormal development of that lung. On the other hand, simple compression of the ipsilateral and contralateral lungs fails to explain the severe morphologic derangements in lung development, such as fewer alveoli with thickened walls, smaller alveolar and gas exchange surface area, decreased vasculature with medial hyperplasia, and extension of the muscle layer into intraacinar arterioles (Done et al., 2008).
The structural abnormalities of the pulmonary vasculature correlate with the pathophysiology, mortality, and morbidity of CDH. The low number of airways, the simple arterial branching pattern, the increase in smooth muscle mass at the level of the resistance vessels, and left ventricular abnormalities add to the severity of cardiorespiratory dysfunction produced by the hernia itself (Schwartz et al., 1994). Alveolar development does occur postnatally, but growth at the preacinar level is limited in that the number of airway generations remains constant after midgestation (Geggel and Reid, 1984). Postnatal vascular remodeling provides larger and less muscular arteries, so the pathology present at birth in the setting of CDH has been documented to reverse to some degree (Beals et al., 1992). Nonetheless, the degree of pulmonary hypoplasia and hypertension is predictive not only of mortality but of the development of long term sequelae in the survivor.
The anatomic abnormalities in the pulmonary vasculature of CDH imply that persistent pulmonary hypertension will commonly accompany this anomaly. The most consistent findings include a fewer number of pulmonary arteries per unit of lung volume and peripheral muscularization of small arteries leading to thickening of the medial and adventitial layers (Geggel et al., 1985). Although small arteries (equal to 200 mcm) predominantly determine pulmonary vascular resistance (PVR), other factors also influence the pulmonary circulation of the newborn.
The fall in PVR (approximately 80%, which normally occurs in the first 24 hours of life) and rise in pulmonary blood flow that is essential for the transition from placental circulation to the postnatal pattern is dependent on adequate function of the endothelial cell (see Chapters 3 and 4, Respiratory Physiology in Infants and Children and Cardiovascular Physiology). Imbalance in the production, release, and circulating levels of vasoconstrictors (leukotrienes C4 and D4, thromboxane A2, platelet activating factor) and vasodilators (NO, prostacylin) seems to be central to the right-to-left shunting observed with pulmonary hypertension associated with CDH (Furchgott and Zawadzki, 1980; Haworth, 2006). Endothelins are also involved in the persistence of high pulmonary vascular resistance. Persistently elevated levels of endothelin 1 (ET-1) have been described in infants with CDH (Rosenberg et al., 1993; Kobayashi and Puri, 1994). In utero, ET-1 contributes significantly to maintaining normal high PVR. Postnatally, various endothelially-derived vasoconstrictive peptides are produced in response to inflammation, ischemia, and other stimuli. Multiple receptors, both in the vascular smooth muscle and in the vascular endothelial cell, mediate response to these molecules. In part, the release of these vasoactive agents is also in response to ventilator-induced epithelial and endothelial damage from hyperinflation of the hypoplastic lungs. Active remodeling of the pulmonary vascular bed continues gradually over the first 2 to 4 weeks of life.
Diagnosis and Treatment
Prenatal Diagnosis and Treatment
Prenatal diagnosis of CDH has increased from approximately 10% in 1985 to near 60% at present (Dillon et al., 2000). The most common findings include displacement of the heart and a fluid-filled bowel in the thorax. Both stomach and small bowel are often present (Garne et al., 2002). Ultrasound diagnosis of a large defect (e.g., dilated intrathoracic stomach, herniated left lobe of the liver, or polyhydramnios) suggests a severe lesion; however, prenatal ultrasounds can have a high incidence of false negatives (Lewis et al., 1997).
A variety of indices including lung-to-head circumference ratio (LHR), diameter of the proximal pulmonary artery indexed to the descending aorta, and others have been developed to estimate prognosis by evaluating the pulmonary circulation to estimate risk for severe pulmonary hypoplasia. Jani et al. (2009) noted that an LHR and intrathoracic position of the liver independently predicted poor outcome. In a study by Ruano et al. (2006), survival was estimated to be approximately 50% when the liver was herniated into the thorax. The LHR can also define extreme lung hypoplasia and predict 100% mortality (Albanese et al. 1998; Desfrere et al. 2000; Sbragia et al., 2000; Cacciari et al. 2001; Muratore et al. 2001; Boloker et al. 2002; Stege et al. 2003). However, differences in the specific method to generate the index may lead to confusion when interpreting results among various institutions. In addition to these indices, in utero magnetic resonance imaging (MRI) has been proposed as a method to define anatomy (e.g., position of the liver or volume of the lungs) and identify associated anomalies (Cannie et al., 2008).
Because the fundamental pathophysiology of CDH is pulmonary hypoplasia, various fetal surgical techniques to improve the growth of hypoplastic lungs in utero have been developed (Harrison et al., 1990, 1993, 1997). Starting with animal models in the 1980s, Harrison and colleagues gradually perfected some open fetal surgical procedures and introduced the ex utero intrapartum treatment (EXIT) procedure. The procedure was eventually abandoned in favor of a minimally invasive technique using fetoscopy to surgically occlude the trachea. Tracheal occlusion is aimed to manipulate the physiology of fetal lung development; that is, the fetal lung development is physiologically accelerated by the airway expansion that results from accumulation of lung fluid while the glottis is occluded. In a group of fetuses with moderate hypoplasia enrolled in a trial sponsored by the National Institutes of Health (NIH), the tracheal occlusion technique marginally improved the survival of the affected fetus. However, the procedure was associated with significant complications, including premature labor and delivery. At the same time, the survival of the infants with CDH with conventional postnatal managements improved (Harrison et al., 2003; de Buys Roessingh and Dinh-Xuan, 2009). Further studies of fetal endoscopic tracheal occlusion (FETO), in which the time of tracheal occlusion and release is adjusted, continue; however, the procedure remains investigational (Done et al., 2008).
Postnatal Diagnosis and Treatment
Clinical Presentation
The classic triad of CDH consists of cyanosis, dyspnea, and apparent dextrocardia. Physical examination reveals a scaphoid abdomen, bulging chest, decreased breath sounds, distant or right-displaced heart sounds, and bowel sounds in the chest. Radiographic examination of the chest shows a bowel gas pattern in the chest, mediastinal shift, and little lung tissue at the right costophrenic sulcus (Fig. 18-15).
Infants who weigh 1000 g, are born before 33 weeks’ gestation, or who have an alveolar-to-arterial oxygen gradient (P[A-a]o2) of more than 500 seldom survive (Raphaely and Downes, 1973). On the other hand, over the last two decades, antenatal diagnosis, neonatal stabilization and delayed surgery, and (most importantly) the avoidance of ventilator-induced lung injury have resulted in significant improvement in morbidity and mortality of infants with severe CDH.
Timing of Surgery
The goal of the initial management of CDH is to avoid a surgical intervention when the infant is hypoxic and acidotic and the cardiorespiratory status is labile. Optimizing hemodynamic and respiratory support rather than the specific timing of surgery contributes to improving outcome (Migliazza et al., 2007). Thus, medical management is directed to stabilizing the cardiorespiratory function by improving oxygenation to achieve a preductal saturation more than 90%, correcting any metabolic acidosis, reducing right-to-left shunting and increasing pulmonary perfusion using the least aggressive ventilation possible (Hazebroek et al., 1988). These goals underlie the rationale for some centers initiating HFOV immediately and introducing ECMO to allow pulmonary rest. Unfortunately, these maneuvers have not been predictably effective.
Surgery affects the respiratory system’s compliance. Sakai et al. (1987) reported that the postoperative compliance of the respiratory system (Crs), as measured with a noninvasive, passive mechanic’s technique, immediately decreased 10% to 77% from the preoperative value, suggesting that pulmonary dynamics often deteriorate after surgical repair of the hernia (Lesouef et al., 1984). These observations prompted a reevaluation of the practice of urgent surgery. Instead, surgery is delayed to allow transition to a stable postnatal cardiorespiratory status.
