10 The nervous system
Afferent: Carrying sensory impulses toward the brain.
Autoregulation: An alteration in the diameter of arterioles to maintain a constant perfusion pressure during changes in systemic blood pressure.
Axon: A long, slender projection from the nerve cell body that transmits nerve impulses away from the cell.
Cistern: A reservoir or cavity.
Commissure: White or gray matter that crosses over in the midline and connects one side of the brain or spinal cord with the other side.
Decussate: Refers to crossing of parts from one side of the brain or spinal cord to the other.
Dendrite: Branched projection from the nerve cell body that transmits nerve impulses into the nerve cell.
Efferent: Carrying motor impulses away from the brain.
Glial Cells: Nonneuronal cells that maintain homeostasis for nerve tissue within the central nervous system.
Gray Matter: Central nervous system tissue consisting primarily of nerve cell bodies, glial cells, and capillaries.
Inferior: Beneath; also used to indicate the lower portion of an anatomic part.
Lower Motor Neurons: Neurons of the spine and cranium that directly innervate the muscles (e.g., those found in the anterior horns or anterior roots of the gray matter of the spinal cord).
Myelin: A product of glial cells that forms an insulating layer around axons, allowing faster nerve impulse conduction.
Neuroglia: The supporting structure of nervous tissue that consists of a fine web of tissue composed of neuroglia or glia cells. It performs supportive and nutritive functions for the nerve network but is not directly involved in nerve impulse transmission.
Postural Reflexes: Reflexes that are basically proprioceptive, concerned with the position of the head in relation to the trunk and with adjustments of the extremities and eyes to the position of the head.
Proprioception: Sensory input from joints, tendons, and muscles that transmit information regarding the position of one body part in relation to another.
Ramus (rami): The primary division of a nerve.
Synapse: A junction between two nerve cells.
Upper Motor Neurons: Neurons in the brain and spinal cord that activate the motor system (e.g., the descending fibers of the pyramidal and extrapyramidal tracts).
White Matter: Central nervous system tissue consisting mostly of myelinated axons.
The nervous system
The nervous system can be broadly divided into two components: the central nervous system (CNS) and the peripheral nervous system (PNS). Although these divisions are commonly used, the boundaries between them can be somewhat arbitrary. The flow of sensory information and motor control signals between the two elements of the nervous system is critical for its normal functioning and the health of the individual.
Central nervous system
The brain
Forebrain
Telencephalon, cerebral cortex
The cerebrum consists of two hemispheres interconnected by a large band of neurons known as the corpus callosum. Each hemisphere is further subdivided into four lobes that correspond in name to the overlying bones of the cranium. These lobes are the frontal, parietal, temporal, and occipital lobes (Fig. 10-1). Both hemispheres consist of an external cortex of gray matter, the underlying white matter tracts, and the basal ganglia (cerebral nuclei). Each hemisphere also contains a lateral ventricle, which is an elongated cavity concerned with the formation and circulation of cerebrospinal fluid (CSF).
Each hemisphere has three sulci between the lobes. The central sulcus (also known as the fissure of Rolando) separates the frontal and parietal lobes. The lateral sulcus (the fissure of Sylvius) lies between the frontal and parietal lobes above and the temporal lobe below. The small parietooccipital sulcus is located between its corresponding lobes (see Fig. 10-1).
The white matter of the cerebrum is situated below the cortex and is composed of three main groups of myelinated nerve fibers arranged in related bundles or tracts. The commissural fibers transmit impulses between the left and right hemispheres. The largest of these fibers is the corpus callosum. The projection fibers are afferent and efferent nerve fibers that transmit impulses between the cortex, lower parts of the brain, and the spinal cord. A notable example is the internal capsule that surrounds most of the basal ganglia and, in part, connects the thalamus and the cerebral cortex. Finally, the association fibers transmit impulses from one part of the cortex to another within the same hemisphere.1
Functional aspects of the cerebrum
Motor areas.
