CHAPTER 81 ANATOMY AND PHYSIOLOGY OF MUSCLE AND NERVE
NERVE
The fundamental property of nerve that distinguishes it from other cells in the body is its ability to produce and conduct the regenerative electrical signals known as action potentials. The generation of an action potential takes place at the neuronal surface membrane; once initiated, the signal may be conducted over relatively long distances.1 This extraordinary feat is accomplished because neurons have excitable membranes that are capable of holding a charge.
The term motor unit was originally introduced by Sherrington2 as a description to include an individual nerve fiber with the bunch of muscle fibers it activates. A contemporary view would, of course, include the entire motor neuron, dendrites, and cell body, as well as its axon (Fig. 81-1). There are three types of motor neurons and two basic categories of striated muscle fibers. α motor neurons are large cells with fast-conducting axons, which innervate the large muscle fibers that make up the bulk of a muscle. These muscle fibers are called extrafusal, to differentiate them from the much smaller specialized intrafusal muscle fibers, which are present only within muscle spindle stretch receptors. Intrafusal muscle fibers are innervated by their own specialized motor neurons and axons, which are referred to as fusimotor or γ motor neurons. There is a third group of motor neurons, referred to as skeletofusimotor or β motor neurons, which innervate both extrafusal and intrafusal motor fibers. α motor neurons are among the largest neurons in the mammalian central nervous system and have remarkably extensive dendritic trees. These cells exhibit a twofold range in average soma diameters and up to a fivefold range in cell body volume and total surface area. The size of α motor neurons is correlated with the diameter of their axons and with their physiological properties. The largest of the α motor neurons are classified as type II, which in turn innervate fast-twitch glycolytic muscle fibers. The smaller of the α neurons, type I, innervate slow-twitch oxidative muscle fibers (Table 81-1). The motor neurons that innervate different muscles are grouped into longitudinal columns that lie with the ventrolateral gray matter of the spinal cord. The position of the nuclear column for a particular muscle is predictable, and the number of motor neurons within these columns is approximately the same from one individual to another. α and γ motor neurons innervating a given muscle are mixed more or less randomly within its motor nucleus. On average, between 25% and 40% of motor neurons in a given motor nucleus are γ. Large muscles generally tend to possess more motor units than do small muscles, but the range of variation is less than might be expected on the basis of relative muscle size. For example, the nerve to the bulky medial gastrocnemius muscle of a human contains about 580 motor axons, whereas the much smaller first dorsal interosseous nerve has 119 axons. The nerve to the tiny lateral rectus muscle contains more than 1700 motor axons. Thus, function as well as muscle volume determine the number of motor neurons present in motor nuclei.
In 1874, Ranvier3 recognized a correlation between muscle color and contraction speed. He found that red muscle had slow-twitch properties and pale muscle had fast-twitch properties. These designations derived from the overall characteristics of whole muscles, but within individual muscles, motor unit properties are actually quite heterogeneous. Burke4 characterized three types of motor unit twitch properties: fast-fatiguing (“FF”), fast resistance (“FR”), and slow-twitch fatigue-resistant (“S”) motor units. As noted previously, slow “S”-type muscle units tend to be innervated by relatively slow-conducting motor axons, and motor neurons tend to present greater electrical resistance to currents passed into them through a micropipet electrode. They also have relatively long action potentials after hyperpolarization, which tends to limit their firing rates to slower frequencies. Fast-twitch motor neurons have lower input resistance and shorter-duration hyperpolarization positive action potentials after activation, which are associated with higher firing rates. In both instances, the firing frequencies of the motor unit appear to be physiologically matched to the twitch properties of the innervated muscle fibers. Fast-twitch muscle units have fast-twitch times and high tetanic fusion frequencies and generate high forces, but they fatigue in ways that do not enable these forces to be maintained for long periods. Slow-twitch motor units, in comparison, are activated by motor neurons that fire at relatively lower frequencies to produce the required tetanic fusion.
MUSCLE
Skeletal muscle is commonly referred to as striated muscle because of its appearance on both light and electron microscopy. Striated muscle is the major tissue component in the body, accounting for 40% to 50% of body weight. Striated muscle is under direct voluntary control and consists of two main categories of fibers: extrafusal and intrafusal.5
Intrafusal muscle fibers are further subdivided into nuclear chain and nuclear bag fibers. These are collectively referred to as the muscle spindle. Spindles are found in all skeletal muscles except facial muscles.6 Spindles are sensory receptors that signal information concerning the degree of stretch applied to a muscle and the velocity of the applied stretch. Extrafusal muscle fibers are the major component of skeletal muscle and are the fibers responsible for the generation of force in movement. The extrafusal fibers are the fibers that are attached to tendons and bone. This section deals primarily with extrafusal muscle fibers.