Nakayama and others (1991) evaluated pulmonary function and outcome of 22 infants with severe CDH treated either with traditional emergency repair or with preoperative stabilization for 2 to 11 days. Of 13 infants who underwent immediate repair of CDH, nine received ECMO support postoperatively, and six of the 13 infants (two after ECMO) survived (46% survival). Of nine infants whose surgery was delayed for preoperative stabilization, six immediately received ECMO support for 4 to 10 days; one with extreme lung hypoplasia died before surgery after an intraventricular hemorrhage. The other eight infants survived after surgery (89% survival). Seven days after surgery, Crs of infants in the immediate repair group did not improve from the preoperative values. In contrast, Crs increased more than 60% from their baseline values in the infants who underwent preoperative stabilization. Although the duration of mechanical ventilation was similar in the survivors of the two groups, the preoperative stabilization group had higher postductal Pao2, lower respiratory rates, lower peak and mean airway pressures, and lower fraction of inspired oxygen values (Fio2). Based on the physiologic and clinical evidence, these researchers concluded that preoperative stabilization is beneficial before the repair of CDH. Surgery should be delayed as long as 5 to 15 days until PVR has decreased and ventilation can be maintained with a low peak inspiratory pressure (less than 25 cm H2O) and Fio2 (about 0.50).
Preoperative care of an infant with severe CDH should start in the delivery room. Positive pressure ventilation by mask and bag has risks, because attempting to expand the noncompliant lungs may damage the hypoplastic lung by overdistention, as well as distend the stomach and intestines (which are in the left hemithorax), further decreasing chest compliance (Wada et al., 1997; Björklund et al., 1997). If spontaneous ventilatory efforts are inadequate, early intubation of the trachea and decompression of the stomach are important initial steps to prevent further distension of and pulmonary compression by the displaced abdominal viscera.
However, after intubation of the trachea, minimizing the trauma from positive pressure ventilation is an important ventilatory intervention. Björklund et al. (1997) found that six manual inflations with a tidal volume of 35 to 40 mL/kg in preterm lambs resulted in persistent respiratory failure and pneumothorax despite surfactant treatment. Wada and colleagues (1997) also found that ventilation of premature lambs at birth with a tidal volume of 20 mL/kg for only 30 minutes resulted in marked decreases in Crs, along with the development of poor gas exchange. In comparison, premature lambs ventilated with 5 or 10 mL/kg of tidal volumes maintained high Crs and normal gas exchange. Although most infants with CDH are born at term or near term, these data may be relevant in CHD where the pulmonary development is abnormal.
Animal studies suggest that ventilatory strategies that employ small tidal volumes maintain high Crs and better gas exchange (Björklund et al., 1997; Wada et al., 1997). Thus, current ventilatory strategies for an infant with CDH incorporate lower peak and end expiratory airway pressures and accept hypercapnea (Wung et al., 1985, 1995; Boloker et al., 2002). In general, HFOV serves as a rescue therapy; however, others advocate respiratory support with HFOV immediately at birth (Migliazza et al., 2007). Ventilation and Fio2 are adjusted to maintain a preductal saturation of 90% to 95%. The Paco2 is allowed to remain as high as 60 to 65 mm Hg (permissive hypercapnea). Crystalloid and blood products are administered to maintain intravascular volume and red–blood-cell mass. Adequate sedation is administered in efforts to minimize increase in PVR. Periods of right-to-left shunting are tolerated if the patient’s underlying hemodynamic status is stable, and vasopressors (e.g., dopamine or milrinone) are infused to maintain hemodynamic stability. In the immediate postnatal period, some infants initially respond to stabilizing maneuvers (e.g., gentle ventilation or hemodynamic support), but in some cases this early stability represents a “honeymoon period” that is followed by a sudden, often unexplained return to a state of persistent pulmonary hypertension and clinical deterioration (e.g., acidosis, hypoxemia, severe hypercapnia, pulmonary hypertension, and right-to-left shunting through the foramen ovale, and patent ductus arteriosus). If a reversion to a state of persistent pulmonary hypertension occurs, then trials of NO and HFOV are initiated and ECMO is considered.
Nitric Oxide
NO is a free radical with a short half-life, produced endogenously but often administered as an inhaled agent to take advantage of its effects as a selective pulmonary vasodilator. Although a role for NO in the treatment of pulmonary hypertension with CDH is a logical extension from its effectiveness in infants with pulmonary hypertension associated with meconium aspiration, sepsis, or congenital heart disease, clear cut effectiveness in the setting of CDH has not been demonstrated (Kinsella et al., 1992; Roberts et al., 1992; Bloch et al., 2007).
NO diffuses across the alveolar capillary membranes and binds to and thereby stimulates enzymes with transition metal centers (e.g., heme), such as cyclic guanylate cyclase. This increases cyclic GMP, activates protein kinase, and causes vascular smooth muscle to relax (see Chapters 3, 4, and 17, Respiratory Physiology in Infants and Children, Cardiovascular Physiology, and Neonatology for Anesthesiologists). Phosphodiesterases quickly metabolize NO, thereby limiting its half-life. In addition to cyclic guanosine monophosphate (cGMP)-mediated action, NO also has non-cGMP actions. For example, NO interacts directly with proteins with active thiol groups, such as those in the heart. NO’s modification of various myocardial proteins has been linked to regulation of contractility, but this action has not been well defined in the setting of CDH and neonatal pulmonary hypertension (Zimmet and Hare, 2006).
In a small series, Shah et al. (1994) noted that patients with CDH may be more resistant to the effects of inhaled NO, whereas Karamanoukian et al. (1994) noted that NO was effective in patients with hypoplastic lungs only after extracorporeal membrane oxygenation. In a study of patients treated with HFOV from birth, only 28% of those with severe pulmonary hypertension achieved reversal after NO. In general, most patients with intractable pulmonary hypertension plus lung hypoplasia do not have a predictable, dramatic response to NO. Nonetheless, because some patients do respond and the drug has limited toxicity in lower doses, NO is often used in infants with CDH who require therapy beyond gentle conventional ventilatory support (e.g., HFOV).
Even in the setting of respiratory failure without CDH, the long-term benefits of NO compared with other therapeutic interventions remain controversial. In a follow-up study of infants (with fewer and more than 34 weeks’ gestation, treated and not treated with NO) who had been enrolled in the INNOVA trial (15 neonatal units in the United Kingdom, Republic of Ireland, Finland, and Belgium), respiratory morbidity was similar when the impact of NO was analyzed. For example, wheezing and rehospitalization were common in both term and preterm infants who received and did not receive NO (Hoo et al., 2009). Clearly, neonates with severe respiratory compromise are at high risk for long-term pulmonary dysfunction.
Extracorporeal Membrane Oxygenation
A notable series of 400 patients were reported in two manuscripts by Azarow et al. (1997) in Toronto and Wilson et al. (1997) in Boston. ECMO was used as rescue therapy in Boston, and HFOV was used in Toronto. Survival in the two cities was nearly identical (53% and 55%). A 2008 Cochrane review concluded that in spite of short-term efficacy, improved long-term survival without major sequelae was not apparent with ECMO (Mugford et al., 2008). Thus, many centers now reserve ECMO as a rescue therapy for infants who have persistent preductal hypoxemia in spite of inotropic and ventilatory support (including HFOV and NO) (Bohn, 2002, 2006).
A common set-up for ECMO is a venoarterial circuit where the patient’s internal jugular vein and common carotid artery are cannulated. In this system, blood is drained by gravity from the right atrium, oxygenated through the membrane oxygenator, and returned to the patient through the arterial cannulae. During venovenous bypass, the patient’s blood returns to the membrane oxygenator by gravity, is oxygenated in the membrane oxygenator, and is then returned to the venous system. Thus, in venovenous ECMO, adequate systemic oxygen delivery depends on an effective cardiac output, whereas in infants undergoing venoarterial ECMO the bypass system can compensate for both pulmonary and myocardial dysfunction. Significant risks are associated with ECMO, including bleeding at surgical or chest tube insertion sites, intracranial hemorrhage, sepsis, hypertension, and brain death. Clearly, the underlying disease (e.g., meconium aspiration without pulmonary hypoplasia vs. CDH with pulmonary hypoplasia) influences the success rates of ECMO (Dalton and Thompson, 1992). In general, because the mortality and the likelihood of intracranial hemorrhage are higher in low–birth-weight infants, ECMO is limited to infants weighing more than 2 kg and with more than 35 weeks’ gestation, and to infants who have no evidence of prior intracranial hemorrhage.