The premotor area of each hemisphere is located in the cortex immediately in front of the primary motor cortex in the frontal lobe. On the whole, this area is concerned with movement of the opposite side of the body, especially with control and coordination of skilled movements of a complex nature, such as throwing or kicking a ball. In addition to its subcortical connections with the primary motor area, its neurons also have direct connections with the basal ganglia and related nuclei in the brainstem, for example, the reticular formation. Many of the axons from these subcortical centers cross to the opposite side before descending as extrapyramidal tracts in the spinal cord. Collectively, the connections from the premotor area to these related nuclei compose the extrapyramidal system, which coordinates gross skeletal muscle activities that are largely automatic in nature. Examples are postural adjustments, chewing, swallowing, gesticulating during speech, and associated movements that accompany voluntary activities. Certain portions of the extrapyramidal tract also have an inhibitory effect on spontaneous movements initiated by the cerebral cortex and serve to prevent tremors and rigidity. Complete structural and functional separation of the pyramidal and extrapyramidal systems is impossible because they are so closely connected in the harmonious work of executing complex coordinated movements.
Tardive dyskinesia is a late-appearing neurologic syndrome that is characterized by stereotypic, involuntary, rapid, and rhythmically repetitive movements, such as continual chewing movements and darting movements of the tongue. Treatment is not always satisfactory because antiparkinsonian drugs sometimes exacerbate tardive dyskinesia. Tardive dyskinesia often persists despite discontinuation of the responsible drug.2,3
Two important structural aspects of the premotor area are worth noting for those who care for neurosurgical patients. First, the fibers from both the primary motor and the premotor areas are funneled through the narrow internal capsule as they descend to lower areas of the CNS. This action is significant because the internal capsule is a common site of cerebrovascular accidents that can result in a variety of motor deficits. Second, lesions within one side of the internal capsule result in paralysis of the skeletal muscles on the opposite side of the body because of the crossing of fibers within the medulla.4
Sensory areas.
The auditory area lies in the cortex of the superior temporal lobe. Each hemisphere receives impulses from both ears. The visual area is located in the posterior occipital lobe, where extremely complex transformations in the signals conveyed by the optic nerve occur. The right occipital cortex receives impulses from the right half of each eye, and the left occipital cortex receives impulses from the left half of each eye. The olfactory area is believed to be located in the medial temporal lobe, and the gustatory area is located nearby at the base of the postcentral gyrus.
Limbic system.
The principal structural and functional units of the limbic system are the two rings of limbic cortex and a number of related subcortical nuclei, the anterior thalamic nuclei, and portions of the basal nuclei (Fig. 10-2). In general, the limbic system is concerned with a wide variety of autonomic somatosensory and somatomotor responses, especially those involved with emotional states and other behavioral responses. Within the limbic system, the benzodiazepine and opiate receptors have been identified (see Chapters 19, 21, and 22).
Basal ganglia.
A cerebral nucleus is a group of neuron cell bodies within the CNS. Five of these deep-lying masses of gray matter are located within the white matter of each hemisphere and are collectively known as the basal ganglia. These masses are the caudate nucleus, the putamen, the globus pallidus, the substantia nigra, and the subthalamic nucleus. Together, they exert a steadying influence on muscle activity. The basal ganglia are an important part of the extrapyramidal motor pathway that connects nuclei with each other, with the cortex, and with the spinal cord. The ganglia also connect with areas in the hindbrain (the red nucleus and the substantia nigra) to assist in the role of smoothing and coordinating muscle movements. Disturbances in these ganglia result in tremor, rigidity, and loss of expressive and walking movements, as seen in Parkinson syndrome.5
Diencephalon
The second major division of the forebrain is the diencephalon (Fig. 10-3), which consists of the thalamus and the hypothalamus. The diencephalon also contains the third ventricle and is almost completely covered by the cerebral hemispheres. This portion of the brain has a primary role in sleep, emotion, thermoregulation, autonomic activity, and endocrine control of ongoing behavioral patterns.
