Chapter 3 The Scientific Basis for Examination and Classification of Knee Ligament Injuries
CLASSIFICATION SYSTEM FOR KNEE LIGAMENT INJURIES
Many different classification systems for knee ligament injuries have been proposed in the sports medicine literature.20,21,31,32,42 A series of studies conducted by the authors enabled the development of an algorithm for the diagnosis and classification of these injuries based on kinematic and biomechanical data.8,14–17,36–42,55,60 The purpose of this chapter is to summarize these studies and provide the clinician with the proper examination techniques that allow precise diagnosis of abnormal knee motions, subluxations, and ligament injuries.
The classification scheme developed from the authors’ investigations is based on seven concepts:
Critical Points CLASSIFICATION SYSTEM FOR KNEE LIGAMENT INJURIES
Noyes, F. R.; Grood, E. S.: Classification of ligament injuries: why an anterolateral laxity or anteromedial laxity is not a diagnostic entity. Instr Course Lect 36:185–200, 1987.
Purpose of a Classification System
Authors’ Classification System Based on Seven Concepts
In this chapter, the studies presented relate to the anterior cruciate ligament (ACL), posterior cruciate ligament (PCL), medial collateral ligament (MCL) and posteromedial structures, the iliotibial band (ITB), and the midlateral capsule. Studies related to the posterolateral structures (fibular collateral ligament [FCL], popliteus muscle-tendon-ligament, and posterolateral capsule) are presented in Chapters 20, Function of the Posterior Cruciate and Posterolateral Ligament Structures, and 22, Posterolateral Ligament Injuries: Diagnosis, Operative Techniques, and Clinical Outcomes.
Concept 1: The Final Diagnosis of Knee Ligament Injuries Is Based on the Specific Anatomic Defect Derived from the Abnormal Motion Limit and Joint Subluxation
Concept 2: Ligaments Have Distinct Mechanical Functions to Provide Limits to Tibiofemoral Motions and the Types of Motions That Occur between Opposing Cartilage Surfaces
The ability of ligaments to limit tibiofemoral motion provides the geometric parameters within which the neuromuscular system is able to control the position of the knee during activity. Although focus is placed on the mechanical function of the ligaments and capsular structures, the reader should be cognizant of the potentially important role of ligaments in providing sensory feedback to the neuromuscular system.24,53 Ligaments have three properties that affect their ability to limit joint motion: location of their attachment on the bones, just-taut length, and stiffness.
The kinematic and biomechanical concepts required to interpret clinical tests are shown in Figure 3-1. First, the appropriate clinical test must be selected to diagnose a specific ligament structural abnormality. Diagnostic information is obtained based on understanding the primary and secondary ligament restraint system. The results of the tests must be understood and communicated in terms of the six-DOF system that determines the abnormal motion limits. The medial and lateral tibiofemoral compartments are examined separately to determine the different types of subluxation. The final diagnosis of the ligament defect must be made in precise anatomic and functional terms and according to the severity of ligament failure (partial or complete).
Concept 3: Although There Are Six DOF, the Manual Stress Examinations Are Designed to Test Just One or Two Limits at a Time
Translation of a rigid body (such as the tibia) is described by the motion of an arbitrarily selected point on the body. Typically, the AP translation is described by the motion of a point located midway between the medial and the lateral margins of the tibia. If only translation motions occur, the amount of motion does not depend upon which point is chosen, that is, whether the point is at the center of the knee or at the medial or lateral joint margin. This is because all points will move along parallel paths. However, when rotation and translation motions are combined, the amount of translation does depend upon which point is used. This can be seen by considering the four cases illustrated in Figure 3-2.
Figure 3-2B shows an anterior translation of 10 mm without associated tibial rotation. All points move anteriorly by the same amount. Figure 3-2C shows an internal rotation of 15° about an axis located midway between the spines of the intercondylar eminence. The point on the rotation axis is stationary while the lateral joint margin (edge) moves anteriorly and the medial margin posteriorly (see Fig. 3-2D). The amount of anterior and posterior motion of the points at the joint margin depends upon the amount of rotation and how far away the points are from the rotation axis (center of rotation). This illustrates that when translation is measured in the presence of a concurrent rotation, it is important to know at what point the translation was measured.
Concept 4: Ultimately, the Clinical Examination Must Be Analyzed by a Six-DOF System to Detect Abnormalities
The field of science that describes the motions between objects is known as kinematics. A fundamental aspect of this field is the recognition that six possible motions may occur in three dimensions. Each of the six motions is discrete and separate from the other five motions. The six motions are referred to as degrees of freedom (DOF). The three rotational DOF in the knee joint are shown in Figure 3-3. Each rotation occurs about one axis: flexion-extension, internal-external, and abduction-adduction.
The flexion-extension axis is located in the femur and oriented in a pure medial-lateral direction perpendicular to the femoral sagittal plane. Rotation of the tibia about this axis does not have associated internal-external rotation or abduction-adduction motions.17 Because these motions occur during flexion, the flexion-extension axis shown in Figure 3-3 does not correspond to the functional flexion axis. The functional flexion axis is skewed in the knee and changes its orientation as the knee is flexed. This skewed orientation accounts for the combined motions of flexion, abduction, and tibial rotation.
There are three linear DOF in the knee joint referred to as translations. One simple approach to describing translations is to visualize relative sliding between the bones along each of the three rotational axes (Fig. 3-4). The sliding motion along the flexion-extension axis is the medial-lateral translation between the tibia and the femur. The sliding motion along the tibial rotation axis results in joint compression and distraction translation. Sliding motions along the abduction-adduction rotation axis produce AP translations. These are also commonly known as drawer motions.
Therefore, six possible motions can occur in the knee, three rotations and three translations. Three axes are required to explain these six motions, one fixed in each bone, as shown in Figure 3-4. Each axis represents two DOF, one a rotation occurring about the axis and the other a translation.
Concept 5: Together, the Ligaments and Joint Geometry Provide Two Limits (Opposite Directions) for Each DOF
Together, the ligaments and joint geometry provide two limits (opposite directions) for each DOF. All together, there are 12 possible limits of motion of the knee (Table 3-1). Injury to the structures that limit each motion increases joint laxity. The position of the joint at the final limits of motions (reflecting the ligament attachment sites) provides the information required for diagnosis. From a diagnostic standpoint, it would be ideal if each of the 12 limits of motion were controlled by a single ligamentous structure. Differential diagnosis could then be performed by evaluating each of the 12 limits separately. Clearly, this ideal situation does not exist. The ligaments, capsular structures, and joint geometry all work together and each contributes to limiting more than one motion. Thus, the problem of diagnosing knee injuries reduces to determining how to apply individual or combination motions to lengthen primarily a single ligament or capsular structure so that structure can be evaluated independently.
Motion Limit | Structures Limiting the Motion |
---|---|
Flexion | Ligaments, leg and thigh shape, joint compression |
Extension | Ligaments and joint compression |
Abduction | Ligaments and lateral joint compression |
Adduction | Ligaments and medial joint compression |
Internal rotation | Ligaments and menisci |
External rotation | Ligaments and menisci |
Medial translation | Bones (spines interlocking with femoral condyles) and ligaments (to prevent distraction) |
Lateral translation | Bones (spines interlocking with femoral condyles) and ligaments (to prevent distraction) |
Anterior translation* | Ligaments |
Posterior translation* | Ligaments |
Joint distraction | Ligaments |
Joint compression | Bone, menisci, and cartilage |
* Menisci, joint compressive effects after injury to primary restraint.
The ability to isolate each structure is the key to differential diagnosis of individual ligament injuries. The isolation of a structure is accomplished by placing the knee at the proper joint position (specifically, knee flexion angle and tibial rotation position) before the clinical stress test is performed. For example, the abduction (valgus) stress test is performed both in full extension and at 20° to 30° of flexion. In the flexed position, the posterior capsule becomes slack, which allows the examiner to primarily load the MCL and midmedial capsule. The evaluation of ACL function is performed at 20° of knee flexion56 as opposed to the 90° position29 commonly used many years ago because the 20° position more often results in increased anterior subluxation because secondary restraints are more slack and less able to block this motion. Diagnosis of an injury to a specific ligament is performed at a joint position at which other structures are the most lax and least able to block the abnormal subluxation from the ligament injury. The lax secondary restraints allow an increase in joint motion before they become taut and resist further joint motion. Thus, isolating a ligament so its integrity may be individually tested requires placing the knee in a position in which other supporting structures are slack.