The criteria for instituting ECMO vary, but guidelines generally include P(A-a)o2 of greater than 600 mm Hg for more than 6 to 8 hours or an oxygen index (Fio2 × mean airway pressure/Pao2) of 51 for 5 hours. Nonetheless, no selection criteria appear to be accurate in predicting survival (Newman et al., 1990; Van Meurs, 1990; Steimle et al., 1994; Bohn, 2006; Mugford et al., 2008). In view of the improved survival with gentle ventilation (including HFOV), coupled with the significant morbidity associated with ECMO, ECMO intervention has been relegated a less central role in the setting of CDH.
Intraoperative and Postoperative Management: Open Repair
Although many centers now approach repair of CDH via minimal access techniques, open repair still remains common (Guner et al., 2008a). Severe pulmonary dysfunction limits the tolerance of thoracoscopy and one-lung ventilation, making minimal access surgery difficult or impossible (see Minimal-Access Surgery in the Neonate, p. 561).
All newborns deserve meticulous attention to temperature stability in the operating room, but infants with a CDH have dramatic risks associated with hypothermia related to its effect on increasing PVR, which may increase right-to-left shunting through a PDA or the foramen ovale (see Chapter 6, Thermoregulation Physiology and Perioperative Disturbances). Hypothermia increases oxygen consumption, and in the setting of marginal cardiorespiratory function, may result in inadequate oxygen delivery and acidosis, which then further increases pulmonary vasoconstriction and worsens oxygen saturation.
The selection of specific anesthetic agents must be based on cardiorespiratory status, the location of the surgery (NICU or operating room), and the plans for intraoperative ventilatory support. Nitrous oxide is avoided in infants with CDH because many require high-inspired oxygen concentration and also because nitrous oxide can diffuse into the viscera and exaggerate lung compression. If an anesthesia machine is available, low concentrations of inhalation anesthetics (sevoflurane or isoflurane) may be advantageous in producing pulmonary vasodilation, but if effects on SVR are greater than PVR, right-to-left shunting may actually worsen. In most cases, high-dose opioids (usually fentanyl) are administered and the narcotic infusion continues into the postoperative period (Hickey et al., 1985; Hickey and Retzack, 1993).
Outcomes
The prolonged and complicated neonatal course of infants with CDH implies that these infants are at high risk for long-term pulmonary, gastrointestinal or nutritional, and neurocognitive disorders. Muratore et al. (2001) noted that therapy with ECMO and the need for a patch correlated with later significant pulmonary disease. However, non-ECMO survivors also required repeated treatment for respiratory problems secondary to ventilator-induced lung injury and the onset of reactive airways disease. For example, 52% of all CDH survivors required therapy with bronchodilators, and 41% received inhaled steroids. Approximately 25% of survivors had evidence of obstructive lung disease. Jaillard et al. (2003) noted significant long-term pulmonary complications, reporting a 22% incidence of chronic lung disease at two years of age, and the use of ECMO and need for patch repair predicted later respiratory illness. Others report higher incidence of chronic lung disease that seemed to improve over time. Nonetheless, exercise performance was described as abnormal in some survivors, and approximately 50% of adults in one study had abnormal pulmonary function (Vanamo et al., 1996). Finally, approximately 4% of survivors require tracheostomy (Muratore et al., 2001; Jaillard et al., 2003).
Growth failure, oral aversion, and gastroesophageal reflux occur in 45% to 90% of infants with CDH, and the incidence correlates with the size of the defect and need for patch repair (Committee on Fetus and Newborn, 2008). At least one third of these infants require nutritional supplements via gastrostomy or nasogastric tube. In these infants, reflux is often exacerbated by the presence of these devices.
Neurocognitive delay and behavioral disorders have been documented in the survivors of CDH, especially in those who had been supported with ECMO, with developmental delay noted in more than one third of some cohorts (Nobuhara et al., 1996). In particular, motor and language problems are common and have been detected in 60% to 70% of patients with CDH at age 3 years (Friedman et al., 2008). In these and other series, the infants treated with ECMO may have a higher incidence of significant neurodevelopmental delays (Bernbaum et al., 1995). Sensorineural hearing loss has been described in as many as 50% of patients with CDH in later infancy, even after normal screening was documented in the NICU. Recurrent or prolonged hypoxemia, exposure to ototoxic drugs (e.g., lasix, aminoglycocides), and ECMO have been suggested as causative factors (Robertson et al., 2002; Fligor et al., 2005; Erikson et al., 2009).
Tracheoesophageal Fistula and Esophageal Atresia
The incidence of TEF and esophageal atresia (EA) ranges between 1:3000 and 1:4000 births (Sparey et al., 2000). Approximately 20% to 25% of these infants have additional congenital defects. The incidence of associated defects is particularly high (50% to 70%) in the group with isolated EA but least common in infants with the H-type fistula. The most common anomalies include congenital heart disease (approximately 35%, including ventricular septal defect, atrial septal defect, tetralogy of Fallot, atrioventricular canal, and coarctation of the aorta), genitourinary disorders (about 24%); gastrointestinal disorders (approximately 24%, including duodenal or ileal atresia, malrotation, and imperforate anus); skeletal anomalies (approximately 13%); and central nervous system disorders (about 10%) (Dave et al., 1999). The term VACTERL (vertebral, anal, cardiac, tracheoesophageal, renal, limb) association refers to these anomalies, because they commonly coexist in various combinations with TEF/EA. As many as 20% to 25% of infants with esophageal atresia have at least three of the lesions included in VACTERL (Rittler et al., 1996).
Recently, a significant incidence of non-VACTERL anomalies (single umbilical artery, 20%; genital defects, 23%; and respiratory tract anomalies, 13%) were retrospectively identified from two pediatric centers in the Netherlands (de Jong et al., 2008). In spite of the significant incidence of multiple anomalies, the risk of recurrence is less than 1% in nonsyndromal TEF/EA (Goyal et al., 2006; Ioannides and Copp, 2009). However, TEF and EA are known to be common in Trisomy 18 (about 25%), Trisomy 13, and to a lesser degree, Trisomy 21. As with CDH, the wide mining of databases and adaptation of techniques such as fluorescent in situ hybridization (FISH) and whole genome arrays have allowed identification of rare and small chromosomal abnormalities (e.g., partial trisomy 3p, partial deletion 5p, distal 13q deletion, interstitial deletions of 17q) in some cases of TEF/EA, but no specific regions have been consistently linked to these anomalies (Felix et al., 2007). Thus, at this point, the etiology seems to be multifactorial and sporadic in the nonsyndromic version. Familial and syndromic patterns occur in fewer than 1% of the cases. Finally, exposure to various teratogens has not correlated consistently with TEF/EA.
A variety of other lung anomalies (e.g., lobar agenesis, pulmonary hypoplasia, horseshoe lung, and absence of a bronchus to the right upper lobe) have been reported in the context of TEF/EA, and some experts have proposed that the lesion should be termed “general foregut malformation” (Kovesi and Rubin, 2004). In addition, TEF/EA has been identified in the setting of DiGeorge’s and Down syndromes as well as with CHARGE association.
More recently, within the same three groups, survival rates improved to 98%, 82%, and 50%, respectively (Lopez et al., 2006). Clearly, the spectrum of presentation from an isolated TEF/EA to a multisystem or chromosomal disorder has major implications for mortality and morbidity, as well as for treatment options and surgical and anesthetic management.
Embryology
The embryogenesis of TEF/EA remains incompletely defined, but several theories have been proposed (Ioannides and Copp, 2009). It is known that the trachea and esophagus develop from the foregut in the first 4 to 5 weeks of gestation. One theory suggests that the respiratory system results from a rapid outgrowth from the foregut. That is, the tracheal primordium buds off the foregut and remains a separate structure during subsequent development. Another theory focuses on active growth of mesenchymal septum that divides the foregut into ventral (respiratory) and dorsal (gastrointestinal) structures. The septum has been considered to begin at the distal site (bronchopulmonary buds), but in fact such a structure has not been clearly identified in studies of tracheoesophageal development. Other theories seem to combine segments of each of these two theories, proposing that the trachea and esophagus separate as a result of foregut folds. Ioannides et al. (2002) suggested that the physical separation of the original foregut is a critical component of the organogenesis of the esophagus and trachea. On the other hand, differentiation of both respiratory and esophageal components usually does not occur in the setting of TEF/EA.
At the molecular level, aberrant signaling in the sonic hedgehog pathways and other regulatory genes has been linked to TEF/EA in several studies (Veraksa et al., 2000).