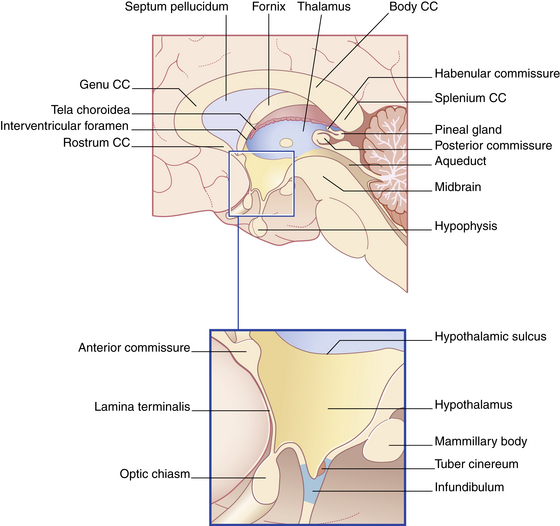
FIG. 10-3 The diencephalon and its boundaries. CC, Corpus callosum.
(From Fitzgerald MJT, et al: Clinical neuroanatomy and neuroscience, ed 6, St. Louis, 2011, Saunders.)
The hypothalamus is a group of bilateral nuclei that forms the floor and part of the lateral walls of the third ventricle. Extremely complex in function, the hypothalamus has extensive connections with the autonomic nervous system and with other parts of the CNS. It also influences the endocrine system by virtue of direct and indirect connections with the pituitary gland and the release of its own hormones. In association with these other structures, the hypothalamus participates in the regulation of appetite, water balance, carbohydrate and fat metabolism, growth, sexual maturity, body temperature, pulse rate, blood pressure, sleep, and aspects of emotional behavior. Because of the connection of the hypothalamus with the thalamus and cerebral cortex, emotions can influence visceral responses on certain occasions.6
Midbrain
The midbrain, or mesencephalon, is a short narrow segment of nervous tissue that connects the forebrain with the hindbrain. The midbrain is vital as a conduction pathway and as a reflex control center. Passing through the center of the midbrain is the cerebral aqueduct, a narrow canal that serves to connect the third ventricle of the diencephalon with the fourth ventricle of the hindbrain for the circulation of CSF. In addition, cranial nerves III (oculomotor) and IV (trochlear) originate in the ventral aspect of the midbrain.7
Hindbrain
The hindbrain, or rhombencephalon, consists of the pons, the medulla oblongata, the cerebellum, and the fourth ventricle (Fig. 10-4).
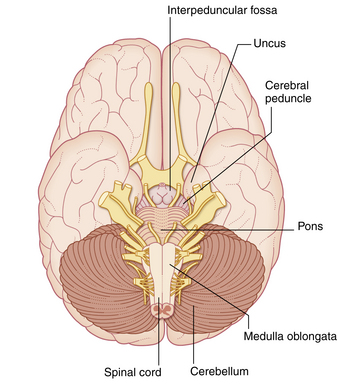
FIG. 10-4 Ventral view of the brainstem in situ.
(From Fitzgerald MJT, et al: Clinical neuroanatomy and neuroscience, ed 6, St. Louis, 2011, Saunders.)
Medulla oblongata
The medulla oblongata is an expanded continuation of the spinal cord and is located between the base of the skull, the foramen magnum, and the pons. It is anatomically complex and not usually amenable to surgery. Many of the white fiber tracts between the brain and spinal cord decussate as they pass through the medulla. Centers for many complex reflexes are located in the medulla oblongata and include those for swallowing, vomiting, coughing, and sneezing. The originating nuclei of cranial nerves IX (glossopharyngeal), X (vagus), XI (accessory), and XII (hypoglossal) are found in the medulla oblongata (Table 10-1). Because of these originating nuclei, the medulla has an essential role in the regulation of cardiac, respiratory, and vasomotor reflexes. Injuries to the medulla, such as those that accompany basal skull fracture, often prove fatal.
Cerebellum
The cerebellum has no sensory function and does not initiate movement as the cerebrum does. Functionally, it coordinates muscle tone and voluntary movements through important connections via the spinal cord with the proprioceptor nerve fibers in skeletal muscles, tendons, and joints. In addition, the cerebellum is involved in reflexes necessary for the maintenance of equilibrium and posture, through its connections with the vestibular apparatus of the inner ear. The cerebellum also receives optic and acoustic information, but the specifics of the anatomic pathways involved have not yet been discerned.
