Chapter 97 Training for Wilderness Adventure
For online-only figures, please go to www.expertconsult.com
For a couple million years, humans have survived in wilderness environments. It is only in the last few millennia that socialization has led to what we call civilization. Modern life has formed a construct for human existence that has largely overcome the need for survival competence. However, there are still populations that rely on physical capabilities and resourcefulness to survive on planet Earth, most of which is still wilderness (Figure 97-1).
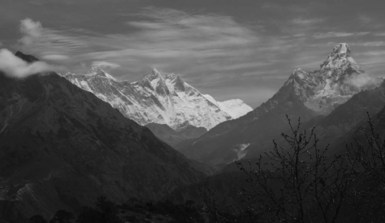
FIGURE 97-1 View in the Khumbu region of Nepal, with Mt Everest, Mt Lhotse, and Mt Ama Dablam appearing most prominent.
(Courtesy Robert B. Schoene.)
Physical Conditioning
Speed training is essential. Strength and flexibility training are covered in Chapter 98. This chapter deals primarily with aerobic fitness and exercise physiology with an emphasis on high-altitude fitness, because adaptation and exercise performance in that environment carry with them concepts universally applicable to all wilderness endeavors.
Aerobic Fitness
The concepts of aerobic fitness are similar for champion and recreational athletes. The parts of the “engine” are the same; it is the quality and fine-tuning that are different. Three essential characteristics are maximum oxygen consumption (max), lactate or anaerobic threshold, and efficiency. These factors are interrelated in a way that results in effective performance, and each is trainable. The interrelationships result in improved endurance, the most important overall factor for enjoyment and survival in the wilderness.34
Maximum Oxygen Consumption
Oxygen consumption () is defined by the Fick equation:
Cardiac output is equal to heart rate multiplied by stroke volume. Oxygen extraction is the difference between the content of oxygen of the arterial and mixed venous blood (i.e., the amount of oxygen that is used as blood traverses tissue beds). The metabolic response of exertion is limited by cardiac output and the limits of oxygen extraction, both of which can be modulated with training. The role of max and its various considerations are discussed by Levine.40
max is the fingerprint of an individual’s physiology. It is a reproducible marker of fitness in an individual that varies depending on training, altitude, and illness. The many genetic factors (i.e., polygenic) that contribute to a person’s
max make it highly unlikely that any one individual could be endowed with all of these genes.60 One’s
max is influenced by both inherited and environmental factors.8 What remains to be explained is the observation that, among sedentary subjects in family groups who were maintained on a supervised aerobic exercise program for 20 weeks, there was great variability in how much
max could be improved7 (Figure 97-2). The improvement in
max ranged from negative values to 30% improvement, and these various levels of improvement were grouped in family clusters. Further data from this series of studies looked at age, race, gender, and initial fitness and found that all subjects experienced gains in
max, but with a great deal of variability and little correlation among the aforementioned factors that contributed to those gains. It is clear that there are limits in training to improve
max. In other words, a “normal” individual with a
max of 42 cc/kg/min may be able to improve his or her
max to the high 40s cc/kg/min but will never be able to approach the 75 to 85 cc/kg/min range of high-performance middle- to long-distance athletes, who chose their parents well.
What parts of one’s aerobic capacity can be trained? Considering the Fick equation, it becomes apparent that an increase in cardiac output, improved extraction of oxygen, or both will improve max. In fact, both things happen, but it is clearly the heart that can be trained more by increasing its stroke volume and improving its muscular strength.20,25 Thus, the heart rate necessary to achieve an appropriate cardiac output for any given metabolic rate is lower in the trained state as compared with the untrained state. Although maximum heart rate does not change with training, resting and submaximal heart rates are lower and can be used as simple markers to monitor training. Although the elements of oxygen extraction somewhat improve, the heart’s stroke volume conveys increased ability to perfuse large volumes of muscle mass such that, with training, there are increased capillary and mitochondrial densities and optimization of the components of oxidative metabolism.3,24,29,30
It is fascinating to put human physiology in perspective with the rest of the animal kingdom. Normal humans in the age range of 20 to 40 years have a max somewhere around 40 cc/kg/min, and accomplished endurance athletes have one in the range of 70 to 85 cc/kg/min; alternatively, some large mammals have extraordinarily high aerobic capacities. For instance, horses have
max values that range from 134 cc/kg/min in standardbred horses2 to 160 cc/kg/min in thoroughbreds.38,43 The North American pronghorn antelope is said to have values as high as 300 cc/kg/min. Although thoroughbred horses were bred several hundred years ago to be great aerobic athletes, the antelope’s evolutionary strategy is to have exercise capabilities that optimize its chance of preserving the small family groups that live on an open plain full of predators (i.e., the pronghorn can run sustainably at 80.5 km/hr [50 mi/hr]).