Histology
Individual skeletal muscles consist of a bundle of muscle fibers within a connective tissue framework. These fibers are multinucleated, long cylindrical cells surrounded by the sarcolemma, which includes the plasma membrane and the basal lamina. Of interest is that the muscle membrane is convoluted along its length and that these folds tend to disappear when the muscle stretches.7 The plasma membrane invaginates into the substance of muscle, forming tunnels, or T (transverse) tubules. The T tubules contain extracellular fluid, forming a channel system throughout the muscle’s interior.8,9
Historically, it was thought that all muscle fibers ran in parallel along the entire length of the muscle. However, it has become increasingly clear that many muscles have a complex array of short fibers or even overlapping fibers.10 Some of these shorter fibers have been noted to be only approximately 2 cm in length.11 Although these morphological arrangements were originally believed to be specific only to long, straplike muscles, there is considerable evidence suggesting that they are common in many muscles with various architectural designs and are seen even across several species.12 On the basis of this information, muscle fibers must be defined as a functional entity rather than an anatomical one. This functional entity can consist of a number of small interdigitating fibers that are then orchestrated into action via intramuscular nerve branches.13 Furthermore, there exists evidence that these in-series short fibers may belong to different motor units.14
Striated muscle is subdivided into three compartments. First, the epimysium provides a tough collagenous elastic envelope, which defines the boundaries of the muscle from adjacent structures and at its ends merges with tendons, aponeurosis, or periosteum. The muscle is then further subdivided into small sections or fascicles by a collagen sheath termed the perimysium. Finally, the individual muscle fibers are separated one from another by the endomysium (Fig. 81-2).
Each myofibril is composed of serially repeating segments, the sarcomeres. An individual sarcomere consists of a dark central band, the A band, with two paler bands on either side. In the center of the A band there is a dark transverse line, the M band. Lying on either side of the M band is a slightly lighter segment. These segments, along with the M band, form the H zone, which lies within the A band. The A band’s major constituent is the protein myosin. The pale I bands are found on either side of the A band and are further subdivided at their midpoint by the Z band or disc. The I bands are composed by the actin filament. The actin filament is formed by three proteins: actin, tropomyosin, and troponin. The Z bands mark the longitudinal boundaries of the individual sarcomeres. Contraction of a myofibril is accomplished by shortening of the sarcomere and apposition of the Z bands to the center A band (Fig. 81-3).15
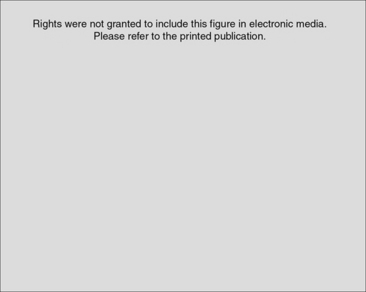
(From http://users.rcn.com/jkimball.ma.ultranet/BiologyPages/M/Muscles.html#sarcomere; accessed April 11, 2006.)
There are many other known protein structures found in muscle; for an excellent review, refer to an article by Au.16
Physiology
Type 1 muscle fibers are also referred to as red fibers,17 because of their greater content of myoglobin. Type 1 muscle fibers are also endowed with more mitochondria, higher capillary density, and greater blood flow. These fibers depend on aerobic respiration and function mainly in postural or sustained activity. Type 2 muscle fibers are white; they are rich in glycogen, have a smaller mitochondrial population and are thus more efficient under anaerobic respiration. Type 2 muscle fibers are more adept at sudden or intermittent activity (Table 81-2).18
ATPase, adenosine triphosphatase.
ATPase typing is accomplished with the histochemical reaction for myofibrillar ATPase in alkaline or acidic media. It is thus possible to differentiate muscle into type 1 (slow-twitch) and type 2 (fast-twitch), as well as into types 2a, 2b, and 2c fibers.19 At a pH of 9.4, the standard or alkaline ATPase reaction, type 1 fibers stain pale and type 2 fibers stain dark. In an acidic medium, the reverse staining pattern occurs.
The oxidative enzyme content of the myofiber reflects its dependence on the tricarboxylic acid cycle, the cytochrome system, and other metabolic pathways for aerobic metabolism. The oxidative enzyme reactions commonly used are the reduced form of nicotinamide adenine dinucleotide-tetrazolium reductase (NADH-TR) and succinic dehydrogenase. Darkly stained fibers are oxidative type 1, and less intensely stained fibers are type 2. In accordance with this enzymatic reaction, type 2 fibers can be further subdivided into type 2b (virtually unstained) and type 2a, with intermediate staining between type 1 and type 2b.
In humans, these muscle fiber types are generally arranged in a checkerboard manner, although the average muscle has about twice the number of type 2 fibers as type 1 fibers. The arrangement of different fiber types in fascicles is determined by the function of the particular muscle. In the vastus lateralis muscle of young healthy men, it was found that the proportion of type 2 fibers was consistently greater at the periphery than internally.20
Common Reaction of Muscle to Injury
Histochemical preparations are used to evaluate the health of muscle. Muscle atrophy or hypertrophy can readily be seen with ATPase staining. Type 2 muscle fiber atrophy, the most common type of selective fiber atrophy, occurs with early stages of denervation, with disuse, and as a paraneoplastic complication of systemic malignancy.21 Type 1 fiber atrophy can also occur in some of the congenital myopathies and myotonic dystrophy.22 The most common cause of selective type 2 fiber atrophy is long-term corticosteroid therapy. Selective type 1 fiber hypertrophy is highly suggestive of Werdnig-Hoffman disease.