Brainstem
Authors disagree to some extent as to what structures collectively constitute the brainstem. All agree that it includes the midbrain, the pons, and the medulla oblongata. Some believe that the diencephalon rightly belongs in the group. Whichever grouping is used, all functions of each structure within it may be considered to be basic activities of the brainstem. All the cranial nerves are attached to the brainstem (if the diencephalon is included), with the exception of the olfactory nerve and the spinal portion of the accessory nerve.
Protection of the brain
The brain is protected by the cranial bones, the meninges, and the CSF (Figs. 10-5 and 10-6).
Cranial bones
Eight cranial bones encase the brain and support and protect it from most ordinary bumps and jarring. In the adult, immovable fibrous joints, or sutures, fuse these bones together to form the rigid walls of the box known as the cranium. The base of the cranium is both thicker and stronger than its roof or walls.
One main opening is located at the base of the skull and is called the foramen magnum. It marks the point at which the brainstem changes structure and becomes identified inferiorly as the spinal cord. Many smaller openings in the skull allow the cranial nerves and some blood vessels to pass through it to and from the face, the jaw, and the neck. The atlas of the vertebral column (C1) supports the skull and forms a moveable joint with the occipital bone.7
Meninges
The meninges (Fig. 10-7) are three fibrous membranes between the skull and the brain and between the vertebral column and the spinal cord. The outer membrane is the dura mater and the inner is the pia mater; between them lies the arachnoid mater.
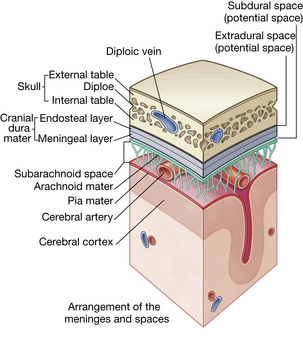
FIG. 10-7 Arrangement of meninges in the cranial cavity.
(From Drake RL, et al: Gray’s anatomy for students, ed 2, Philadelphia, 2010, Churchill Livingstone.)
Dura mater.
The dura mater is a shiny, tough, inelastic membrane that envelops and supports the brain and spinal cord and, by various folds, separates parts of the brain into adjoining compartments. The portion within the skull differs from the dura of the spinal cord in three ways. First, the cranial dura is firmly attached to the skull. The spinal dura has no attachment to the vertebrae. Second, the cranial dura consists of two layers; it covers the brain (meningeal dura) and lines the interior of the skull bones (periosteal dura). Third, the two layers of the cranial dura are in contact with each other in some places but separate in others where the inner layer dips inward to form the protective partitions between parts of the brain. In addition, the spaces or channels formed by these separations of dural layers are filled with venous blood that is leaving the brain; these spaces are called cranial venous sinuses and are an elaborate network unique to the brain (see Fig. 10-7).
Encased between the two dural layers are two major groups of venous channels that drain blood from the brain. None of these vascular channels possesses valves, and their walls are extremely thin because of the absence of muscular tissue. The superior-posterior group consists of one paired and four unpaired sinuses. The anterior-inferior group consists of four paired sinuses and one plexus. The sinuses function to drain venous blood into the internal jugular veins, which are the principal vessels responsible for the return of the blood from the brain to the heart (Fig. 10-8).
Arachnoid mater.
The arachnoid forms a type of roof over the pia mater, to which it is joined by a network of trabeculae in the subarachnoid space. It does not follow the depressions of the surface architecture. The arachnoid sends small, tuftlike extensions through the meningeal layer of the dura into the cranial venous sinuses. These extensions are called the arachnoid granulations or arachnoid villi. The arachnoid villi serve as a pathway for the return of CSF to the venous blood system. Subarachnoid CSF is most abundant in the grooves between the gyri, particularly at the base of the brain, where the more freely communicating compartments form six subarachnoid cisternae, or reservoirs.
Pia mater.