Sustainable Threshold
The onset of unsustainable work intensity essentially involves a shift of fuel supply within the cell. At workloads below the LT, free fatty acids are the primary oxidative fuel. Above the LT, when the oxidative turnover of free fatty acids cannot keep up with the demand for adenosine triphosphate, glycolysis occurs. Muscle glycogen is broken down as fuel, with lactic acid being produced at a rate beyond the body’s ability to use it.33,49 Blood lactate levels correlate with intensity of work and thus are inversely correlated with the duration of a competitive event (Figure 97-3).
Improving Human Performance
Malleability of the Lactate Threshold
The ability of a muscle to sustain work is related to its oxidative capacity. This capacity is quite malleable, and depends on the level of the muscle’s activity while it is engaged.19,28 Among high-level athletes, oxidative capacity can be several-fold greater than among untrained individuals. Functionally, then, high-level athletes have inherently high max levels, and can perform sustainable work at a much higher percentage of their maximum capacity. For example, an international cyclist may have a maximum work capacity of 550 watts and be able to sustain 450 watts of work during an hour-long hill climb. This is an extraordinary level of work output. A more usual and quasi-sedentary individual may have a maximum workload of 200 watts and be able to sustain 50% to 60% of that work intensity, which is considered to be “normal.”
In highly specialized athletes such as cyclists, the muscle mass involved in the effort has been shown to be progressively recruited in a way such that the oxidative stress is balanced and shared.13,15 As much as 25% of the cyclist’s muscle mass can be spared on a rotating basis, which reduces the oxidative stress of muscle fibers, thus prolonging the onset of the LT. This phenomenon may perhaps be a way to acquire more endurance, delay fatigue, and promote efficiency. Furthermore, with a finite fuel supply, this strategy would preserve glycogen stores and delay the onset of glycolysis (and thus the production of lactate).
The crux of cellular oxidative metabolism is convection of oxygen to the cell by the circulation, diffusion of oxygen across the cell membrane into the cytosol, and then diffusion of oxygen into the mitochondria. The actual diffusion gradient necessary to get oxygen to the mitochondria is on the order of 2 to 3 mm Hg at each of these steps.52 Thus, perfusion rather than hypoxemia per se is a limiting factor. Therefore, one of the most important adaptive steps is augmenting blood flow through angiogenesis of the microcirculation. In this regard, in two studies, highly trained cyclists and triathletes with comparable values of max were exercised at 88% of their maximum aerobic capacity until fatigue.13,14 There were two patterns that showed a shorter and longer time to fatigue. The athletes with more endurance had a substantially greater capillary density than did the athletes who fatigued earlier, despite comparable maximum aerobic capacities. Because both groups were highly trained, it is not clear whether, in certain athletes, there is some inherent propensity for greater signaling of angiogenesis that comes from training. The authors speculated that this augmented perfusion may be important not only for the convective phase of oxygen but also for providing a greater volume of the effluent portion of metabolic byproducts. Another study looked at subtle factors that contribute to fatigue at very high levels of exercise and found that very small changes in energy expenditure when a person is at exercise intensities of greater than 80% of
max can lead to rapid onset of fatigue.44 Therefore, it is critical for an athlete—whether on the field or in the wilderness—to know the location of his or her “edge” so there is some reserve for optimally finishing an activity.
Training Effect on the Lactate Threshold
Much has been written since the late 1970s about plasticity of the LT. It behooves any athlete to be able to perform at the highest percentage of his or her maximum aerobic capacity. One of the first studies to look at the effect of aerobic training on the LT involved nine sedentary men who performed 9 weeks of supervised endurance training for 45 minutes per day for 4.1 days per week.16 There was a comparable untrained control group. The exercise group increased its LT by 44% expressed as absolute , and 15% expressed as
max.
max also increased 25%. Maximum work rate increased 28%, with decreases in the ventilatory equivalent seen at submaximal levels of work. The volume of work was similar in the test group, so the study did not answer the following questions (Figure 97-4): How much volume is necessary to induce these changes? If some work is good, is more or less better?