Another example of the importance of selecting the joint position for clinical tests is the diagnosis of PCL injury. Figure 3-5 shows the amount of increased posterior tibial translation that occurs when the PCL is removed.10,16,17 The increase in posterior translation is two to three times greater at 90° of flexion than at 20° of flexion. This phenomenon is easily understood using a bumper model analogy in which the amount of joint motion after a ligament is injured depends on the role and function of the remaining ligaments that must ultimately limit the joint motion (Fig. 3-6). Thus, the increase in joint motion that occurs when a ligament is injured reflects the amount of additional joint motion required before the remaining intact ligaments become stretched and are able to limit further motion.
Figure 3-7 illustrates the limits to internal tibial rotation in the knee joint.16 At 30° flexion (see Fig. 3-7A), the limits to internal rotation are provided by posteromedial structures, lateral structures, and the ACL all working together. Sectioning either the ACL or the lateral structures produces a small increase in internal rotation. When both of these structures are cut together, a larger increase in internal rotation occurs. The further limit to internal rotation is the FCL, based upon its anatomy.
The ACL dominates at flexion angles less than 30°, whereas the lateral structures dominate at flexion angles greater than 30°. This can be explained by considering the changes that occur in ligament slackness with flexion and extension. As the knee is extended past 20°, the amount of AP translation decreases owing to reduction in the combined slackness of both cruciate ligaments. This brings these bumpers closer together. The posteromedial capsule (PMC) also tightens, moving its bumper anteriorly. This combination (see Fig. 3-7B) results in a decreased role of the anterolateral structures because the tibia can no longer rotate to the point where they become taut.
Figure 3-8 illustrates the limits to external rotation. At 30° flexion, external rotation is limited only by the extra-articular restraints. On the lateral side, this includes all of the posterolateral structures, which act as a unit. Large increases in rotation do not occur until all structures are cut. At 90° flexion, the posterior capsule is slack and the PCL blocks significant increases in external rotation when the posterolateral structures are sectioned. In laboratory studies, external rotation increases an average of only 5.3° ± 2.6° when all of the posterolateral structures are sectioned and the PCL is intact. When the PCL is also sectioned, a large additional increase in external rotation occurs, ranging from 15° to 20°.
An example of the changes in motion limits in ACL ruptures is shown in Figure 3-9. In cadaver knees, cutting the ACL causes an abnormal increase in both anterior tibial translation and internal tibial rotation.8 The increase in anterior tibial translation is the primary abnormality, because it increases 100% while there is a small increase in internal rotation (approximately 15%). Cutting the ACL alone produced a small but significant increase in internal rotation, greatest at 0° and 15° (Fig. 3-10). Subsequently, sectioning the ITB and lateral capsule produced statistically significant increases at 30° and greater.
The amount of anterior tibial translation induced during anterior drawer testing is dependent upon the amount of internal or external tibial rotation applied at the beginning of the test (Fig. 3-11). The instrumented knee joint is shown for measuring rotations and translation motions during the clinical examination in Figure 3-12. This is because rotation tightens the extra-articular secondary restraints. The greatest amount of anterior or posterior tibial translation will be produced when the tibial is not forcibly rotated internally or externally, tightening extra-articular structures, during the clinical test. If the tibia is internally or externally rotated prior to the start of testing, the amount of tibial translation elicited will be smaller. Thus, the clinician controls the amount of translation both by the initial rotational position of the tibia and by the amount of rotation imposed during the test. There is considerable variation in examination techniques of clinicians that makes all of the clinical tests highly subjective and qualitative, as is discussed.
The pivot shift12 and flexion-rotation44 drawer (Fig. 3-13) tests involve a complex set of tibial rotations and AP translations. At the beginning of the flexion-rotation drawer test, the lower extremity is simply supported against gravity (Fig. 3-14, position A). After ligament sectioning, both anterior tibial translation and internal rotation increase as the femur drops back and externally rotates into a subluxated position.42 This position is accentuated as the tibia is lifted anteriorly (see Fig. 3-14, position B). At approximately 30° of flexion, the tibia is pushed posteriorly, reducing the tibia into a normal relationship with the femur (see Fig. 3-14, position C). This is the limit of posterior tibial translation resisted primarily by the PCL. From position C to position A, the knee is extended to produce the subluxated position again.
The pivot shift and flexion-rotation drawer tests are graded only in qualitative terms because it is not possible to determine accurately the actual amount of internal tibial rotation or anterior translation elicited. A fully positive pivot shift test (grade III) indicates a gross subluxation of the lateral tibiofemoral articulation along with an increased anterior displacement of the medial tibial plateau (Table 3-2). The amount of anterior subluxation elicited is indicative of rupture to the ACL and laxity to the secondary extra-articular restraints. The lateral tibial plateau demonstrates the greater subluxation in a positive pivot shift test, indicating that the lateral restraints (ITB, lateral capsule) are not functionally tight. This does not mean that these restraints are injured because a physiologic slackness of the ITB tibiofemoral attachments is normal at the knee flexion position used in this test. These attachments are tightest at knee flexion angles of 45° and higher. Therefore, the majority of knees with an isolated ACL tear will have a positive pivot shift phenomenon.
In knees with a grade III pivot shift test, the amount of anterior tibial subluxation is so great that the posterior margin of the lateral tibial plateau impinges against the lateral femoral condyle and blocks further knee flexion during the test. The examiner must add both a maximal anterior force and an internal tibial rotation force to determine whether the maximum subluxation position can be reached. In revision ACL reconstructions, a combined intra-articular graft and extra-articular ITB surgical approach is often considered, as is discussed.34
Concept 6: Rotatory Subluxations Are Characterized by the Separate Compartment Translations That Occur to the Medial and Lateral Tibial Plateaus during the Clinical Test
A simple concept may assist in explaining the abnormal motions that occur after ACL rupture: rotatory subluxations can be classified according to the amount of anterior and posterior translation of each tibiofemoral compartment. Figure 3-15A shows a Lachman test performed on a knee in which the combined motions of anterior tibial translation and internal tibial rotation occur about a medially located rotation axis. In this example, only planar motion occurs; the ACL rupture doubles the amount of anterior tibial translation and slightly increases internal tibial rotation. The rotation axis shifts medially. The ratio of tibial translation to degrees of internal tibial rotation determines how far medially the axis of rotation shifts.
The abnormalities in tibial rotation and translation are easily expressed in terms of the different amounts of anterior translation that occur to the medial and lateral compartments (see Fig. 3-15B) in biomechanical tests. During the clinical tests, the clinician may qualitatively palpate and observe the anterior or posterior translation of each tibial plateau. The AP translation of each plateau is characterized instead of defining the individual components of translation, rotation, and rotation axis location that all lead to the anterior subluxation. The combined effect of the rotation and translation determines the translation of the medial and lateral tibiofemoral compartments.
The type of rotatory subluxations that occur depends on both the ligament injury and the knee flexion position. The subluxations of the medial and lateral compartment are usually recorded at two knee flexion positions, such as 20° and 90°. To be described later is the dial test for posterolateral injuries, in which the examiner determines whether increases in external tibial rotation reflect anteromedial or posterolateral tibial subluxations. It should be noted that rotatory subluxations are historically based on increases in tibial internal or external rotation and in only a few studies have the actual medial and lateral tibial subluxations in an AP direction been determined.16,45 There are complex rotatory subluxations involving increases in translation, but in opposite directions of both the medial and the lateral compartments with combined medial and lateral ligament injuries.
Concept 7: The Damage to Each Ligament and Capsular Structure Is Diagnosed Using Tests in Which the Primary and Secondary Ligament Restraints Have Been Experimentally Determined
Tears to the ACL and injury to the extra-articular ligamentous and capsular structures may be diagnosed using the Lachman, pivot shift, and flexion-rotation drawer tests. These tests provide the basic signs that allow the clinician to determine which structures are injured based on abnormal motion limits and resultant joint subluxations. The tests are performed in knee flexion positions in which the secondary restraints are unable to resist abnormal motions so that maximum displacement (subluxation) of the joint is produced. Table 3-3 provides a general summary of the primary and secondary restraints for the major tests used in the clinical examination. Later in this chapter, the specific restraining function of the ligaments is discussed in detail.
The qualitative grading of the pivot shift phenomenon is illustrated in Figure 3-16 to explain how ligament structures resist combined tibiofemoral motions. The cruciate ligaments are represented by a set of central bumpers that limit the amount of AP translation. There are also medial and lateral sets of bumpers that resist medial and lateral tibiofemoral compartment translations. For the medial and lateral bumpers, different ligament structures commonly work together as systems to provide the resistance. The bumpers represent not the anatomic position of the ligament structures, but rather a visual schematic to show the final restraints to tibial motion, summarizing the effect of the ligaments, menisci, and capsular structures. The tension-retraining effect of the ligaments is replaced by an opposite mechanism, a compressive bumper.