Effects of Aging on Muscle
Aging has been associated with a loss of muscle mass that is referred to as sarcopenia.23 This decrease of muscle mass becomes more pronounced after the sixth decade of life. Several changes take place in muscle during senescence: there is loss of power, strength, and endurance. This decrease in whole muscle size is mirrored by a loss of fiber number.24 The loss of muscle fibers is multifactorial; loss of motor units lead to denervation, a decrease in circulating trophic factors, and an increase in catabolic agents.
Au Y. The muscle ultrastructure: a structural perspective of the sarcomere. Cell Mol Life Sci. 2004;61:3016-3033.
Monti RJ, Roy RR, Edgerton VR. Role of motor unit structure in defining function. Muscle Nerve. 2001;24:848-866.
Sumner AJ. The Physiology of Peripheral Nerve Disease. Philadelphia: WB Saunders, 1980.
1 Sumner AJ, editor. The Physiology of Peripheral Nerve Disease. Philadelphia: WB Saunders, 1980.
2 Sherrington CS. Ferrier Lecture—some functional problems attaching to convergence. Proc R Soc Lond (Biol). 1929;105:332-362.
3 Ranvier L. De quelques faits relatifs à l’ histologie et à la physiologie des muscles striés. Arch Physiol Norm Pathol. 1874;1:5-18.
4 Burke RE. On the central nervous system control of fast and slow twitch motor units. Desmedt JE, editor. New Developments in Electromyography and Clinical Neurophysiology. vol 1. Basel, Switzerland: Karger; 1973:69-94.
5 Guyton AC. Textbook of Medical Physiology, 8th ed., Philadelphia: WB Saunders; 1991:38-50.
6 Cooper S. Muscle spindles and other muscle receptors. In: Bourne GH, editor. The structure and function of muscle. New York: Academic Press; 1960:381.
7 Dumitru D, Amato AA, Zwarts MJ. Electrodiagnostic Medicine, 2nd ed., Philadelphia: Hanley & Belfus; 2002:12.
8 Fawcett DW. Bloom and Fawcett: A Textbook of Histology. Philadelphia: WB Saunders, 1986.
9 McComas AJ. Neuromuscular Function and Disorders. Boston: Butterworth, 1977.
10 Huber GC. On the form and arrangement of fasciculi of striated voluntary muscle fibers. Anat Rec. 1917;11:149-168.
11 Trotter JA, Richmond FJR, Purslow PP. Functional morphology and motor control of series-fibered muscles. Exerc Sport Sci Rev. 1995;23:167-213.
12 Monti RJ, Roy RR, Ederton VE. Role of motor unit structure in defining function. Muscle Nerve. 2001;24:848-866.
13 Loeb GE, Pratt CA, Chanaud CM, et al. Distribution and innervation of short, interdigitated muscle fibers in parallel-fibered muscles of cat hindlimb. J Morphol. 1987;191:1-15.
14 Pratt CA, Loeb GE. Functionally complex muscles of the cat hindlimb. I. Patterns of activation across sartorius. Exp Brain Res. 1991;85:243-256.
15 Mastaglia FL, Walton J. Skeletal Muscle Pathology. Edinburgh: Churchill Livingstone, 1982;12-21.
16 Au Y. The muscle ultrastructure: a structural perspective of the sarcomere. Cell Mol Life Sci. 2004;61:3016-3033.
17 Ashhurst DE. The fine structure of pigeon breast tissue. Tissue Cell. 1969;1:485.
18 Nelson JS, Parisi JE, Schochet SS. Principles and Practice of Neuropathology. St. Louis: CV Mosby, 1993.
19 Brooke MH, Kaiser KK. Muscle fibre types: how many and what kind? Arch Neurol. 1970;23:369-379.
20 Lexell J, Downham D, Sjostrom M. Distribution of different fibre types in human skeletal muscles. A statistical and computational study of the fibre type arrangement in m. vastus lateralis of young healthy male. J Neurol Sci. 1984;65:353-365.
21 Barron SA, Heffner RR. Weakness in malignancy: evidence for a remote effect on distal axons. Ann Neurol. 1978;4:268-274.
22 Bethlem J. Myopathies, 2nd ed. New York: Elsevier, 1980.
23 Deschenes MR. Effects of aging on muscle fibre type and size. Sports Med. 2004;34:809-824.
24 Lexell J, Henriksson-Larsen K, Winblad B, et al. Distribution of different fibre types in human skeletal muscles: effects of aging studied in whole muscle cross sections. Muscle Nerve. 1983;6:588-595.