Several studies have looked at a number of variations on the IT theme and its effect on performance, LT, serum lactate, time to exhaustion, muscle physiology, and so forth. One study enrolled seven trained male distance runners and added 3 days of intense levels of training (i.e., >95% heart rate maximum) per week for 8 weeks.1 The results showed no change in max, but there was improvement in 10,000-m times, increased time to exhaustion on a set treadmill pace and incline, decreased serum lactate concentrations at 85% and 90% maximum heart rate, and correlations of the decrease in lactate with improvements in performance times. In another study among recreationally active young males, a mere six bouts of four to seven “all-out” Wingate tests spread out over 2 weeks (with recovery days in between) resulted in a 100% increase in cycle endurance time, with muscle biopsies showing a 26% increase in muscle glycogen and a 38% increase in citrate synthase, both markers of muscle oxidative capacity10 (Figures 97-5 and 97-6).
Another study divided 16 active young males into intense IT and endurance training groups that were followed for 2 weeks. The time commitment for the two groups was 2.5 hours and 10.5 hours, respectively. Training results for both groups were similar in that they showed improved time trial times and similar changes in markers of oxidative capacity and buffering capacity in muscle biopsy samples after the training intervention23 (Figure 97-7).
In a study of a somewhat similar design and intent with more outcome variables, this same investigative group showed comparable improvements in performance, endurance time, and oxidative markers in muscle biopsies9 (Figure 97-8). After 4 weeks of speed IT in a group of healthy recreationally active subjects, similar findings resulted when compared with those of an endurance group, and the muscle-capillary–to–fiber ratio was similar in both groups.31
Thus, training intensity was heralded as an important part of overall training.13 From a practical standpoint, for busy lives, efficiency of training may be an important consideration; thus, this is a significant finding.
Efficiency of Movement
A fascinating example of efficient energy expenditure comes from a study at 4700 m in Tibet, where the authors did maximal exercise testing for 17 Tibetans native to the area and 14 recently migrated Han Chinese. Although the Han had higher max values than did the Tibetans (i.e., 36 versus 30 cc/kg/min), the Tibetans generated significantly higher work output (i.e., 176 versus 150 watts) at those maximum
levels, LTs at higher percentage of
max (i.e., 84% versus 62%), and lower blood lactate concentrations.22 There is ongoing speculation as to whether these characteristics are genetic or adaptive; however, regardless of the mechanism of these differences, the Tibetans appear to be ideal work machines at high altitude, and they are able to perform more efficient work with less energy expenditure. Thus, the question arises: Can we train ourselves not only to be more fit but also more efficient (i.e., the perfect adaptation for the wilderness adventurer)?
There are a number of studies demonstrating that—with aerobic, anaerobic, and resistive training—modest improvements in work efficiency can be attained. In a previously cited study,31 speed endurance training in competitive runners resulted in 5.7% to 6.6% lower oxygen consumptions at three set levels of speed on a treadmill compared with endurance-trained runners. In another training study in runners comparing endurance and interval training for 4 weeks,6 many of the measured variables, including max, were unchanged. The most notable finding was that, at maximum energy expenditure (which was unchanged between the two groups), velocity was significantly higher in the runners who had used IT, suggesting greater running economy. Although the topic of exposure to intermittent hypoxia will be discussed more later in this chapter, it is worthy of brief mention to note that, in one study, college track athletes were randomly assigned to 29 days of low-altitude normoxia, constant simulated high altitude (3000 m), or nocturnal hypoxia. Although there were no changes in
max, hemoglobin, endurance, or LT, the athletes exposed to intermittent nocturnal hypoxia showed about 5% improvement in running economy at a high race speed. The mechanisms for these improvements are only speculative, but some insight may be gained from a study looking at work efficiency in highly trained cyclists. The investigators looked at work related to caloric expenditure and found a positive correlation with type I (aerobic) muscle fiber types taken from thigh muscle biopsies.15 Of note was the large range (i.e., 32% to 76%) of type I fibers in these athletes. One of the potential mechanisms of energy cost saving with intense training of any type may be a decrease in ventilatory demand and thus the work of breathing, which, although modest, may be critical to performance.21 The addition of explosive strength to normal endurance training in competitive runners as compared with ongoing endurance training resulted in improved 5000-m times, which correlated with the improvement in running efficiency. It has even been suggested that ongoing training and competition by a repeat Tour de France winner have resulted in improved efficiency.13 Although the improvements in efficiency are modest in most of these studies, such improvements may be critical not only to competitive athletes but also to survival in a wilderness environment.