In diagnosing abnormal knee motion limits, the Lachman test involves primarily tibial translation without significant tibial rotation, testing the central bumper represented by the ACL. A bumper model representation of a partial ACL tear or an ACL-deficient knee with tight medial and lateral extra-articular restraints that limit the amount of anterior tibial translation is shown in Figure 3-16A. The amount of central and lateral tibial translation is only slightly increased. The bumper model illustrates how the anterior restraints limit motion during the flexion-rotation drawer test, which allows the maximal anterior excursion of the medial and lateral tibiofemoral compartments. In this knee, the pivot shift test is qualitatively listed as a grade I.
The most common type of anterior subluxation that occurs after an ACL rupture is shown in Figure 3-16B. The center of rotation shifts medially outside the knee joint, with a resultant increase in translation to both the medial and the lateral compartments, with the anterior subluxation of the lateral compartment being the greatest. The anatomic structures include the ACL and the lateral extra-articular restraints. In this knee, the pivot shift test is qualitatively listed as a grade II.
A knee with gross anterior subluxation is represented in Figure 3-16C. There is increased translation and subluxation to both the medial and the lateral compartments and the rotation axis shifts even further medially outside the knee joint. The pivot shift test is listed as a grade III, indicative of gross subluxation with impingement of the posterior aspect of the tibia against the femoral condyle. Partial damage to the medial ligamentous structures may be present.
LIGAMENTOUS RESTRAINTS TO AP TRANSLATION
In a series of biomechanical cadaveric experiments,8 the ranked order of the importance of each knee ligament and capsular structure in resisting the clinical anterior and posterior drawer tests was determined, providing the primary and secondary restraints to specific knee motions. The ranked order was based on the force provided by each ligament in resisting AP translation.
Prior to these experiments, investigators performed studies in which selective sectioning of ligaments was conducted and the increases in anterior or posterior tibial displacement were measured.5,6,11,13,19,28,29,47,50, For example, the displacement test was done by applying a force on an intact knee, cutting a ligament, repeating the test, and measuring the increase in displacement. One problem with this experimental design is that the increase in displacement is dependent on the order in which the ligaments are sectioned. If this order is altered, the measured increase in displacement will change. Therefore, it is not possible to define the function of a single ligament in a precise manner. In addition, the amount of residual joint displacement after ligament sectioning is dependent on the just-taut length of the remaining ligaments, which varies between physiologic “tight” and “loose” knee joints.
Fourteen cadaver knees were tested from donors aged 18 to 65 years (mean, 42 yr). The knee specimens were mounted in an Instron Model 1321 biaxial servocontrolled electrohydraulic testing system (Fig. 3-17). A pair of grips was used for the femur and tibia that allowed for their precise position to be adjusted. First, the femur was secured with its shaft aligned along the axis of the load cell. The tibia was placed horizontally, with its weight supported by the lower grip. The output of the load cell was adjusted to zero to compensate for the weight of the upper grip and femur. The tibia was placed in a rotated position halfway between its limits of internal and external rotation. The output of the load cell was monitored while the tibia was secured in order to avoid pre-loading the ligaments. Single-plane anterior and posterior drawer tests were conducted by causing the actuator to move up and down without rotation. This is a constrained test in which coupled tibial rotation is purposely blocked. Specific details regarding the tests and data acquisition and statistical analyses are described in detail elsewhere.8
An AP drawer test is shown in Figure 3-18. Two curves are present, one for each direction of motion, as a result of the viscoelastic behavior of the knee ligaments. The peak restraining force of this specimen is approximately 500 N (112 lb) at 5 mm of drawer. The general shape of the force-displacement curve for the intact knee is nonlinear. The stiffness of the knee, or slope of the curve, is smallest near the neutral position and increases as the joint is displaced. The average restraining force in the intact knee is approximately 440 N (95 lb) at 90° of flexion and approximately 333 N (75 lb) at 30° flexion. This is comparable with forces expected during moderate to strenuous activity and is well above the manual force applied during clinical drawer testing.27,28
The effect of sectioning the ACL is shown by the decrease in slope of the anterior curve and increase in displacement in Figure 3-18. Note that the anterior curve does not drop to zero owing to the presence of secondary ligament restraints. The ACL is the primary restraint to anterior translation (Fig. 3-19). Its contribution at displacements from 1 to 5 mm is shown in Figure 3-20. The percentages given above the bars are for 90° of knee flexion. Nearly identical results are shown at 30° of flexion, which represents the position of the knee during the Lachman test. No significant differences were found between 1 and 5 mm of drawer regardless of the trend toward increasing percentages at larger joint displacements.
The secondary restraints to anterior translation when the ACL is sectioned are shown in Table 3-4. The range in values for each structure demonstrates the large variation in results between specimens. No statistical difference was found among the percentages calculated. The contributions of the PCL, the anterior and posterior capsules, and the popliteal tendon were not included because they provided minimum restraining force.
It is important to characterize the effect of the ACL and secondary restraints on the coupled motions of anterior translation and internal-external tibial rotations. Figure 3-21 shows the effect of the lateral secondary restraints on both anterior translation and internal tibial rotation.
The PCL provides 94.3% ± 2.2% of the total restraining force at 90° of knee flexion, with similar findings at 30° of flexion (Fig. 3-22). No other structure contributes greater than 3% of the total restraint. The secondary restraints to posterior drawer after the PCL is sectioned (including the lateral meniscofemoral ligament when present) are shown in Table 3-5. The posterolateral capsule and popliteus tendon (combined contribution, 58.2%) and the MCL (15.7%) provided the greatest restraint. The posterior medial capsule, FCL, and midmedial capsule contributed only modest restraints. The combined restraint provided by the posterolateral capsule and popliteus tendon was significantly different from those provided by the other structures.
Critical Points LIGAMENTOUS RESTRAINTS TO ANTEROPOSTERIOR TRANSLATION
This investigation was the first to introduce the concepts of primary and secondary ligament restraints to joint motion. The cruciate ligaments are the primary restraints to AP drawer and provide approximately 90% of the total restraining force at 5 mm of joint displacement. The remaining structures provide only a small contribution. This study also made a distinction between clinical forces, which are small loads applied to the knee during a clinical examination, and functional forces, which are large in vivo loads experienced during moderate or strenuous activities (Fig. 3-23). The increase in joint displacement after the loss of either the ACL or the PCL depends on the forces applied to the knee. Whereas the light forces applied during a clinical test may produce only a slight increase in joint displacement, these increases are expected to be much larger under moderate or strenuous functional forces. Therefore, all clinical ligament examination tests do not predict the magnitude of joint displacement that may occur during functional activities.
LIGAMENTOUS AND CAPSULAR RESTRAINTS RESISTING MEDIAL AND LATERAL JOINT OPENING
The authors previously determined the ligaments and capsular structures that resist medial and lateral joint opening in cadaver knees. The ligaments were ranked in order of importance based on the percent of the total restraining force that each provides. The results are independent of the order in which ligaments are sectioned, allowing all ligaments to be studied in each cadaver knee. Most prior studies of knee ligament function were based on knee motion limits after cutting selected ligaments6,19,28,58 or on the injury patterns associated with observed clinical pathologies.5,9,20,21,25,33,46,47,54
An Instron model-1321 biaxial testing system was used in which the femur was secured to the load cell with two grips that allowed its position to be adjusted.15 The tibia was attached to the actuator through a plantar hinge mechanism that prevented axial rotation and flexion of the tibia during testing. Each knee was placed to the full-extended (hyperextended) position by applying a 5-Nm extension moment. The tests were performed with the knee flexed 5° and 25° from this position. Single-plane varus and valgus displacements were produced by causing the actuator to move up and down, but not rotate. The tests were done in a fixed manner that first produced medial and then lateral joint opening. A constant rate was used so that peak opening occurred in 1 second. A series of 25 conditioning tests that produced 6 mm of medial and 6 mm of lateral joint opening were done at the two knee flexion angles. Typically, the peak force changed less than 0.25% per cycle during the last 5 conditioning tests. Then, baseline tests were done at both knee flexion angles. A ligament was cut and the test repeated. The restraint due to the cut ligament was calculated to be the decrease that occurred in the joint restraining moment compared with the moment determined in the test prior to the ligament sectioning. This process was repeated after cutting other structures until the restraining moments due to all of the ligamentous and capsular structures had been measured. The medial and lateral tests were performed in 16 knees obtained from 11 cadavers 18 to 55 years old (mean, 36.8 yr).