Hedgehog proteins are signaling glycoproteins involved in many processes of early development, including embryogenesis of the foregut as well as development of the craniofacial structures, heart, and great vessels. Of the multiple hedgehog proteins, only sonic hedgehog (Shh) is expressed in mammalian gut and lung. Mutants for Shh and for the Gli proteins (transcription factors) have been noted with tracheoesophageal malformations and various other well-described associated anomalies (e.g., VACTERL) (Kim et al., 2001).
In studies examining the expression of thyroid-transcription factor-1 (TTF-1) known to be specific to the respiratory tract, the distal esophagus in TEF/EA was proposed to be of tracheal origin. TTF-1 expression localizes to the lung bud but not to the esophagus early in gestation. TTF-1 is expressed in the fistula tract throughout gestation. Based on this work, the distal “esophagus” might be of embryonic lung origin. This finding, in part, could explain the pathophysiology and clinical findings of TEF/EA: poor esophageal motility, gastroesophageal reflux, esophageal stenosis, and pseudostratified columnar (respiratory epithelium) rather than squamous epithelium (esophageal epithelium) (Crisera et al., 1999a, 1999b). Other explanations for abnormal esophageal motor function in TEF/EA focus on the abnormal innervation of the esophagus. For example, dysplasia of both Meissner’s plexus (submucosal nerves) and Auerbach’s plexus (myenteric) occur along with an abnormal distribution of mitochondria, and high levels of vasoactive intestinal polypeptide and NO synthase (NOS) (Li et al., 2007).
Anatomy of TEF
The anatomic variations of TEF have been well described and, in most cases TEF and EA occur together (Types B, C, E) (Fig. 18-16). The most common lesion (more than 90% of cases) is type C, in which a fistula exists between the trachea and the lower esophageal segment at a point slightly above the carina, and the upper esophageal segment ends blindly in the mediastinum at the level of the second or third thoracic vertebra.
Prenatal Diagnosis and Clinical Presentation
TEF should be suspected in the presence of maternal polyhydramnios, which may result from esophageal obstruction that prevents the swallowing of amniotic fluid. Preterm labor may then develop as a result of the excessive intrauterine volume. However, in most cases polyhydramnios is a nonspecific sign and develops idiopathically; congenital anomalies account for only 10% to 20% of cases (Dashe et al., 2002). For example, polyhydramnios can develop secondary to impaired fetal swallowing because of abnormal central nervous system function, including weakness associated with neuromuscular dysfunction. Unfortunately, polyhydramnios and small or absent fetal stomach bubble are the most common ultrasound findings associated with TEF/EA. These have a low positive predictive value, and false positives are common (Houben and Curry, 2008). Similarly, when TEF accompanies EA, amniotic fluid can pass into the stomach from the trachea, usually enough to form a stomach bubble but not enough to prevent polyhydramnios. In addition, polyhydramnios is rarely identified before about 24 weeks’ gestation (Pretorius et al., 1987). A dilated blind-ending upper pouch of the esophagus (upper pouch sign) has been described on ultrasound, but as with MRI studies, it has not been deemed an accurate prenatal method for diagnosing TEF/EA (Houben and Curry, 2008). Overall, the prenatal detection rate is 40% to 50%. The significant incidence of coexisting abnormalities and syndromes (e.g., Trisomy 18) implies that an in utero diagnosis should precipitate karyotyping and an intense search for other structural anomalies. As with other severe congenital anomalies, prenatal diagnosis of TEF/EA may improve outcome by allowing optimal prenatal counseling and postnatal treatment. Unlike with CDH, supportive interventions for TEF/EA are less urgent and the implications of birth outside a major medical center are less risky, so prenatal diagnosis, although ideal, is of somewhat less critical significance.
Postnatal diagnosis depends on the specific anatomy of the TEF/EA, except in the case of an H-fistula, when the newborn infant exhibits excessive salivation, drooling, cyanotic spells, swallowing problems, and coughing, which is relieved to some extent by suctioning. The diagnosis of EA can be confirmed in the delivery room by the inability to pass a catheter down the esophagus into the stomach. When a radiopaque catheter is used, a radiograph reveals the catheter in the blind upper pouch. If a TEF is present, a plain radiograph of the chest and abdomen reveals air or gas bubbles in the stomach and intestines that have entered through the fistula (Fig. 18-17). Ultrasonography is important in the diagnosis of any associated cardiac, renal, or genitourinary abnormalities. Often, H-type fistulas escape diagnosis until an infant, child, or adult is evaluated for recurrent pneumonia.
Intraoperative Management
Surgical Technique
Unless the aortic arch is right-sided, the surgical approach for open repair is through a right thoracotomy using a posterolateral extrapleural approach. On occasion, the distal portion of the esophagus is either absent or too short to reach the proximal segment. Type C (EA with a TEF between the distal esophagus and the trachea) is most commonly associated with a gap short enough to allow a primary repair, and Type A (EA) is least commonly associated with such a gap. Usually, at some point preoperatively, the gap between the proximal and distal esophagus is measured. However, the methods to assess the gap range from simple radiographic contrast studies (“unstressed”) to images with metal dilators inside the proximal and distal pouches (if a gastrostomy is present). In any case, the measurement only serves as an estimate for operative approach. In general, a gap smaller than two vertebral bodies is considered appropriate for a primary repair. If the gap is between two and six vertebral bodies, a delayed anastomosis might be considered, and if it is greater than six vertebral bodies, some would suggest that primary anastomosis is impossible. Others propose various methods to induce growth. In some series, EA with a long gap is defined in terms of centimeters between the ends. A gap of longer than 2.5 cm is sometimes considered the starting point for the category of long-gap EA (Foker et al., 2005). Thus, comparing approaches and results among series remains challenging because definitions and methods to measure the gap vary.
In cases in which a delayed surgery is planned, surgeons have traditionally ligated the fistula and inserted a gastrostomy, and some have also exteriorized the upper pouch through an esophagostomy. The baby can receive nutrition via the gastrostomy until surgery. In most cases, definitive repair is undertaken between 3 and 6 months of age, depending on the infant’s status (e.g., growth and cardiorespiratory status) and the opinion of the surgeon. At that time, the two esophageal segments are either directly anastomosed or surgically bridged with an interposed bowel segment or gastric tube graft. In many centers, these traditional approaches have been replaced with those focused on inducing growth of the esophagus or simply waiting for spontaneous growth. The goal is to accomplish a primary anastomosis within the first few months of life, aiming to avoid the high incidence of reflux, dysmotility, and other associated complications using nonesophageal tissue to close the gap (see Outcomes, p. 578).
Techniques to lengthen the native esophagus in order to shorten the distance between the proximal and distal ends have remained controversial, but in some cases they have allowed primary anastomosis (Foker et al., 2009). Foker et al. (2005) described inducing esophageal lengthening by placing external or internal traction sutures to induce rapid growth, creating an anastomosis of the esophageal ends without disruption of the wall by myotomies, and placing the esophageal junction below the diaphragm. In most cases, normal growth of the esophagus has been induced, with traction allowing increases in width as well as length. Often at least two thoracotomies are required in this approach; the same small incision (3 cm) is used, thus sparing the seratus anterior muscle, minimizing the incidence of rib fusion, and decreasing both short- and long-term complications (Foker et al., 2009). Other techniques to induce growth of the esophagus have been proposed, and recently thoracoscopic elongation of the esophagus has been reported (van der Zee et al., 2007). In some cases, simply delaying the primary closures for several weeks or months allows adequate growth (i.e., no tension either internally or externally).
Airway Management
A key decision for the surgeon and anesthesiologist involves whether one-lung ventilation would facilitate the surgical procedure, and if so, appropriately select those patients who are likely to tolerate the technique (see Minimal-Access Surgery in the Neonate, p. 561). If tolerated, collapse of the lung may improve surgical exposure, but in some cases an unacceptable fall in oxygen saturation (in spite of high Fio2 and continuous positive airway pressure [CPAP] to the nonventilated lung) requires a return to two-lung ventilation. Thus, it may be illogical to commit significant time to bronchoscopy, and in some cases doing so may needlessly prolong the operative time. Finally, even with initial ideal positioning of the endotracheal tube, in some patients ventilation through the fistula still occurs, especially if high peak airway pressures are required.