Aging and Training
As one ages, wisdom—more than speed—in the wilderness may be one’s saving grace, but it does not preclude ongoing commitment to conditioning. There is a wealth of literature about the effects of aging on muscle and aerobic performance; a brief summary is important to include here, because so many of us will continue to venture into the wilderness. It is difficult to define old age, but one could say that it begins with the later part of the sixth decade, when there seems to be an inexorable decrease in aerobic capacity and strength. There is another phase of aging, called senescence, when there is a profound and irretrievable decline in mitochondrial function, muscle mass, and cardiac compliance. The topic of master athletes and the underlying physiologic mechanisms accounting for the decline in aerobic capacity with age despite rigorous training are also important areas for aging wilderness adventurers to consider.56
The heart also loses elasticity as the ventriclas stiffen and impair end-diastolic filling. In addition, there is a reduction of maximum heart rate. This reduction can be minimized if one continues to train into older age. The oft-quoted maximum heart rate of 220 beats/min minus age is but a gross approximation taken from the cross-sectional data of mostly unfit individuals. There is a decline in peripheral vascular compliance, which may play a role in further hindering blood flow and oxygen delivery.48 Some of the earlier data also suggested a linear decline in max beginning sometime during the third decade of life,17,32 but subsequent studies have shown that little reduction in
max occurs from the age of 20 years to the mid 50s if athletes continue active training47,57 (Figure 97-9).
The cross-sectional data certainly reflect the changes in lifestyle that come with families, jobs, and geography. Although max declines in everyone at a certain point, the decline is probably a result of decreases in oxygen delivery and cardiac output as well as a decrease in oxygen use with declining mitochondrial function, especially among the truly elder.5,39,54
It also used to be thought that, after a certain age, neither strength nor aerobic capacity can be improved.4,18 However, many studies over the last two decades have clearly shown that older individuals who are otherwise healthy can train and improve both strength and aerobic capacity. The earlier studies were of rather low intensity and duration, whereas later studies showed that substantial gains could be made with higher intensity and longer duration of training. These gains can translate into practical function in any wilderness environment.
Comparing the decline in max between masters and sedentary subjects more than 60 years old, Rogers and colleagues51 showed that, with ongoing aerobic training, older individuals could decrease the decline per decade in
max from 10% to 5%. Kohrt and colleagues37 took 110 untrained men and women between the ages of 60 and 71 years, measured their prestudy
max, and then had them perform 9 to 12 months of walking or running 4 days per week for 40 minutes per session at 80% of their predicted maximum heart rates. Men and women improved their maximum values by 26% and 23%, respectively; when they were divided into three equal age groups, there also was similar improvement. In terms of improvements, these values are comparable with those seen among younger subjects. Conley and colleagues12 engaged sedentary healthy men and women between the ages of 65 and 92 years in 4 months of both aerobic and strength training and used
max testing, Cybex strength measurements, muscle biopsies, and nuclear magnetic resonance spectroscopy to measure creatine phosphate dynamics were determined before and after training. They compared the results of these subjects with those of healthy controls, and found substantial improvements in all of the outcome variables, which were equal in percentage improvement to those obtained with younger subjects.
High Altitude and Exercise
During the summer of 1968 in Mexico City, three world records were set in track and field, and one favored American miler was soundly defeated. Tommie Smith (200 m), Lee Evans (400 m), and Bob Beamon (long jump) set records that stood for decades. Jim Ryun, the favorite in the 1500 m, was beaten by Kip Keino from Kenya. Aside from the fact that the first three were serendipitously some of the greatest sprinters and jumpers of all time, part of their advantage may have been the slightly thinner air at the altitude of 2200 m (Figure 97-10).
Effect of High Altitude on Exercise
The next two sections of this chapter cover the effects of altitude on exercise as well as the positive and negative aspects of training at high altitude (Figure 97-11). Both topics have important implications for wilderness travel, much of which occurs in the cold, thin air. Chapter 1 covers the broad topics of high-altitude acclimatization and illness.