In six other cadaver lower limbs, the three-dimensional motion of the knee joint was measured during the clinical examination for medial and lateral joint opening. The motions were determined first in the intact knee and then after a collateral ligament was sectioned to measure the increase in joint opening. The opposite collateral ligament was then sectioned and the increase in joint opening documented. The goals were to determine the actual motions produced in uninjured knees during a clinical examination and to evaluate the change in joint opening that occurred with cutting each collateral ligament. The knee motions were measured using the instrumented kinetic chain55 (Fig. 3-24), which was positioned across the knee on the lateral side. The leg was positioned over the side of a table. The joint line was palpated with one hand while a force was applied at the ankle with the other hand. The force applied was not measured in order to conduct the examination in the normal manner. In order to make these measurements, it was necessary to know the position of the ends of the instrumented chain with respect to the femur and tibia. These positions were established by performing a three-dimensional analysis using biplane radiographs.7 Tests for reproducibility demonstrated that translational and rotational motions could be measured within ±0.5 mm and ±0.5°, respectively.
The ligaments and capsular structures studied were the ACL, PCL, superficial parallel fibers of the MCL, the FCL, the popliteus musculotendinous unit including the popliteofibular ligament (POP), the medial and lateral halves of the capsule (subdivided into anterior, middle, and posterior thirds), and the femorotibial portion of the ITB. The middle third of the medial capsule was considered to be the deep fibers of the MCL described by others.54,59 The posterior third included the complex of capsular structures previously detailed22,59 and the remaining portion of the medial portion of the capsule extending to the midpopliteal region (including the oblique popliteal ligament). The lateral half of the capsule was divided into the anterior third (from the lateral margin of the patellar tendon to Gerdy’s tubercle), the middle third (from Gerdy’s tubercle to just anterior to the FCL), and the posterior third (the popliteus muscle-tendon-ligament unit and the rest of the capsule extending back to the midpopliteal region).
Results
The results of a typical test on an intact knee and then after sectioning the MCL are shown in Figure 3-25. The curve marked “Intact” represents behavior after conditioning but before ligaments were sectioned. The restraining moment was greater during loading (upper curve) than during unloading (lower curve) owing to the viscoelastic properties of the ligaments.
During clinical testing in which varus and valgus forces of 45 N are applied at the ankle, a moment of approximately 18 Nm is produced at the knee. These moments produced a medial and lateral joint opening in the knee shown in Figure 3-26. When the MCL was cut (“MCL CUT” curve) and a valgus moment applied, the medial opening increased approximately 3 mm. The secondary restraints blocked further joint opening, because they were sufficient to resist the small forces typically induced during a clinical examination. The restraining moment produced by the MCL alone is shown in Figure 3-26.
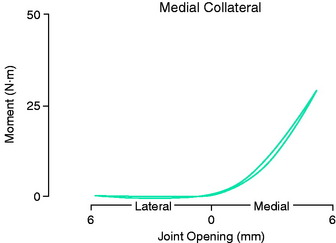
FIGURE 3-26 The curve for the restraining moment of the MCL alone versus joint opening. This curve was obtained by subtracting the curve after the L was cut from the curve for the intact knee shown in Figure 3-25.
(From Grood, E. S.; Noyes, F. R.; Butler, D. L.; Suntay, W. J.: Ligamentous and capsular restraints preventing straight medial and lateral laxity in intact human cadaver knees. J Bone Joint Surg Am 63:1257–1269, 1981.)
Medial Restraints
The ligaments and capsular structures resisting 5 mm of medial joint opening are shown for 5° of flexion in Figure 3-27 and for 25° of flexion in Figure 3-28. The MCL was the primary restraint at both knee flexion angles, providing 57.4% ± 3.5% of the total restraining moment at 5° and 78.2% ± 3.7% at 25° of flexion. The increase in contribution with flexion was due primarily to a decrease in the contribution of the posteromedial portion of the capsule, which became increasingly slack as flexion occurred. The anterior and middle parts of the medial half of the capsule provided weak secondary restraint limiting medial joint opening, equivalent to 7.7% ± 1.7% of the total restraint at 5° and to 4.0% ± 0.9% of the total at 25° with 5 mm of opening. The posterior portion of the medial half of the capsule provided 17.5% ± 2.0% of the total restraint at 5° and 5 mm of medial opening. At 25° of flexion, the restraint due to this part of the capsule dropped to 3.6% ± 0.8%. The effect of increasing medial joint opening on the contribution of the MCL is shown in Figure 3-29. At 5° of knee flexion, the contribution of the MCL decreased from 70.0% at 2 mm to 53.2% at 6 mm of opening.
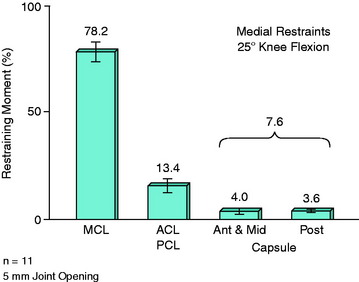
FIGURE 3-28 The percent restraining contributions of the medial structures at 5 mm of opening and 5° of flexion.
(Redrawn from Grood, E. S.; Noyes, F. R.; Butler, D. L.; Suntay, W. J.: Ligamentous and capsular restraints preventing straight medial and lateral laxity in intact human cadaver knees. J Bone Joint Surg Am 63:1257–1269, 1981.)
Cruciate Ligaments
The medial restraint provided by the ACL and PCL in combination was 14.8% ± 2.1% of the total at 5° of flexion and 13.4% ± 2.7% at 25°. In nine specimens, the contribution of one cruciate was separated from that of the other (Table 3-6). At 25° flexion, the PCL accounted for approximately 75% of the combined restraint exerted by the cruciates to medial opening, and the ACL accounted for 25%. This result did not depend on the order of cruciate sectioning. However, at 5° of flexion, the order of cruciate ligament sectioning affected the results. When the PCL was cut first, it accounted for approximately 70% of their combined restraint. When the PCL was cut after the ACL, its contribution was only 20% of their combined restraint. These findings indicate that the cruciates do not function independently of each other when the knee is near full extension.
Lateral Restraints
The average contributions of the lateral ligaments, lateral half of the capsule, and cruciate ligaments to the restraining moment at 5 mm of lateral joint opening are shown in Figure 3-30 at 5° of flexion and in Figure 3-31 at 25° of flexion. The FCL was the primary restraint limiting lateral opening of the joint at both knee flexion angles, providing 54.8% ± 3.8% of the total restraint at 5° of flexion and 69.2% ± 5.4% at 25°. The increased contribution of the FCL with knee flexion was due to a marked decrease in the restraint provided by the posterolateral capsule. There was a large variability in the data for the FCL, with its contribution ranging from 34.6% to 8.37% at 5° and from 40.5% to 94.7% at 25°. Still, the FCL provided a restraining moment greater than the combined moments of the entire lateral half of the capsule, the ITB, the popliteus tendon, and the cruciate ligaments.
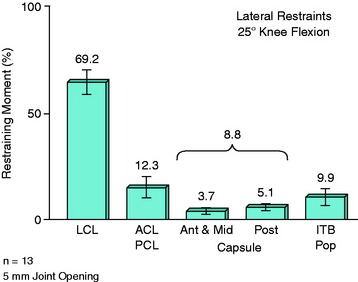
FIGURE 3-31 The percent contribution of the lateral structures at 5 mm of lateral joint opening and 25° of knee flexion.
(Redrawn from Grood, E. S.; Noyes, F. R.; Butler, D. L.; Suntay, W. J.: Ligamentous and capsular restraints preventing straight medial and lateral laxity in intact human cadaver knees. J Bone Joint Surg Am 63:1257–1269, 1981.)
The entire lateral half of the capsule provided 17.2% of the varus restraint at 5° of flexion (see Fig. 3-30) and 8.8% of this restraint at 25° (see Fig. 3-31). The anterior and middle thirds of the lateral half of the capsule contributed only a small secondary restraint, 4.1% ± 1.5% at 5° and 3.7% ± 1.5% at 25°. The posterolateral capsule became slack with flexion, and provided only 5.1% ± 1.3% of the total restraint at 25° of flexion.