After the endotracheal tube is in its “assigned” position (i.e., one- or two-lung ventilation), end-tidal carbon dioxide and oxygen saturation should be monitored, and the stomach and chest should be auscultated to ensure that the lung(s) are adequately ventilated. Ideally, the stomach is not distended with inspired gases (i.e., ventilation does not enter the stomach via the fistula, or the fistula itself has not been intubated). Salem et al. (1973) suggested distal positioning of the endotracheal tube, with the bevel facing anteriorly and the posterior wall of the endotracheal tube occluding the fistula, but this maneuver is challenging to achieve and maintain.
Although precordial stethoscopes have been relegated to secondary rank because monitoring oxygen saturation and end-tidal carbon dioxide have become standards of care, a precordial stethoscope can contribute importantly to intraoperative care during repair of TEF. The precordial stethoscope can be positioned in the left axilla (i.e., during a right thoracotomy) to allow breath-to-breath monitoring of adequate ventilation to the dependent lung. Ideally, in infants with unstable cardiorespiratory status or congenital heart disease, an arterial catheter (umbilical or radial) should be available. In the setting of right-to-left shunting via a patent ductus arteriosus, pre- and postductal oxygen saturation monitoring with two pulse oximeters may provide critical data about shunting and pulmonary hypertension (see Congenital Diaphragmatic Hernia, p. 567).
A gastrostomy may serve as a low-resistance vent through which some of the tidal volume escapes, especially if high peak airway pressures are needed to ventilate noncompliant lungs and the chest wall. Although inserting a Fogarty catheter (or other ballooned device) retrograde via the gastrostomy or via a bronchoscope into the TEF has been reported, this technique is often impractical in the setting of small infants (Filston et al., 1982; Karl, 1985). Precise positioning of a catheter so that the balloon is occluding only the fistula is difficult to achieve, and even if the positioning is perfect on initial attempt, maintaining this precise position of the catheter for any length of time is almost impossible. Furthermore, displacement of the balloon can be disastrous (e.g., occluding the trachea). Finally, a high-pressure balloon may impinge on small pulmonary vessels or airways, compromising pulmonary blood flow or ventilation.
Diaz et al. (2005) reported the incidence of intraoperative critical events (as well as overall survival) in open-surgical TEF/EA repair in patients with and without congenital heart disease (excluding patent ductus and or patent foramen ovale). In this report, all patients with ductus-dependent lesions were mechanically ventilated preoperatively, and the incidence of clinically important events was higher in this group, even when compared with infants who had less significant heart anomalies. Difficulty with intubation and oxygen saturation secondary to lung retraction, plugging of the endotracheal tube, and hypotension dominated the events. Perioperative mortality in the highest risk group was 23%, consistent with the survival rates (82% survival, even with a major cardiac lesion) as reported by Lopez et al. (2006), using a three-tiered risk assignment.
Outcomes
GER is probably the most common and significant long-term problem after TEF/EA. GER is recognized in at least 40% of patients (35% to 58%), and is more common after anastomosis under tension, after gastrostomy, and after delayed primary repair (Kovesi and Rubin, 2004; Spitz, 2006). GER seems to correlate with many of the long-term problems associated with TEF/EA: strictures; pulmonary disease (e.g., recurrent aspiration pneumonia, reactive airways disease, and parenchymal disease); feeding problems and oral aversion; and poor growth. Follow up and evaluation of GER are critical for all survivors of TEF/EA.
Esophageal dysmotility/disordered peristalsis and abnormal lower esophageal sphincter function is recognized in at least 75% of patients with EA and in all children who have undergone delayed repair with interposition procedures. In childhood, choking episodes are common. Many children eat slowly and often compensate by drinking water and other liquids during meals. Choking episodes may be associated with apnea and cyanotic spells. Esophageal obstruction may develop, and some patients require removal of impacted material. Although dysphagia may persist into adulthood in 10% to 30% of patients, symptoms seem to dissipate after the first decade of life (Kovesi and Rubin, 2004).
Tracheomalacia and other abnormalities of the trachea also define long-term outcome after TEF/EA. Although common, tracheomalacia appears to be clinically significant in only about 10% of patients (Spitz, 2007). Tracheomalacia tends to improve after the first 3 to 5 years of life, and is rare in isolated EA. Tracheomalacia is generally identified at or just above the site of the TEF, and therefore is most prominent in the lower one third of the trachea. The trachea seems to have an excessive membranous portion that is prone to instability. A brassy cough (“TEF cough”) is common in infants and children but rarely persists into adulthood. When symptoms are severe, stridor may develop. Treatment for tracheomalacia is required only in patients with severe symptoms (e.g., apnea, recurrent pneumonia, or worsening stridor) and usually involves an aortopexy (i.e., suturing the aorta to the posterior surface of the sternum), which draws the anterior trachea forward as the posterior aspect of the aorta adheres to the trachea. Recently, a stenting procedure has been effective in treating tracheomalacia and may be less invasive than aortopexy (Kovesi and Rubin, 2004).
In addition to the major structural abnormalities, the epithelium at the site of the TEF (but at times in all the major airways) can be abnormal, lacking both cilia and goblet cells, and thereby decreasing mucociliary clearance (Goyal et al., 2006). This lesion may be exacerbated by or be secondary to GER. Rarely, secretions may be retained to the point of inducing tracheal metaplasia. A blind pouch at the site of the former TEF can be a nidus for trapping secretions.
Recurrent upper and lower respiratory infections occur in 35% to 75% of infants and children, especially throughout the first 3 to 4 years of life, and they may be related to GER or impaired mucociliary clearance secondary to the abnormal tracheal epithelia (Dudley and Phelan, 1976; Spitz, 2007). Wheezing commonly accompanies these infections (Chetcuti and Phelan, 1993). Even with decreased symptoms over time, pulmonary function studies 6 to 37 years after repair show a high incidence of obstructive and restrictive forms of lung disease (Chetcuti et al., 1992; Robertson et al., 1995). Specifically, abnormal expiratory flow rates (e.g., low forced expiratory volume at 1 second [FEV1] in 25%, low forced expiratory flow 25%-75% [FEF25%-75%] in 14%), reduced lung volume (e.g., low vital capacity in 15%), and airway hyperreactivity have been documented in survivors.
Open posterolateral thoracostomy in infants has been associated with musculoskeletal malformations, including “winged” scapula and chest-wall asymmetry (see Minimal-Access Surgery in the Neonate, p. 561). Scoliosis has been attributed to both the thoracostomy and associated vertebral anomalies. Scoliosis would predictably reduce total lung capacity, so in survivors of TEF/EA, compromise may be exaggerated when a structural chest-wall deformity is added to other sources of pulmonary dysfunction.
Necrotizing Enterocolitis
Necrotizing enterocolitis (NEC) persists as a major source of perinatal and life-long morbidity and mortality, primarily affecting premature infants with a gestational age of fewer than 36 weeks (usually fewer than 32 weeks), and particularly virulent in ELBW infants. In its acute and severe phase, NEC is a gastrointestinal emergency. Although centered in the gastrointestinal tract, NEC is a systemic process primarily related to the sepsis that accompanies intestinal necrosis and increased mucosal permeability. Isolated intestinal perforation (IP) refers to a clinical scenario that some suggest is distinct from NEC (Pumberger et al., 2002). Of note, IP seems to occur earlier and with lower incidence of pneumatosis and pneumoperitoneum, and in the ELBW infant it may have a lower mortality (38.3%) compared with that of NEC (55.2%) (Blakely et al., 2005). Others have reported that early exposure to indomethacin (but not prenatal steroids) plays a role in the etiology of IP (Attridge et al., 2006). The two entities are difficult to accurately differentiate preoperatively, so they are treated similarly—both medically and surgically. This discussion centers on “classic NEC.”