Anyone who has sojourned quickly to 3000 m or higher cannot exert themselves without dyspnea and exercise limitation. With acclimatization over time, the limitations discovered at 3000 m can be diminished or eliminated.27 At altitudes of 3500 to 8000 m, sea-level performance can not be attained despite prolonged adaptation. Although the fraction of oxygen in the earth’s atmosphere is constant at 0.2093, the barometric pressure and thus the content of oxygen decreases as one ascends. For example, with some variation depending on the weather, the barometric pressure at sea level is approximately 760 mm Hg, whereas, at the summit of Mt Everest (as measured by Chris Pizzo on October 23, 1981), the barometric pressure is 253 mm Hg. Thus, at the summit, there is approximately one-third of the amount of oxygen available for aerobic activity as compared with sea level.59 With less oxygen available in the air, there is a decrease in oxygen availability at each step of the delivery of oxygen from air to the lungs to the blood to the tissues and mitochondria. As a climber ascends, his or her max decreases55,58(Figure 97-12), and the speed of ascent decreases.
One of the limiting factors is the increased work of breathing that occurs for any given level of energy expenditure. For example, the ventilatory equivalent is almost four times greater at 6300 m than it is at sea level.11,53 Thus, blood flow necessary to perfuse the muscles of respiration is stolen from the muscles of locomotion. Furthermore, because of a diffusion limitation of oxygen from air to the blood at high altitude, the higher one ascends, the greater the amount of oxygen desaturation with exercise (Figure 97-13).
In terms of training for high-altitude ventures, other than being generally fit, there is not much a lowlander can do to prepare. Chapter 1 suggests rates of ascent to minimize the chances of getting altitude illnesses. These rates are similar to those needed for people to gain acclimatization and fitness. The most common mistake is to take too little time for a trip to the Himalayas or the Andes—or even a weeklong ski trip to Colorado. A rigid schedule that does not allow sufficient time for acclimatization is dissatisfying at best and dangerous at worst. Having been pre-exposed to high altitude before going on a challenging trek is always beneficial, but usually not feasible or practical.46 Such issues have become important considerations for military personnel, who may need to ascend rapidly for certain operations.45
Hypoxic Training
When it finally dawned on trainers and coaches that full-time hypoxic exposure might not be beneficial, a number of methods were tried to get “enough” hypoxic exposure without having too much. Levine and Stray-Gundersen41 set out on a prolonged series of experiments in accomplished runners. The study design included four groups: (1) athletes who lived and trained at low altitude (LLTL); (2) those who lived high and trained high (LHTH); (3) those who lived low and trained high (LLTH); and (4) those who lived high and trained low (LHTL). Part of the rationale for such a design was to test the hypothesis that training at high altitude does not allow an athlete to achieve as intense a training effect as he or she could achieve at lower altitude. The altitude exposure of the LHTL group was 4 weeks of 20 hours per day at 2500 m. The upshot from these series of studies was that the athletes who improved their max and 5000-m times were in the LHTL group, and this improvement correlated with those who had an erythropoietic response: the increase in
max corresponded precisely with the increase in oxygen-carrying capacity. However, even in the LHTL group, there were responders and nonresponders, which were differentiated by improvements in 5000-m race time. The responders’ improvements corresponded with whether they had an erythropoietic response. Levine contends that there is a threshold (i.e., specific hypoxic dose) that is necessary to inducing these responses and that, in a number of previous studies, the dose may not have been long or high enough.42 In his discussion, he reminds the reader that the mechanism of response to hypoxia is signaled by hypoxia-inducible factor-1α and that this protein is one of the most evanescent proteins of gene transcription described in the body: when the hypoxic stimulus is removed, hypoxia-inducible factor-1α disappears within milliseconds. Thus, all subsequent gene transcription ceases, and no further growth factors (e.g., erythropoietin) are stimulated. The hypoxic exposure must be intense and sufficiently prolonged to keep the cycle going. Not everyone responds, and the gains are minimal among those who do. So is it all worth it?
Many athletes think it is worth it. The urge to excel has led to “hypoxic sleeping tents” that can be purchased. With such a tent, the athlete can sleep in his or her bed and dial in progressive altitudes for weeks of “natural acclimatization and blood doping.” This trend has led to a number of studies of intermittent hypoxia (i.e., normobaric or hypobaric) to see if effects similar to those obtained at true altitude could be found. Julian and colleagues35 exposed athletes to progressive normobaric hypoxia (i.e., a fraction of inspired oxygen of 0.11%) for 2 weeks for 70 minutes each day and found no changes in performance or erythropoietic markers. Using normobaric hypoxia (i.e., a fraction of inspired oxygen of 14.5%) for 6 weeks, Zoll and colleagues61 divided runners into two groups: LLTL and LLTH. They looked at performance (i.e., max and time to exhaustion) and muscle biopsies to evaluate markers of hypoxic transcription and oxidative phosphorylation. With the LLTH design, the researchers found a modest improvement in
max (5%), a remarkable improvement in time to exhaustion (26%), and substantial increases in the transcription factors and markers of oxidative phosphorylation. Gore and colleagues26 exposed athletes to a simulated altitude of 4000 to 5500 m for 3 hours per day on 5 days per week for 4 weeks and found an increase in erythropoietin but no increase in markers of red-cell production. With a similar design, this same research group studied swimmers and found no improvement in swimming times with the intermittent hypoxia.50 They concluded that the “hypoxic dose” was not adequate enough.