Cruciate Ligaments
The cruciate ligaments together provided 22.2% ± 2.6% of the total restraining moment at 5° of flexion and 12.3% ± 4.2% at 25°. There was no effect on the order of cruciate sectioning. However, there was large variation in these data between specimens. The combined restraint of the cruciates ranged from 10.7% to 36.7% at 5° of flexion and from 1.9% to 45.7% at 25° flexion. The ACL provided the greater portion of the combined restraining effects (Table 3-7) at both knee flexion angles.
TABLE 3-7 Percentage of Restraining Moment Due to the Cruciate Ligaments for Lateral Joint Opening*
Ligament | 5° Knee Flexion | 25° Knee Flexion |
---|---|---|
ACL | 19.7 ± 2.7 | 10.3 ± 4.1 |
PCL | 2.7 ± 0.7 | 4.1 ± 1.1 |
Both | 22.4 ± 3.0 | 14.4 ± 4.7 |
ACL, anterior cruciate ligament; PCL, posterior cruciate ligament.
Iliotibial Tract, Popliteus Tendon, and Biceps Tendon
The effect of lateral opening on tension in the ITB and in the biceps tendon was investigated by applying a 225-N (50-lb) force to the ITB with a deadweight-and-pulley system. A curve of the restraining moment is shown in Figure 3-32 for an intact knee before and after the tension was applied. The tension produced an increase in the lateral restraining moment. The effect of the tension alone (Fig. 3-33) demonstrated that the applied force produced a nearly constant restraining moment, independent of the amount of lateral joint opening.
Location of Rotation Axes
The locations of the axes for varus-valgus tests on the femur at 5° of flexion are shown in Figure 3-34. The tibia, which moves during the test, is drawn in the neutral position, corresponding to the beginning of the varus-valgus loading test. The rotation axes are located above the joint contact area on the lateral femoral condyle for valgus displacement and above the medial contact area for varus displacement. The lower points represent the rotation axes for the first half of the varus and valgus test (~0–2.5 mm of joint opening). The upper points represent the axes for the last half of each test (2.5–5 mm of opening). For the total varus and valgus motion, the axes are located near the midpoints of the lines connecting the lower and upper points.15
The reader should note that the positions of the axes above the joint line indicate that a tibiofemoral sliding motion occurs during the loading test. The tibia slides laterally during a valgus test in the same direction as the applied force. The opposite sliding motion occurs during a varus test as the tibia moves medially. The proximal movement of the instant center reflects an increase in the amount of medial-lateral shear movement for each degree of varus-valgus rotation during the test. The increase in the amount of shear movement per degree of rotation occurs when the rotational stiffness of the joint increases more rapidly than its shear stiffness.15
Joint Motions during Clinical Examination
The increases in joint opening after the collateral ligaments were sectioned are shown in Table 3-8. The increases in motion after cutting the FCL during varus testing were 0.84 ± 0.5 mm at 5° of flexion and 2.56 ± 0.8 mm at 25° of flexion. The greater amount of joint opening with flexion was explained by the loss of the restraint provided by the posterior portion of the capsule and the increase in the contribution of the FCL. However, the increases in motion at both knee flexion angles were small owing to the influence of the secondary restraints under the low forces applied during the clinical examination.
TABLE 3-8 Increase in Joint Opening after Sectioning of the Collateral Ligaments*
Mean ± Standard Deviation (mm) | Range (mm) | |
---|---|---|
Lateral | ||
5° flexion | 0.84 ± 0.46 | 0.3–1.3 |
25° flexion | 2.56 ± 0.80 | 0.4–2.3 |
Medial | ||
5° flexion | 1.24 ± 0.69 | 1.7–3.7 |
25° flexion | 3.90 ± 1.43 | 2.0–5.5 |
* N = 5. All numbers are in millimeters of joint opening; 1 mm is equivalent to approximately 1° of tibial angulation.
The increases in medial joint opening after the MCL was sectioned during valgus testing were larger. This was due to the larger contribution to varus-valgus restraint provided by the MCL in comparison with that provided by the FCL. The largest increase measured in medial joint opening was 5.5 mm in one knee at 30° of flexion; however, the average increase was less than 5 mm. Therefore, only small joint openings may be demonstrated on clinical examination even when the medial or lateral primary restraint is ruptured. A 5- to 8-mm increase measured clinically after an acute injury indicates significant collateral ligament injury, including the secondary restraints. The concept of a “grade I laxity” (defined as an up to 5-mm increase in medial or lateral joint opening) as not representing a significant injury is not supported by these data. This raises the need to carefully evaluate any increase in joint opening, because this represents significant damage to the restraining function of a primary collateral ligament. The treatment aspects of acute injuries to the medial and lateral knee ligaments are discussed in Chapters 22, Posterolateral Ligament Injuries: Diagnosis, Operative Techniques, and Clinical Outcomes, and 24, Medial and Posteromedial Ligament Injuries: Diagnosis, Operative Techniques, and Clinical Outcomes. Table 3-9 shows the traditional classification system of the American Medical Association for medial and lateral ligament injury. A second-degree injury has only a few millimeters of joint opening, which is barely discernible. An increase up to 5 mm would represent a third-degree injury. Rather than use grades to classify the injury, it is more accurate to define the degrees of injury as first, second, or third and then estimate the millimeters of increased joint opening at 5° and 25° of flexion. Sequential increases of even 3 mm (rather than 5 mm) have important implications of injury to additional ligament structures that, in turn, effect treatment options.
Critical Points LIGAMENTOUS AND CAPSULAR RESTRAINTS RESISTING MEDIAL AND LATERAL JOINT OPENING
The middle third of the medial capsule was considered to be the deep fibers of the MCL.
The ITB functions as a single unit; when removed from its proximal pelvic attachments, the femorotibial portion becomes slack and incapable of restraining lesser amounts of lateral opening. The major function of the proximal muscle fibers appears to be the transmission of the tension maintaining a tautness of the ITB.15 There appears to be two main sources of tension in the tract23: the passive ligament-like tension between the iliac and the femoral insertions of this structure and the active muscle forces transmitted by the tract. The passive ligament-like tension should increase during lateral joint opening. The ilium-to-tibia distance is so long, however, that lateral joint opening of a few millimeters would not be expected to produce much additional tension in the tract.15
Conclusions
In cases of ACL rupture, the cradled position (holding the lower leg above the table) to induce varus or valgus tests should be avoided. With the leg elevated, an anterior tibial displacement occurs.35 The joint is partially subluxated and a medial to lateral rocking motion can be obtained that may be misinterpreted as increased medial or lateral joint opening. To prevent this, the knee should be examined with the thigh supported by the examination table in a reduced position in which the weight of the leg prevents the anterior tibial subluxation.
FUNCTION OF MEDIAL AND POSTEROMEDIAL LIGAMENTS IN ACL-DEFICIENT KNEES
The motion limits in normal knees and ACL-deficient cadaveric limbs were studied to define the role of the medial ligamentous structures in limiting anterior translation, abduction (degrees of medial joint opening), and external and internal tibial rotation.18 The results provide a scientific basis for clinical tests for the diagnosis of combined ACL-MCL ruptures. A six-DOF instrumented spatial linkage at the knee joint was used to measure the motion limits under defined loading conditions using the techniques previously published.55 The forces and moments in the experiment were: 100 N for anterior and posterior motion limits, 15 Nm for abduction-adduction limits, and 5 Nm for internal-external tibial rotation limits. After the motion limits were determined in the intact cadaveric knee, the ACL, MCL, and PMC were sectioned in different patterns to determine function when cut alone or after one of the other ligament structures. The ligaments cut were the ACL, superficial long fibers of the MCL (including deep medial one third meniscofemoral but not meniscotibial), and the entire PMC, including the posterior oblique portion.
The increases in motion limits are shown in Table 3-10. The changes in the anterior translation limits after the ligament sectioning procedures are shown in Figure 3-35. The typical pattern of a major increase in anterior translation at low flexion angles as compared with high flexion angles was statistically significant (P < .001). Note that subsequent sectioning of the MCL resulted in major increases in anterior translation at high flexion angles, with the amount of anterior translation equal at low flexion angles. This means that major increases in anterior translation at 90° knee flexion indicate that the secondary restraints are also insufficient. When the ACL was intact, there was no increase in anterior translation even when all of the medial ligament structures were sectioned.