In spite of intense basic, clinical, and epidemiologic research, the precise etiology of this life-threatening and common disease is unknown and considered to be multifactorial. The only clear-cut risk factor is prematurity. At least 90% of infants diagnosed with NEC are premature, and severity of symptoms, complications, and mortality are inversely related to gestational age (Hsueh et al., 2002). Of note, 11.5% of infants weighing 401 to 750 g, 9% of those between 751 and 1000 g, 6% of those between 1001 and 1250 g, and 4% of those between 1251 and 1500 g are affected (Guillet et al., 2006). As the survival rate of premature infants, especially ELBW infants, has improved, the incidence of NEC has increased in some populations. Although enormous progress in the delivery of neonatal care is apparent, estimates of incidence (10% to 20% in infants weighing less than 1500 g), requirement for surgery, and mortality associated with NEC have remained remarkably static. In part, the increased number of susceptible infants may explain the lack of improved overall survival in infants with NEC. Recent data note that 1 to 5 of every 1000 live births in the United States are diagnosed with NEC, and 1% to 7% of all patients admitted to the NICU and approximately 10% of VLBW infants are affected (Hsueh et al., 2002; Guillet et al. 2006; Holman et al., 2006; Lin and Stoll, 2006; Hunter et al., 2008; Lin et al., 2008b). Mortality ranges from 15% to 30% and is highest among the most immature and those treated surgically (30% to 50%). Between 20% and 40% of patients are treated surgically.
Bell et al. (1978) described three stages of NEC. Stage I refers to mild disease, with the infant having only nonspecific symptoms (e.g., vomiting, gastric residuals, apnea, bradycardia, and guaiac-positive stools). There is no definitive radiologic evidence for NEC, and the state of the bowel is completely unknown. Stage II includes infants with definitive NEC. They have clinical symptoms similar to the infants in Stage I, but radiographs show that they have pneumatosis intestinalis or portal venous air (Figs. 18-18 and 18-19). Stage II infants are suitable candidates for medical management. Stage III includes infants with advanced disease. These infants have evidence of intestinal necrosis or perforation along with clinical signs of hemodynamic, respiratory, and hematologic instability. Although these three stages are not distinct and represent a continuum of clinical disease, the three-stage concept helps define management strategies. Walsh and Kleigman (1986)expanded the classification, but the primary framework of suspected, definite, and advanced disease persists. Nonetheless, some have recommended abandoning Bell’s criteria in favor of new guidelines, because NEC is not a uniform disease entity (i.e., does not have a specific etiology) and the pathophysiology remains incompletely defined (Table 18-1) (Gordon et al., 2007).
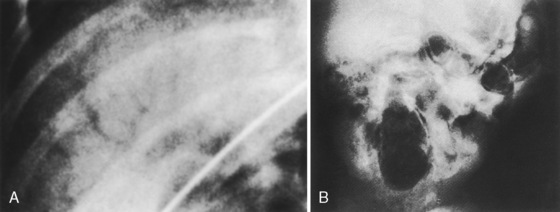
FIGURE 18-18 Air pattern in the portal venous system of the liver in an infant with NEC.
(From Rowe MI: Necrotizing enterocolitis. In Welch KJ et al., editors: Pediatric surgery, ed 4, Chicago, 1986, Year Book.)
Pathophysiology
NEC is a paradoxical disease. Infants with milder disease usually recover with outcomes similar to those of other infants of the same gestational age, but severely affected infants who require surgery have high mortality, and those who survive usually develop significant gastrointestinal and neurodevelopmental morbidity. Although miniepidemics do occur, most cases of NEC are sporadic and cannot be correlated with any specific infectious agent. The disease only occurs postnatally (not in stillborns), and it is rare in full-term infants and infants who have never been fed (Hsueh et al., 2002). The full-term infant who does develop NEC tends to display symptoms and signs in the first 1 to 3 days of life, sometimes before feeding has been initiated. There is usually a history of a hypoxic and ischemic events such as perinatal asphyxia, respiratory distress, or congenital heart disease. In contrast, the preterm infant tends to develop symptoms and signs after 2 to 3 weeks of life after feeding has been initiated, often without any preceding dramatic cardiorespiratory event.
The most common anatomic site for NEC is the ileocolic region. However, NEC is often discontinuous, with patchy distribution in both the small and large intestines in as many as 50% of cases (Fig. 18-19). Large bowel involvement is most common in the full-term infant. Perforations often are multiple and although commonly identified at the junction of a necrotic and normal bowel, perforations are also found within the affected areas.
The primary pathologic findings in NEC are coagulative or ischemic necrosis, as well as inflammation. The inflammatory response seems to be unique in that abscesses do not form, as seen in inflammatory bowel disease, infectious colitis, or acute arterial occlusion (Hsueh et al., 2002). The combination of ischemia and bacteremia seems to underline the pathophysiology of the disease. For example, NEC does not evolve after an episode of vascular injury in utero, when the bowel is sterile (Musemeche et al., 1986). Instead, an intestinal atresia or stenosis may develop. The formation of gas bubbles (pneumatosis intestinalis) reflects fermentation of an intraluminal substrate by bacteria; however, NEC is not associated with a specific organism or with particularly virulent bacteria. A wide range of organisms have been identified in the stool of infants with NEC, some who also have a bacteremia with the same organism: Escherichia coli; various strains of Enterobacter, Klebsiella, and Pseudomonas; coagulase-negative Staphylococci; and others. Viral agents have been associated with NEC but are not considered a major infectious etiology.
Classically, although the hypothesis for the etiology of NEC has focused on circulatory insufficiency or infection, the pathophysiology of NEC in the preterm infant also involves an exaggerated inflammatory response in the setting of abnormal bacterial colonization, an inadequate epithelial barrier (e.g., defects in mucus or immature tight junctions), an immature intestinal motility (e.g., disordered peristalsis), and an immature gastrointestinal immunity (Hunter et al., 2008). In fact, the immature gastrointestinal tract is characterized by inadequate secretion and production of mucin, which may predispose it to increased permeability to and adherence of bacteria. The immature immunity of the preterm intestine increases the susceptibility of an inadequate barrier to injury from uncontrolled inflammatory responses.
Activation of inflammatory and apoptotic pathways and antiapoptotic mechanisms relies on the interaction of systems in the invading organism with specific receptors in the host. So-called microbial-associated molecular patterns (MAMPs) interact with a specific pattern-recognition receptor (PRR) on host cells. A well-known PRR is the human toll-like receptor 4 (TLR4), a receptor for lipopolysaccharide (LPS), a substance in the cell walls of some bacteria. Of relevance to the preterm infant, hypoxia and exposure to infant formula may exaggerate expression of this receptor. Adult human intestine is characterized by minimal TLR4 expression, but expression in fetal intestine is high. The overexpression of the normal TLR4 receptor may predispose the immature intestine to enhanced proinflammatory injury; interaction with MAMPs of pathogenic bacteria leads to apoptosis and inhibition of healing from injury (Leaphart et al., 2007). Apoptosis of the intestinal epithelia has been noted in human infants with NEC (Ford et al., 1997). Thus the preterm infant may be predisposed to NEC via an inability to maintain adequate inflammatory responses while avoiding overactivation of proinflammatory responses (e.g., via TLR4 receptors), which lead to injury.
Although multiple inflammatory markers and various cytokines (IL-8, IL-10, liver and intestine fatty acid binding protein) have been associated with NEC, no single marker has been identified that allows early diagnosis and management of NEC (Young et al., 2009). Several “models” of NEC, emphasizing the role of inflammatory and vasoactive mediators, platelet-activating factor (PAF), and tumor necrosis factor-α (TNF α) have been developed (Fong et al., 1990; Sun and Hsueh, 1991; Sun et al., 1996; Wand et al., 1997; Tan et al., 2000). However, no experimental approach has been ideal, because models for this disease do not exactly mimic the clinical disease (Sodhi et al., 2008).
Nonetheless, by correlating clinical studies to experimental models, it appears that similar to sepsis, NEC is driven by the pathway of activation of cytokines, prostaglandins, and NO, which interact to initially induce intestinal injury via polymorphonuclear neutrophil (PMN) activation and PMN adhesion (Fig. 18-20). PAF and TNF α seem to play a central role in inducing intestinal injury.
One theory is that PAF induces cell injury after inducing production of various reactive oxygen species (ROS), but the precise mechanism is unclear. Of note, the xanthine dehydrogenase/xanthine oxidase system is abundant in the intestine and seems to generate superoxide during ischemia. Elevated levels of PAF have been measured in infants with NEC.(Rabinowitz et al., 2001). In addition, premature infants have low levels of the PAF-degrading enzyme acetylhydrolase (Caplan et al., 1990). Exactly how other mechanisms included in the pathogenesis of NEC (e.g., immature immunologic mechanisms, abnormal patterns of bacterial overgrowth, and deficient mesenteric blood flow regulation) interact with the intense inflammatory response is not completely understood. Finally, differences in the content of sialic acid and N-acetylglucosamine residues of the mucosa may affect anatomy and function of the microvilli, leading to certain types of bacterial colonization, bacterial adhesion, and eventually, permeation of the gastrointestinal tract (Claud and Walker, 2001).