Not all intermittent hypoxic studies have been negative. Katayama and colleagues36 exposed a small group of runners to a simulated altitude of 4500 m for 90 minutes per day three times per week for 3 weeks. In the hypoxic group, they found improvements in running time and time to exhaustion as well as lower levels at submaximal exercise levels, with no other changes in hemodynamic or hematologic variables. Thus, the authors contended that this type of exposure led to an improvement in running efficiency. However, they did not offer give any insight into the mechanism of improvement.
Artificial Training Methods: Blood Transfusion
There is a surfeit of information on blood transfusions (“doping”) and erythropoietic medications.19a,23a,49a The interest in enhancement of performance by increasing oxygen-carrying capacity with extra red blood cells was brought to attention via the 1968 Mexico City Olympics and then into the 1970s, when certain middle-distance Scandinavian track athletes were suspected of blood doping. Subsequent data supported the benefit of increased red blood cell mass and improved aerobic performance.8a Such augmentation was achieved by either autologous or allogeneic transfusions, and later when recombinant erythropoietin (EPO) was developed. Administration of this drug was rampant in a number of sports venues, most notoriously European cycling. Although the benefit of increased red cell mass was acknowledged, the philosophy that, “If some is good, more is better” led to fatal complications in a number of young athletes, because the downside sequelae of increased red blood cell mass were increased blood viscosity, strokes, and death.
1 Acevedo EO, Goldfarb AH. Increased training intensity effects on plasma lactate, ventilatory threshold, and endurance. Med Sci Sports Exerc. 1989;21:563.
2 Armstrong RB, Essén-Gustavsson B, Hoppeler H, et al. O2 delivery at max and oxidative capacity in muscles of standardbred horses. J Appl Physiol. 1992;73:2274.
3 Befroy DE, Petersen KF, Dufour S, et al. Increased substrate oxidation and mitochondrial uncoupling in skeletal muscle of endurance-trained individuals. Proc Natl Acad Sci U S A. 2008;105:16701.
4 Benestad AM. Trainability of old men. Acta Med Scand. 1965;178:321.
5 Betik AC, Hepple RT. Determinants of max decline with aging: An integrated perspective. Appl Physiol Nutr Metab. 2008;33:130.
6 Billat VL, Flechet B, Petit B, et al. Interval training at : Effects on aerobic performance and overtraining markers. Med Sci Sports Exerc. 1999;31:156.
7 Bouchard C, An P, Rice T, et al. Familial aggregation of response to exercise training: Results from the HERITAGE Family Study. J Appl Physiol. 1999;87:1003.
8 Bouchard C, Daw EW, Rice T, et al. Familial resemblance for max in the sedentary state: The HERITAGE family study. Med Sci Sports Exerc. 1998;30:252.
8a Brien AJ, Simon TL. The effects of red blood cell infusion on 10 km race time. JAMA. 1987;257:2761.
9 Burgomaster KA, Howarth KR, Phillips SM, et al. Similar metabolic adaptations during exercise after low volume sprint interval and traditional endurance training in humans. J Physiol. 2008;586:151.
10 Burgomaster KA, Hughes SC, Heigenhauser GJ, et al. Six sessions of sprint interval training increases muscle oxidative potential and cycle endurance capacity in humans. J Appl Physiol. 2005;98:1985.
11 Cibella F, Cuttitta G, Romano S, et al. Respiratory energetics during exercise at high altitude. J Appl Physiol. 1999;86:1785.
12 Conley KE, Esselman PC, Jubrias SA, et al. Ageing, muscle properties and maximal O(2) uptake rate in humans. J Physiol. 2000;526:211.
13 Coyle EF. Improved muscular efficiency displayed as Tour de France champion matures. J Appl Physiol. 2005;98:2191.
14 Coyle EF, Coggan AR, Hopper MK, et al. Determinants of endurance in well-trained cyclists. J Appl Physiol. 1988;64:2622.