TABLE 3-10 Increases in Motion Limits Relative to the Intact Knee That Occurred When the Indicated Structures Were Sectioned*
The changes in coupled internal and external tibial rotation during the anterior translation and abduction loading tests are shown in Table 3-11. The anterior loading produced a coupled internal tibial rotation, as expected. The coupled internal tibial rotation decreased after the ACL was sectioned, but still remained. However, sectioning the MCL produced a loss of the coupled internal rotation. This indicates the importance of the MCL in maintaining a rotation point for the coupled internal rotation to occur that is lost with MCL insufficiency, as already discussed. In pivot shift tests with a combined ACL-MCL injury, the magnitude of anterior tibial subluxation results in a grade III pivot shift (tibial impingement). In the abduction (valgus) test for medial joint opening, as long as there is an intact ACL, a coupled internal tibial rotation occurs. However, with an ACL and MCL injury, this internal tibial rotation with abduction is lost and there is an associated increase in external tibial rotation. These subtle changes in internal tibial rotation with combined ACL-MCL injuries are important, because the obligatory coupled rotation of anterior translation–internal tibial rotation is lost, which can be detected on clinical examination.
TABLE 3-11 Mean Degrees of Coupled Rotation during Anterior Translation and Abduction Tests for Intact Knees and after Ligament Sectioning*
The increase in the external tibial rotation limit with ligament sectioning is shown in Figure 3-36. Sectioning of the MCL produced major increases in external tibial rotation that increased with each subsequent ligament sectioning. The MCL acted as the primary restraint to external tibial rotation at all flexion angles. Cutting just the PMC (ACL, MCL intact) did not result in any increase in external tibial rotation (see Table 3-10).
The increase in the internal tibial rotation limit is shown in Figure 3-37. Note that the internal tibial rotation limit increases in the intact knee with knee flexion. ACL sectioning produced small increases in internal rotation (<3°) at 0° and 15° knee flexion (see Table 3-10). Sectioning the PMC alone had no effect on internal tibial rotation; however, PMC sectioning after MCL and ACL sectioning produced a major increase in the internal limit from 0° to 45° knee flexion.
The changes in the abduction rotation limits in the intact knee and with ligament sectioning are shown in Figure 3-38. The MCL was the primary restraint; however, the data show only small increases in abduction (medial joint opening) with complete MCL sectioning, with further increases in the motion limits after the PMC was sectioned. The ACL cut allowed for even further increases in abduction, indicating that it is a secondary restraint after the medial ligaments (MCL, PMC) are cut.
The results of the authors’ studies are in agreement with recently published data in cadaveric knees. Robinson and coworkers51 studied the superficial medial collateral ligament (SMCL) and deep medial collateral ligament (DMCL) and the PMC and measured the changes in motion limits under AP drawer, valgus, and internal-external rotation loads by sequential MCL cutting in 18 cadaveric knees. These authors reported that the PMC limited valgus, internal rotation, and posterior drawer in extension, resisting 42% of a 150-N drawer force when the tibia was in internal rotation (Figs. 3-39 to 3-42). The SMCL resisted valgus at all angles and was dominant from 30° to 90° of flexion, plus internal rotation in flexion. The DMCL resisted tibial anterior translation of the flexed and externally rotated knee and was a secondary restraint to valgus.
In regard to the anatomic description in these biomechanical experiments, Robinson and associates52 dissected the MCL and capsular structures in 20 cadaver knees and reported on the anatomy of the SMCL, DMCL, and PMC. In the PMC, there were oblique fibers, referred to as capsular condensations, that attached at the posterior margin of the SMCL femoral attachment at the femoral epicondyle, proceeding in a distal direction to blend in with the capsule and semimembranosus tendon sheath expansions. These capsular fibers tightened with internal tibial rotation, and the entire PMC tightened with knee extension. The authors reported that the three distinct bands corresponding to the posterior oblique ligament (POL) described by Hughston and Eilers22 could not be identified, preferring instead to use the nomenclature of the PMC.
EFFECT OF SECTIONING THE MCL AND the PMC ON POSTERIOR TIBIAL TRANSLATION
Ritchie and colleagues49 studied in 14 cadaver knees the contribution of various structures in the PCL-deficient knee in resisting posterior tibial translation.49 Single-plane posterior drawer tests were performed with the knee in neutral tibial rotation and in 20° of internal tibial rotation. The authors reported that with the knee in internal tibial rotation, posterior displacement was significantly less compared with that in neutral rotation when the SMCL was sectioned. The results showed that the SMCL was responsible for a decrease in posterior tibial translation in the PCL-deficient knee and not the PMC, including the POL.
ROLE OF THE POL
Petersen and coworkers,48 in a cadaveric study using a robotic testing system, examined the restraint of the SMCL, the DMCL, the POL, and the PMC in resisting posterior tibial translation after PCL sectioning. The study reported that the POL has a much larger role than the SMCL and DMCL in resisting posterior tibial translation and internal tibial rotation. It should be noted that these two structures were first sectioned; no studies were done when the POL was sectioned first. This indicates there could be a sectioning artifact introduced in the study. Even so, there are posteromedial oblique capsular fibers from the lateral femoral condyle to the tibia, just posterior to the SMCL, that resist internal tibial rotation and posterior tibial translation (after PCL sectioning). The study concluded that there are discrete oblique fibers that form the middle arm of the POL described by Hughston and Eilers.22 Of interest in this cadaveric study was the finding that a valgus loading of the knee joint close to knee extension (with PCL-deficiency) produced an increased posterior tibial translation of the medial compartment with absence of the POL and PMC.
Robinson and associates52 conducted a cadaveric study of the medial and posteromedial structures and could not identify a distinct separate POL structure, but did identify an oblique portion of the PMC where fibers could be tensioned under internal tibial rotation loading. Robinson and coworkers51 and Haimes and associates18 studied the contribution of the PMC, which included the POL. Robinson and coworkers51 reported that the PMC resisted 28% of the posterior tibial load when the tibia rotated freely in the extended knee, which rose to 42% when the tibia was subjected to internal rotation. These authors concluded that the PMC resisted posterior tibial translation close to full extension, and less so with knee flexion, which relaxes the PMC. With knee flexion, the SMCL resisted posterior tibial translation.
VARIABILITY BETWEEN CLINICIANS DURING CLINICAL KNEE LIGAMENT TESTING
An investigation was conducted with 11 experienced knee surgeons to determine differences in clinical examination testing techniques, accuracy in estimating knee displacements, and skill in diagnosing specific ligament injuries in knees with multiple abnormal motion limits.36,40 Knee joint positions and abnormal motions were measured in right-left cadaveric knees by a three-dimensional instrumented spatial linkage. A comparison was made of the clinicians’ estimate of the knee motion limits and subluxations with the actual measured values. The three-dimensional limits of knee motion were measured in the laboratory under defined loading conditions before and after the clinicians’ examination.
AP Displacement
Wide variability existed among examiners in the starting position of knee flexion and tibial rotation for AP displacement during the Lachman test (Fig. 3-43) and for the amount of tibial translation and rotation induced. Whereas some of the clinicians displaced the knee to the maximal displacement limits obtained in the laboratory, others failed to do so by a wide margin. The conclusion was reached that there was a wide variation in the loads applied among the examiners during the tests.
Pivot Shift Testing
The starting position for the pivot shift test varied among examiners, but was typically close to 5° extension (Fig. 3-44). As the knee was flexed, varying amounts of anterior tibial translation and internal tibial rotation were produced. During flexion, the maximal amount of internal tibial rotation was achieved first, followed by the maximal amount of anterior tibial translation. Although the amount of anterior translation of the lateral tibial plateau was similar among examiners, large differences existed among the clinicians (range, 6–16.9 mm) in the amount of maximum anterior translation of the medial tibial plateau. Examiners who produced the greatest amount of internal tibial rotation during the pivot shift test also significantly limited the amount of anterior translation of the medial tibial plateau (R = –0.79; P < .01).
The maximal amount of anterior tibial translation and the limits to anterior and posterior translation produced by each examiner are shown in Fig. 3-45. The normal and abnormal limits of tibial translation are shown before and after combined ACL and MCL ligament sectioning. The maximal amount of anterior translation (central point) ranged from 10 to 18 mm among examiners, and the maximal amount of anterior subluxation of the lateral tibial plateau ranged from 14 to 19.8 mm.
The mean value for maximal internal tibial rotation induced during the pivot shift test was 15.8 ± 3.6° (range, 11°–24°). Maximal internal rotation occurred at an average knee flexion angle of 15.6° ± 5.2° (range, 5°–23°). The limits to internal and external tibial rotation are shown in Figure 3-46. Two examiners exceeded the normal intact internal tibial rotation limit obtained under 5 Nm of torque. Only a slight increase occurred in the degrees of internal tibial rotation after the ACL and MCL were cut. Increases in external tibial rotation limits were also measured after ACL/MCL sectioning, which occurred during the tibial reduction phase of the pivot shift maneuver.