Another pathway that plays a critical role in the pathophysiology of NEC centers on the interaction of NO, prostaglandins, and epidermal growth factor (EGF) in regulating epithelial function. Prostaglandins function in maintaining tight junctions. Normal low levels of NO regulated by endothelial nitric oxide synthase (NOS) activity enhance blood flow to the mucosa and maintain microvascular flow. However, high levels of NO in response to stimulation of inducible NOS may cause cytotoxicity as a result of damage to the epithelial barrier (disrupting epithelial tight junctions) and excessive apoptosis, as seen in early NEC (Ford et al., 1997; Chokshi et al., 2008). Thus, although NO seems to be critical in maintaining mucosal blood flow, high levels may have injurious effects, disrupting the tight junctions of the epithelium and inducing abnormal apoptosis. In addition, endothelin (a vasoconstrictor) is released in response to various pathologic conditions, such as NEC. EGF seems to induce migration and proliferation of epithelial cells in response to injury, but levels of this mediator are low in some preterm infants, specifically those with NEC. The cytoprotective effect of EGF, which is found in high levels in breast milk, may eventually be incorporated into nutritional strategies to prevent and treat NEC (Guner et al., 2008b; Nair et al., 2008).
Finally, various genetic factors (i.e., prematurity, respiratory distress syndrome, and periventricular leukomalacia) may interact either directly or indirectly in the development of NEC. For example, recent analyses of preterm twins uncovered a familial origin for NEC, as well as for intraventricular hemorrhage (IVH) and bronchopulmonary dysplasia (BPD) (Bhandari et al., 2006). That is, 52% of the variance for NEC could be explained by genetic and shared environmental factors. The TLR4 gene and its products have been reported to be higher in an animal model of NEC, and the risk for NEC has been associated with a specific genotype of interleukin (CPS1 T1405N polymorphism, IL-18[607]) (Jilling et al., 2006). The incidence of the genotype was significantly higher in those with stage III compared with those who had stage I or II NEC (Moonen et al., 2007).
The observation that inflammation is the result of NEC, rather than the cause, has prompted a variety of interventions aimed at enhancing epithelial integrity, such as human milk feeds, various amino acid supplementations, and various probiotics, prebiotics, and postbiotics (Lin et al., 2008a). Although a specific role for any agents other than human milk remains unclear, any introduction of pharmacologic agents may have dramatic effects on other therapeutic agents used in the NICU and the operating room.
Preoperative Management
In “classic NEC,” gastrointestinal signs appear between the first and tenth days of life in more than 90% of cases, and they include abdominal distention, retained gastric secretions and high gastric residuals after feeding (may be bile-tinged), vomiting, bloody or mucoid diarrhea, and occult blood in stools. Figure 18-21 shows the probability of a patient experiencing intramural gas, bloody stools, or portal venous gas when diagnosed with NEC vs. gestational age. In some cases, these signs are preceded by nonspecific abnormalities such as apnea, bradycardia, thermal instability, or lethargy, with a gradual development of gastrointestinal problems. In other infants, the course is fulminant with rapid onset of abdominal signs with acidosis, hemodynamic instability, and disseminated intravascular coagulation (i.e., septic shock), often with bowel perforation. In infants with acute onset of advanced NEC, surgical intervention is emergent. Many infants with obvious NEC have decreased platelet counts (50,000 to 75,000/mm3 or lower) and prolonged prothrombin and partial thromboplastin times. Some infants have leukocytosis with a high percentage of bands, but others develop profound leukopenia and neutropenia. Early in the disease process, abdominal radiographs may reveal dilated, fixed (adynamic ileus) loops of bowel, but in advanced NEC, pneumatosis (intramural air in the intestine), gas in the portal venous system, and pneumoperitoneum develop.
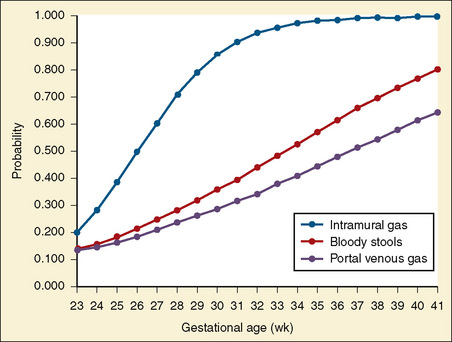
FIGURE 18-21 Probability curves for symptoms of bloody stools, intramural gas, or portal venous air in babies with NEC.
(From Sharma R et al.: Clinical manifestations of rotavirus infection in the neonatal intensive care unit, Pediatr Infect Dis J 21:1099, 2002.)
Without evidence of intestinal necrosis or perforation, the initial treatment for NEC is nonoperative. Decompressing the stomach with an orogastric tube, no feeding, administering fluid and electrolyte therapy (including parenteral nutrition), delivering broad-spectrum antibiotics after obtaining cultures of blood and urine, and correcting hematologic abnormalities are the main components of medical therapy. Supportive hemodynamic therapy, including inotropic agents and steroids, may be needed to treat septic shock. Bowel perforation is the only generally accepted indication for surgery, but relative indications may include peritonitis, air in the portal system, bowel wall edema, ascites, and a progressively deteriorating cardiorespiratory status (Box 18-2).
The role for primary peritoneal drainage in the surgical treatment of NEC and IP has been scrutinized. Peritoneal drainage was initially proposed as a method to treat the most unstable infants with NEC, usually ELBW infants (Ein et al., 1977). Some have reported that this intervention provides advantages in the ELBW infants but not in those weighing more than 1500 g (Azarow et al., 1997). Theoretically the ELBW infant may benefit from such a temporizing procedure that would allow resuscitation and stabilization. However, the effectiveness of peritoneal lavage in “buying time” for an infant to achieve hemodynamic, acid/base, and hematologic stability is actively debated.
In a prospective cohort of 16 NICUs (NICHD Neonatal Research Network), the overall in-hospital mortality associated with NEC in this group of ELBW infants was 49%. Poor prognosis was associated with lower gestational age and birth weight, preoperative vasopressors, and HFOV support. Mortality was 55% for patients with NEC and 38% for those with IP. However, in this study, initial treatment with a peritoneal drain vs. laparotomy was at the discretion of the surgeon (i.e., subject to bias), therefore mortality could not be accurately correlated with surgical management (Blakely et al., 2005). Subsequently, a multicentered randomized trial compared outcome of primary peritoneal drainage with laparotomy in another group of preterm infants. In this study, the infants were lighter than 1500 g and had fewer than 34 weeks’ gestation (compared with a weight of less than 1000 g in the earlier study), who had free intraperitoneal air either on abdominal radiograph or by clinical examination (by both the surgeon and neonatologist), or had stool, bile, or pus on paracentesis. Because of clinical deterioration, 5 of the 55 (9%) in the peritoneal drainage group underwent laparotomy. Because of stricture, obstruction, or feeding intolerance, another 16 (29%) underwent laparotomy. Mortality at 90 days was similar (peritoneal drainage, 34.5%; laparotomy, 35.5%), as was dependence on intravenous nutrition (peritoneal drainage, 47.2%; laparotomy, 40%). Length of hospitalization did not differ between the groups. Of note, the investigators did not uncover a difference in mortality when outcome was stratified according to gestational age subsets (younger than 24, 25 to 26, 27 to 30, and 30 to 34 weeks’ gestation) (Moss et al., 2006).
In a multicenter randomized trial including 31 NICUs in 13 European countries, ELBW infants with pneumoperitoneum on radiograph either underwent laparotomy or placement of an intraperitoneal drain. Survival at 6 months was similar (51.4%, intraperitoneal drain; 63.6%, laparotomy). In contrast to Moss et al.’s (2006) study, 74% of infants underwent laparotomy between 0.4 and 21 days. These investigators suggest that peritoneal drainage is ineffective as a temporizing measure or as a definitive treatment. That is, if a drain is placed, a laparotomy should be performed soon afterward (Rees et al., 2008).