15 Coyle EF, Sidossis LS, Horowitz JF, et al. Cycling efficiency is related to the percentage of type I muscle fibers. Med Sci Sports Exerc. 1992;24:782.
16 Davis HA, Gass GC. Blood lactate concentrations during incremental work before and after maximum exercise. Br J Sports Med. 1979;13:165.
17 Dehn MM, Bruce RA. Longitudinal variations in maximal oxygen intake with age and activity. J Appl Physiol. 1972;33:805.
18 Devries H. Physiological effects of an exercise training regimen upon men aged 52-88. J Gerontol. 1970;25:325.
19 Dudley GA, Abraham WM, Terjung RL. Influence of exercise intensity and duration on biochemical adaptations in skeletal muscle. J Appl Physiol. 1982;53:844.
19a Eichner ER. Blood doping: Infusions, erythrpoeitin, and artificial blood. Sports Medicine. 2007;37:389.
20 Ekblom B, Hermansen L. Cardiac output in athletes. J Appl Physiol. 1968;25:619.
21 Franch J, Madsen K, Djurhuus MS, et al. Improved running economy following intensified training correlates with reduced ventilatory demands. Med Sci Sports Exerc. 1998;30:1250.
22 Ge FL, Chen QH, Wang LH, et al. Higher exercise performance and lower max in Tibetan than Han residents at 4,700 m altitude. J Appl Physiol. 1994;77:684.
23 Gibala MJ, Little JP, van Essen M, et al. Short-term sprint interval versus traditional endurance training: Similar initial adaptations in human skeletal muscle and exercise performance. J Physiol. 2006;575:901.
23a Giraud S, Sottas P, Robinson N, et al. Blood transfusions in sports. Handbook of Experimental Phramacology. 2010;195:295.
24 Gollnick PD, Armstrong RB, Saltin B, et al. Effect of training on enzyme activity and fiber composition of human skeletal muscle. J Appl Physiol. 1973;34:107.
25 González-Alonso J, Calbet JA. Reductions in systemic and skeletal muscle blood flow and oxygen delivery limit maximal aerobic capacity in humans. Circulation. 2003;107:824.
26 Gore CJ, Rodríguez FA, Truijens MJ, et al. Increased serum erythropoietin but not red cell production after 4 wk of intermittent hypobaric hypoxia (4,000-5,500 m). J Appl Physiol. 2006;101:1386.
27 Grover RF, Reeves JT, Grover EB, et al. Muscular exercise in young men native to 3,100 m altitude. J Appl Physiol. 1967;22:555.
28 Holloszy JO. Muscle metabolism during exercise. Arch Phys Med Rehabil. 1982;63:231.
29 Holloszy JO, Coyle EF. Adaptations of skeletal muscle to endurance exercise and their metabolic consequences. J Appl Physiol. 1984;56:831.
30 Howald H, Hoppeler H, Claassen H, et al. Influences of endurance training on the ultrastructural composition of the different muscle fiber types in humans. Pflugers Arch. 1985;403:369.
31 Iaia FM, Hellsten Y, Nielsen JJ, et al. Four weeks of speed endurance training reduces energy expenditure during exercise and maintains muscle oxidative capacity despite a reduction in training volume. J Appl Physiol. 2009;106:73.
32 Inbar O, Oren A, Scheinowitz M, et al. Normal cardiopulmonary responses during incremental exercise in 20- to 70-yr-old men. Med Sci Sports Exerc. 1994;26:538.
33 Jones AM, Wilkerson DP, Berger NJ, et al. Influence of endurance training on muscle [Pcr] kinetics during high-intensity exercise. Am J Physiol Regul Integr Comp Physiol. 2007;293:R392.
34 Joyner MJ, Coyle EF. Endurance exercise performance: The physiology of champions. J Physiol. 2008;586:35.
35 Julian CG, Gore CJ, Wilber RL, et al. Intermittent normobaric hypoxia does not alter performance or erythropoietic markers in highly trained distance runners. J Appl Physiol. 2004;96:1800.
36 Katayama K, Matsuo H, Ishida K, Mori S, et al. Intermittent hypoxia improves endurance performance and submaximal exercise efficiency. High Alt Med Biol. 2003;4:291.
37 Kohrt WM, Malley MT, Coggan AR, et al. Effects of gender, age, and fitness level on response of max to training in 60-71 yr olds. J Appl Physiol. 1991;71:2004.