A few examiners demonstrated variability during the pivot shift test in regard to enhancement of internal tibial rotation (Figs. 3-47 to 3-48). The millimeters of anterior translation of the medial, central, and lateral points varied depending on how the test was performed.
Medial-Lateral Joint Space Opening
During abduction and adduction rotation testing, the examiners were instructed to begin the tests with the femoral condyle in contact with the tibial plateau. Data from the medial joint space abduction test demonstrated that most of the examiners’ estimates were within 3 mm of the actual measured values in the laboratory (Fig. 3-49). The starting flexion angle averaged 11.6° (range, 3.1°–21.3°). Each examiner performed the tests at a different flexion angle and reached a different final tibiofemoral position in both the intact knee and the ACL/MCL-sectioned knee (Fig. 3-50).
Medial-Lateral Compartment Translations during External Tibial Rotation
After sectioning the ACL and MCL, the amount of external tibial rotation increased from 17.8° (intact knee) to 22.1°. Most of the examiners produced an increase in anterior translation of the medial tibial plateau during the external rotation test. The displacement of the medial and lateral tibial plateaus in both the intact and the ACL/MCL-sectioned states are shown in Figure 3-51. The average center of tibial rotation in both states was in the lateral tibiofemoral compartment. The lateral shift in the axis of tibial rotation is demonstrated in Figure 3-51B, along with the increase in anterior displacement (range, 3.0–8.5 mm) of the medial tibial plateau. Seven of the 11 examiners incorrectly diagnosed an injury to the posterolateral structures, even though the lateral tibial plateau did not displace further posteriorly.
Study Limitations and Conclusions
The investigation demonstrated that many examiners induced coupled motions during the pivot shift test of anterior tibial translation and internal tibial rotation to produce anterior tibial subluxation without constraining or enhancing either motion. These examiners produced a greater anterior subluxation of the medial and lateral tibial plateaus than those who induced greater amounts of internal tibial rotation, which significantly decreased anterior subluxation of the medial tibial plateau (P < .01; Fig. 3-52). The recommendation can, therefore, be made to avoid intentional enhancement of internal tibial rotation when performing this test to allow the tibia to subluxate in the least constrained manner.
Even though variation existed among examiners in the estimated displacements, 9 of the 11 clinicians correctly diagnosed the ACL/MCL injury. However, numerous errors were made in the diagnosis of other ligament injuries, most notably, to the posterolateral structures. An increase in external tibial rotation was interpreted by many examiners to be a result of a posterior subluxation of the lateral tibial plateau and, therefore, injury to the posterolateral structures. The abnormality was actually an anterior subluxation of the medial tibial plateau, created by sectioning the ACL and MCL. To avoid this misdiagnosis, the examiner should palpate the medial and lateral tibial plateaus and their position relative to the femoral condyle in the maximum position of external and internal tibial rotation.43,45 Because this provides only a qualitative estimate, the need exists for instrumented or radiographic methods to diagnose more accurately the complex rotatory subluxations of the knee joint.
DEFINITION OF TERMS FOR KNEE MOTIONS, POSITIONS, AND LIGAMENT INJURIES
Considerable discrepancy exists in the orthopaedic literature in the implied meanings of many terms commonly used to describe knee motions, positions, and ligament injuries. As a result, confusion may develop when clinicians communicate or compare the results of studies. In addition, the use of precise terminology is essential in the development of a valid ligament classification system, as described earlier in this chapter. In recognition of this problem, surgeons and scientists from two institutions conducted a study and made recommendations regarding the definitions of medical and engineering terms commonly used to describe the motion and position of the knee observed during clinical testing.43
A systematic format was adopted to (1) categorize the terminology used in major articles on knee ligament injuries, (2) compare terms used in the selected articles to determine whether unique definitions had evolved over time through common usage, (3) review and compare definitions of these terms from a variety of primary, secondary, and tertiary sources, and (4) provide a recommendation for use of these terms in the orthopaedic literature. Dictionaries were considered primary sources2,57; textbooks,26 secondary sources; and published articles, tertiary sources. Terms that had controversial or multiple definitions in the orthopaedic literature were classified according to the least ambiguous meaning based on simplicity and clarity.
The definitions of terms used to describe positions of the knee (position, dislocation, and subluxation) are shown in Table 3-12, and the terms used to describe motion of the knee (motion, displacement, translation, rotation, range of motion, limits of motion, coupled displacement and motion, constrained and unconstrained motion, force, moment, laxity, and instability) are shown in Table 3-13. The terms used to describe injury to the knee (sprain, rupture, and deficiency) are shown in Table 3-14.
When applied to a ligament, the term laxity is used to indicate slackness or lack of tension—a lax ligament. Ligaments may purposely be made slack in an uninjured knee by positioning the tibia so that the distance between the femoral and the tibial attachments is shortened. Laxity may be normal or abnormal; abnormal laxity may be congenital or result from an injury. The adjective abnormal should be used to indicate when laxity is pathologic. In the orthopaedic literature, the term laxity is also used to indicate looseness of a joint or some amount of motion that results from the application of forces and moments. One source defines laxity as “either normal free motion or greater than normal free motion, as of a joint.”3 The problem with this term is that the specific type of motion is not stated. For instance, the term anterior laxity of the knee may refer to the combined motions of both anterior translation and tibial rotation or just to the amount of anterior tibial translation. If the latter is true, it is preferable to use the term anterior translation, thus avoiding ambiguity and allowing the millimeters of anterior tibial translation that occurred under defined loads to be reported. If the amount of anterior translation reported is the difference between the injured and the contralateral knee, this should always be indicated.
The term sprain is usually defined as an injury to a ligament in which portions of the ligament are torn but not completely disrupted.1,3,4 The American Medical Association’s classification system sorts ligament injuries into three categories—first-, second-, and third-degree sprain—as previously described in Table 3-9.
1 Dorland W.A., editor. Dorland’s Illustrated Medical Dictionary, 25th ed, Philadelphia: W. B. Saunders, 1974.
2 Funk and Wagnall’s Standard Desk Dictionary. 7th ed. New York: Funk and Wagnall; 1976.
3 Landau S.I., editor. International Dictionary of Medicine and Biology. New York: John Wiley, 1986.
4 Stedman’s Medical Dictionary. 24th ed. Baltimore: Williams & Wilkins:1982.
5 Abbott L.C., Saunders J.B., Bost F.C., Anderson C.E. Injuries to the ligaments of the knee joint. J Bone Joint Surg. 1944;26:503-521.
6 Brantigan O.C., Voshell A.F. The mechanics of the ligaments and menisci in the knee joint. J Bone Joint Surg. 1941;23A:44-61.
7 Brown R.H., Burstein A.H., Nash C.L., Schock C.C. Spinal analysis using a three-dimensional radiographic technique. J Biomech. 1976;9:355-365.
8 Butler D.L., Noyes F.R., Grood E.S. Ligamentous restraints to anterior-posterior drawer in the human knee. A biomechanical study. J Bone Joint Surg Am. 1980;62:259-270.
9 Ellison A.E. Skiing injuries. Clin Symp. 1977;29:1-40.
10 Fukubayashi T., Torzilli P.A., Sherman M.F., Warren R.F. An in vitro biomechanical evaluation of anterior-posterior motion of the knee. Tibial displacement, rotation, and torque. J Bone Joint Surg Am. 1982;64:258-264.
11 Furman W., Marshall J.L., Girgis F.G. The anterior cruciate ligament. A functional analysis based on postmortem studies. J Bone Joint Surg Am. 1976;58:179-185.
12 Galway H.R., MacIntosh D.L. The lateral pivot shift: a symptom and sign of anterior cruciate ligament insufficiency. Clin Orthop Relat Res. 1980;147:45-50.
13 Girgis F.G., Marshall J.L., Monajem A.L. The cruciate ligaments of the knee joint. Anatomical, functional and experimental analysis. Clin Orthop. 1975;106:216-231.
14 Grood E.S., Noyes F.R. Diagnosis of knee ligament injuries: biomechanical precepts. In: Feagin J., editor. The Crucial Ligaments. New York: Churchill Livingstone, 1988.
15 Grood E.S., Noyes F.R., Butler D.L., Suntay W.J. Ligamentous and capsular restraints preventing straight medial and lateral laxity in intact human cadaver knees. J Bone Joint Surg Am. 1981;63:1257-1269.