Outcome
A devastating complication in survivors of NEC is short bowel syndrome, which develops in as many as 20% to 25% of surgically treated patients (Rees et al., 2008). In these cases, the bowel resection is so extensive that the infant is unable to establish enteral feedings, implying a need for long-term intravenous alimentation despite its high-risk morbidity (e.g., sepsis and hepatic failure). Although site and amount of resection seem to correlate with the development of short bowel syndrome, diffuse mucosal injury may persist in areas of bowel injured by NEC but not resected. Current interventions include intestinal lengthening procedures or even bowel transplantation. The use of tissue-engineered small intestine is under intense investigation (Guner et al., 2008b). Evidence suggests that breast milk decreases the incidence of NEC and its complications. The roles for treatment with probiotics, nonspecific (steroids, indomethacin, magnesium, copper) and specific antiinflammatory agents (PAF receptor blockers), growth factors (erythropoietin), or antibiotics remain speculative (Millar et al., 2003).
Beyond the gastrointestinal outcome, the risk for long-term neurodevelopmental problems was first reported in 50% of survivors almost three decades ago (Stevenson et al., 1980). Deficits in psychomotor and mental development have been confirmed in the VLBW survivors of NEC, especially those who have undergone surgery (Vohr et al., 2000; Stoll et al., 2004; Hintz et al., 2005; Rees et al., 2007; Schulzke et al., 2007). The risk for those treated surgically is approximately twice that of those treated medically. In fact, those treated medically have outcomes similar to those of the same gestational age without a history of NEC (see Chapter 17, Neonatology for Anesthesiologists).
Imperforate Anus (Anal Atresia)
The incidence of anorectal malformations is 1:5000 live births. Miller et al. (2009) reported an association of anorectal atresia with caffeine intake, cigarette smoking, and environmental tobacco smoke. Imperforate anus can range from a mild stenosis to a complex syndrome with other associated congenital anomalies. The higher the anatomic relation of the terminal bowel to the puborectalis sling of the levator musculature, the greater is the incidence of associated anomalies. The incidence of additional genitourinary abnormalities is 48%, but it ranges from 14% in infants with perineal fistulas to 90% in infants with bladder fistulas. Twenty-four percent of infants have a tethered spinal cord (Levitt et al., 1997). Male infants with imperforate anus may require an operation soon after birth for relief of obstruction. In female infants, a rectovaginal fistula usually prevents total bowel obstruction so that surgical treatment is not an emergency.
Intraoperative Management
Anesthetic requirements vary depending on the severity of the abdominal distention and complexity of the surgery: a simple perineal anoplasty, a temporary colostomy, or an extensive abdominoperineal repair. Anorectal malformation can be classified by the presence or absence of a fistula and by the fistula’s location (Pena and Hong, 2000) (Box 18-3). Perineal fistulas in both male and female infants represent the simplest defect, and treatment generally consists of an anoplasty performed in the neonatal period. Imperforate anus with no fistula is the least common, presentation, whereas rectourethral fistulas are the most common, with the exception of the perineal fistula. The standard surgical approach has generally involved the following three steps:
Surgical trends, however, have been aimed at performing the primary repair without a colostomy. The primary repair involves a posterior surgical approach. Laparoscopic techniques have been used to assist in the pull-through technique (Georgeson, 2000).
Intubation of the trachea of infants with an apparently normal upper airway can be accomplished with an awake or rapid-sequence technique. If the anesthesiologist suspects that the upper airway will be difficult to visualize, the usual airway precautions should be observed. That is, neuromuscular blocking agents, deep sedation, and general anesthesia are avoided and an awake technique is attempted. Supplemental support systems for the difficult airway (e.g., neonatal bronchoscopes, light wand, and LMA) should be available (see Chapter 10, Equipment).
Intestinal Obstruction
Duodenal Obstruction
The incidence of duodenal obstruction in the neonate is 1:10,000 to 1:40,000 births and is often associated with other congenital anomalies such as Down syndrome, cystic fibrosis, renal anomalies, intestinal malrotation, and especially midline defects such as esophageal atresia and imperforate anus. An intraluminal diaphragm, a membranous web, or an annular pancreas can also be associated with obstruction of the duodenum. The degree of obstruction varies from severe or complete atresia to incomplete obstruction or stenosis (Mustafawi and Hassan, 2008). Air contrast films reveal a dilated stomach and a dilated proximal duodenum, resulting in the “double-bubble” appearance (Fig. 18-22).
Jejunoileal Atresia
The etiology of jejunoileal atresia is uncertain but is thought to involve intrauterine vascular accidents. Four types of atresia have been identified (Fig. 18-23). Type I is not a true atresia but actually is a membranous obstruction of the lumen in an intestine of otherwise normal length and diameter. Type II, a true atresia, consists of two blind ends that are often connected by a fibrous strand with slightly shortened intestinal length. Type IIIA lesions have blind ends separated by a mesenteric defect. The type IIIB lesion is also called “apple peel” or “Christmas tree” deformity and consists of a long jejunal atresia with a very short remaining ileum. The superior mesenteric artery is missing, and the blood supply to the ileum is by retrograde flow via a branch of the ileocolic artery. Type IIIB lesions are rare but have a very high mortality rate. Type IV lesions involve multiple intestinal atresias.
Meconium Ileus
Meconium ileus is a luminal obstruction of the distal small bowel by abnormal meconium. Meconium ileus is found almost exclusively in patients with cystic fibrosis, but only 20% of patients with cystic fibrosis have meconium ileus. Because meconium ileus manifests in the neonatal period, respiratory symptoms of cystic fibrosis generally are not present. Both surgical and nonsurgical therapies are used to relieve the obstruction. The nonsurgical approach involves diatrizoate meglumine enemas, which can be both diagnostic and therapeutic. Diatrizoate meglumine, a water-soluble contrast agent, loosens and softens the meconium, thereby facilitating its evacuation. When medical management does not succeed, surgery is performed. After the peritoneal cavity is entered and the obstruction is located, diatrizoate meglumine or acetylcysteine is injected into the bowel lumen and allowed to mix with the meconium. When the meconium has loosened, it is massaged into the colon. If this is unsuccessful, an enterostomy is performed, and the sterile meconium is evacuated from the small bowel (Fig. 18-24).
Malrotations and Volvulus
Malrotations are rare and generally result from abnormalities in the rotation of the bowel, which usually occur during the tenth to twelfth weeks of gestation. Consequently, areas of ischemia and atresia develop, along with volvulus, resulting in strangulation of bowel, bloody stools, abdominal distention, peritonitis, and hypovolemic shock (Fig. 18-25). Nonrotation or malrotation is twice as common in boys as in girls and often produces symptoms of intestinal obstruction in the first 1 to 2 months after birth. In other cases, symptoms do not appear until later, even adulthood. The diagnosis of malrotation cannot be excluded if diarrhea is present, if clinical symptoms are mild, or if x-rays are normal (Miller et al., 2003). In patients with intestinal malrotation without volvulus, surgery often involves Ladd’s procedure, which can be performed either open or laparoscopically (Draus et al., 2007). Malrotations are often associated with duodenal stenosis or atresia or small intestinal atresia, as well as with cardiac, esophageal, urinary, and anal anomalies. Major anatomic defects of the abdominal wall (e.g., gastroschisis and omphalocele) and CDH universally have intestinal malrotation or nonrotation.
Sacrococcygeal Teratoma
Embryology and Diagnosis
Sacrococcygeal tumors generally arise from the tip of the coccyx and vary with the amount of internal and external extension. These tumors are classified into four types according to their locations (Box 18-4; Fig. 18-26).
Intraoperative Management
Surgical treatment involves complete resection of both the tumor and coccyx. Failure to remove the coccyx completely can result in local recurrence. In utero treatment options include open fetal surgery, endoscopic laser ablation, and radiofrequency ablation (Bullard and Harrison, 1995; Hecher and Hackeloer, 1996; Paek et al., 2001) (see Chapter 19, Anesthesia for Fetal Surgery).
Outcome
Predictors of poor outcome have been associated with diagnosis before 20 weeks’ gestation, delivery before 30 weeks’ gestation, development of hydrops, low birth weight, and a 5-minute Apgar score of less than 7 (Chisholm et al., 1999). Long-term issues involve urologic complications, urinary incontinence, problems with defecation, and cosmetically unacceptable scars (Derikx et al., 2007; Tailor et al., 2009). Associated congenital anomalies are common in patients with congenital anorectal malformations (Stoll et al., 2007).