38 Langsetmo I, Weigle GE, Feddle MR, et al. kinetics in the horse during moderate and heavy exercise. J Appl Physiol. 1997;83:1235.
39 Lenaz G, Bovina C, Castelluccio C, et al. Mitochondrial complex I defects in aging. Mol Cell Biochem. 1997;174:329.
40 Levine BD. max: What do we know and what do we still need to know? J Physiol. 2008;586:25.
41 Levine BD, Stray-Gundersen J. “Living high-training low”: Effect of moderate-altitude acclimatization with low-altitude training on performance. J Appl Physiol. 1997;83:102.
42 Levine BD, Eichner ER. Sports Medicine. 2007;37:389.
43 McDonough P, Kindig CA, Erickson HH, et al. Mechanistic basis for the gas exchange threshold in thoroughbred horses. J Appl Physiol. 2002;92:1499.
44 Mortensen SP, Dawson EA, Yoshiga CC, et al. Limitations to systemic and locomotor limb muscle oxygen delivery and uptake during maximal exercise in humans. J Physiol. 2005;566:273.
45 Muza SR. Military applications of hypoxic training for high-altitude operations. Med Sci Sports Exerc. 2007;39:1625.
46 Muza SR, Beidleman BA, Fulco CS. Altitude preexposure recommendations for inducing acclimatization. High Alt Med Biol. 2010;11:87.
47 Pollock ML, Mengelkoch LJ, Graves JE, et al. Twenty-year follow-up of aerobic power and body composition of older track athletes. J Appl Physiol. 1997;82:1508.
48 Proctor DN, Shen PH, Dietz NM, et al. Reduced leg blood flow during dynamic exercise in older endurance-trained men. J Appl Physiol. 1998;85:68.
48a Pugh LG. Cardiac output in muscular exercise at 5800 m (19,000 feet). J Appl Physiol. 1964;19:441.
49 Robergs RA, Ghiasvand F, Parker D. Biochemistry of exercise-induced metabolic acidosis. Am J Physiol Regul Integr Comp Physiol. 2004;287:R502.
49a Robinson N, Giraud S, Saudan C, et al. Erythropoeitin and blood doping. Br J Sports Med. 2006;40:i30.
50 Rodríquez FA, Truijens MJ, Townsend NE, et al. Performance of runners and swimmers after four weeks of intermittent hypobaric hypoxic exposure plus sea level training. J Appl Physiol. 2007;103:1523.
51 Rogers MA, Hagberg JM, Martin WH3rd, et al. Decline in max with aging in master athletes and sedentary men. J Appl Physiol. 1990;68:2195.
52 Schoene RB. Gas exchange in the lung and muscle at high altitude. In: Roca J, Rodriguez-Roisin R, Wagner PD, editors. Pulmonary and peripheral gas exchange in health and disease. New York: Marcel Dekker, 2000.
53 Schoene RB. Limitations of respiration at high altitude. Clin Chest Med. 2005;26:405.
54 Short KR, Bigelow ML, Kahl J, et al. Decline in skeletal muscle mitochondrial function with aging in humans. Proc Natl Acad Sci U S A. 2005;102:5618.
55 Sutton JR, Reeves JT, Wagner PD, et al. Operation Everest II: Oxygen transport during exercise at extreme simulated altitude. J Appl Physiol. 1988;64:1309.
56 Tanaka H, Seals DR. Endurance exercise performance in masters athletes: Age-associated changes and underlying physiological mechanisms. J Physiol. 2008;586:55.
57 Trappe SW, Costill DL, Vukovich MD, et al. Aging among elite distance runners: A 22-yr longitudinal study. J Appl Physiol. 1996;80:285.
58 West JB, Boyer SJ, Graber DJ, et al. Maximal exercise at extreme altitudes on Mount Everest. J Appl Physiol. 1983;55:688.
59 West JB, Lahiri S, Maret KH, et al. Barometric pressures at extreme altitudes on Mt. Everest: Physiological significance. J Appl Physiol. 1983;54:1188.
60 Williams AG, Folland JP. Similarity of polygenic profiles limits the potential for elite human physical performance. J Physiol. 2008;586:113.
61 Zoll J, Ponsot E, Dufour S, et al. Exercise training in normobaric hypoxia in endurance runners. III. Muscular adjustments of selected gene transcripts. J Appl Physiol. 2006;100:1258.