16 Grood E.S., Stowers S.F., Noyes F.R. Limits of movement in the human knee. Effect of sectioning the posterior cruciate ligament and posterolateral structures. J Bone Joint Surg Am. 1988;70:88-97.
17 Grood E.S., Suntay W.J. A joint coordinate system for the clinical description of three-dimensional motion: application to the knee. J Biomech Eng. 1983;105:136-144.
18 Haimes J.L., Wroble R.R., Grood E.S., Noyes F.R. Role of the medial structures in the intact and anterior cruciate ligament–deficient knee. Limits of motion in the human knee. Am J Sports Med. 1994;22:402-409.
19 Hsieh H.H., Walker P.S. Stabilizing mechanisms of the loaded and unloaded knee joint. J Bone Joint Surg Am. 1976;58:87-93.
20 Hughston J.C., Andrews J.R., Cross M.J., et al. Classification of knee ligament instabilities. Part II: the lateral compartment. J Bone Joint Surg Am. 1976;58:173-179.
21 Hughston J.C., Andrews J.R., Cross M.J., Moschi A. Classification of knee ligament instabilities. Part I: The medial compartment and cruciate ligaments. J Bone Joint Surg Am. 1976;58:159-172.
22 Hughston J.C., Eilers A.F. The role of the posterior oblique ligament in repairs of acute medial (collateral) ligament tears of the knee. J Bone Joint Surg Am. 1973;55:923-940.
23 Kaplan E.B. The iliotibial tract. J Bone Joint Surg Am. 1958;40:817-832.
24 Kennedy J.C., Alexander I.J., Hayes K.C. Nerve supply of the human knee and its functional importance. Am J Sports Med. 1982;10:329-335.
25 Kennedy J.C., Fowler P.J. Medial and anterior instability of the knee. An anatomical and clinical study using stress machines. J Bone Joint Surg. 1971;53A:1257-1270.
26 Larson R.L., Jones D.C. Part II: dislocations and ligamentous injuries of the knee. In: Rockwood C.A.Jr., Green D.P., editors. Fractures in Adults. Philadelphia: J. B. Lippincott; 1984:1480-1591.
27 Markolf K.L., Graff-Radford A., Amstutz H.C. In vivo knee stability. A quantitative assessment using an instrumented clinical testing apparatus. J Bone Joint Surg Am. 1978;60:664-674.
28 Markolf K.L., Mensch J.S., Amstutz H.C. Stiffness and laxity of the knee—the contributions of the supporting structures. A quantitative in vitro study. J Bone Joint Surg Am. 1976;58:583-594.
29 Marshall J.L., Wang J.B., Furman W., et al. The anterior drawer sign: what is it? J Sports Med. 1975;3:152-158.
30 Mueller W., editor. The Knee—Form, Function and Ligament Reconstruction. New York: Springer-Verlag, 1983.
31 Muller W. Kinematics of the cruciate ligaments. In: The Cruciate Ligaments. Diagnosis and Treatment of Ligamentous Injuries About the Knee. New York: Churchill Livingstone; 1988:217-233.
32 Nicholas J.A. Report of the committee on research and education. Am J Sports Med. 1978;6:295-306.
33 Nicholas J.A. The five-one reconstruction for anteromedial instability of the knee. Indications, technique, and the results in fifty-two patients. J Bone Joint Surg Am. 1973;55:899-922.
34 Noyes F.R., Barber-Westin S.D. Revision anterior cruciate surgery with use of bone–patellar tendon–bone autogenous grafts. J Bone Joint Surg Am. 2001;83:1131-1143.
35 Noyes F.R., Bassett R.W., Grood E.S., Butler D.L. Arthroscopy in acute traumatic hemarthrosis of the knee. Incidence of anterior cruciate tears and other injuries. J Bone Joint Surg Am. 1980;62:687-695. 757
36 Noyes F.R., Cummings J.F., Grood E.S., et al. The diagnosis of knee motion limits, subluxations, and ligament injury. Am J Sports Med. 1991;19:163-171.
37 Noyes F.R., Grood E.S. Classification of ligament injuries: why an anterolateral laxity or anteromedial laxity is not a diagnostic entity. Instr Course Lect. 1987;36:185-200.
38 Noyes F.R., Grood E.S. Diagnosis of knee ligament injuries: five concepts. In: Feagin J., editor. The Crucial Ligaments. New York: Churchill Livingstone, 1988.
39 Noyes F.R., Grood E.S., Butler D.L., Malek M. Clinical laxity tests and functional stability of the knee: biomechanical concepts. Clin Orthop. 1980;146:84-89.
40 Noyes F.R., Grood E.S., Cummings J.F., Wroble R.R. An analysis of the pivot shift phenomenon. The knee motions and subluxations induced by different examiners. Am J Sports Med. 1991;19:148-155.
41 Noyes F.R., Grood E.S., Suntay W.J. Three-dimensional motion analysis of clinical stress tests for anterior knee subluxations. Acta Orthop Scand. 1989;60:308-318.
42 Noyes F.R., Grood E.S., Suntay W.J., Butler D.B. The three-dimensional laxity of the anterior cruciate deficient knee as determined by clinical laxity tests. Iowa Orthop J. 1983;3:32-44.
43 Noyes F.R., Grood E.S., Torzilli P.A. Current concepts review. The definitions of terms for motion and position of the knee and injuries of the ligaments. J Bone Joint Surg Am. 1989;71:465-472.
44 Noyes F.R., Mooar P.A., Matthews D.S., Butler D.L. The symptomatic anterior cruciate-deficient knee. Part I: the long-term functional disability in athletically active individuals. J Bone Joint Surg Am. 1983;65:154-162.
45 Noyes F.R., Stowers S.F., Grood E.S., et al. Posterior subluxations of the medial and lateral tibiofemoral compartments. An in vitro ligament sectioning study in cadaveric knees. Am J Sports Med. 1993;21:407-414.
46 O’Donoghue D.H. Treatment of acute ligamentous injuries of the knee. Orthop Clin North Am. 1973;4:617-645.
47 Palmer I. On the injuries to the ligaments of the knee joint. Acta Chir Scand. 91(suppl 53), 1938.
48 Petersen W.J., Loerch S., Schanz S., et al. The role of the posterior oblique ligament in controlling posterior tibial translation in the posterior cruciate ligament–deficient knee. Am J Sports Med. 2008;36:495-501.
49 Ritchie J.R., Bergfeld J.A., Kambic H., Manning T. Isolated sectioning of the medial and posteromedial capsular ligaments in the posterior cruciate ligament–deficient knee. Influence on posterior tibial translation. Am J Sports Med. 1998;26:389-394.
50 Robichon J., Romero C. The functional anatomy of the knee joint, with special reference to the medial collateral and anterior cruciate ligaments. Can J Surg. 1968;11:36-40.
51 Robinson J.R., Bull A.M., Thomas R.R., Amis A.A. The role of the medial collateral ligament and posteromedial capsule in controlling knee laxity. Am J Sports Med. 2006;34:1815-1823.
52 Robinson J.R., Sanchez-Ballester J., Bull A.M., et al. The posteromedial corner revisited. An anatomical description of the passive restraining structures of the medial aspect of the human knee. J Bone Joint Surg Br. 2004;86:674-681.
53 Schultz R.A., Miller D.C., Kerr C.S., Micheli L. Mechanoreceptors in human cruciate ligaments. A histological study. J Bone Joint Surg Am. 1984;66:1072-1076.
54 Slocum D.B., Larson R.L., James S.L. Late reconstruction of ligamentous injuries of the medial compartment of the knee. Clin Orthop Relat Res. 1974;100:23-55.
55 Suntay W.J., Grood E.S., Hefzy M.S., et al. Error analysis of a system for measuring three-dimensional joint motion. J Biomech Eng. 1983;105:127-135.
56 Torg J.S., Conrad W., Kalen V. Clinical diagnosis of anterior cruciate ligament instability in the athlete. Am J Sports Med. 1976;4:84-93.
57 Traupman J.C., editor. New College Latin and English Dictionary. New York: AMSCO School, 1966.
58 Warren L.A., Marshall J.L., Girgis F. The prime static stabilizer of the medial side of the knee. J Bone Joint Surg Am. 1974;56:665-674.
59 Warren L.F., Marshall J.L. The supporting structures and layers on the medial side of the knee: an anatomical analysis. J Bone Joint Surg Am. 1979;61:56-62.
60 Wroble R.R., Grood E.S., Cummings J.S., et al. The role of the lateral extra-articular restraints in the anterior cruciate ligament–deficient knee. Am J Sports Med. 1993;21:257-262. discussion 263