The inner layer of the meninges, the pia mater, is a fine membrane rich in blood (choroid) plexuses and mesothelial cells. This layer is closely associated with the arachnoid and covers the brain intimately, following the invaginations and convolutions of the brain surface. The veins of the brain lie between threadlike trabeculae in the subarachnoid space. Branches of the cortical arteries in the subarachnoid space are carried with the pia mater and enter the brain substance itself.5
Cerebrospinal fluid system
Cerebrospinal fluid is found within the ventricles of the brain, in the cisterns that surround it, and in the subarachnoid spaces of both the brain and the spinal cord (Figs. 10-9 and 10-10). The largest of the cisterns is the cisterna magna, which is located beneath and behind the cerebellum.
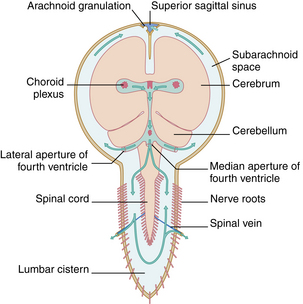
FIG. 10-9 Circulation of cerebrospinal fluid.
(From Fitzgerald MJT, et al: Clinical neuroanatomy and neuroscience, ed 6, St. Louis, 2011, Saunders.)
The main route of reabsorption of excess CSF is through the arachnoid villi that project from the subarachnoid spaces into the venous sinuses of the brain, particularly those of the superior sagittal sinus. The arachnoid villi provide highly permeable regions that allow free passage of CSF, including protein molecules and some small particulate matter contained within it. The process of osmosis is believed to be mainly responsible for the reabsorption of the fluid.6
Blood-brain barriers of the central nervous system
On the other extreme of the spectrum is brain tissue. Brain tissue is highly sensitive to changes in its extracellular environment and the introduction of foreign or unusual substances. To facilitate the maintenance of this uniquely balanced environment, the body has evolved a blood-brain barrier to tightly regulate what may enter the extracellular environment of the brain from the capillaries.
The speed with which substances penetrate the blood-brain barrier is inversely proportional to their molecular size and directly proportional to their lipid solubility. Only water, carbon dioxide, and oxygen cross the blood-brain barrier rapidly and readily, whereas glucose crosses more slowly and by a facilitated transport mechanism. Water-soluble compounds, electrolytes, and large protein molecules generally cross very slowly or not at all. Most general anesthetics effectively cross the blood-brain barrier because of their high lipid solubility.8,9
Arterial blood supply to the brain
The entire arterial blood supply to the brain, with the exception of a small amount that flows in the anterior spinal artery to the medulla, is carried through the neck by four vessels: the two vertebral arteries and the two carotid arteries (Figs. 10-11 and 10-12).
The two vertebral arteries supply the posterior portion of the brain. They ascend in the neck through the transverse foramina on each side of the cervical vertebrae, enter the skull through the foramen magnum, and join near the pons to form the basilar artery of the hindbrain. A relatively small volume of the total blood flow to the brain is carried by the vertebral or basilar artery. The circle of Willis, in turn, is formed by the union of the basilar artery and the two internal carotid arteries. Before they join the circle of Willis, these arteries send essential branches to the brainstem, cerebellum, and falx cerebelli.
The circle of Willis is a ring of blood vessels that surrounds the optic chiasm and the pituitary stalk. The circle of Willis gives rise to three pairs of arteries: the anterior, the middle, and the posterior cerebral arteries. Each pair of arteries supplies specific areas of the brain: (1) the anterior cerebral arteries supply approximately half of the frontal and parietal lobes, including much of the corpus callosum; (2) the middle cerebral arteries perfuse most of the lateral surfaces of the hemispheres and send off branches to the corpus striatum and the internal capsule; and (3) the posterior cerebral arteries supply the occipital lobes and the remaining portions of the temporal lobes that are not supplied by the middle cerebral arteries.7
Regulation of cerebral blood flow
The body has several mechanisms to ensure the uninterrupted high rate of blood flow required by the brain. As in other tissues in the body, the brain’s requirement varies with metabolic activity. The more active the brain, the greater the blood supply it requires. Regulation of this blood supply is primarily based upon the concentration of carbon dioxide in the brain tissue. Carbon dioxide is a normal byproduct of neuronal cellular metabolism. The concentration of carbon dioxide in the brain tissue increases as neuronal cellular metabolism increases with an increase in brain activity. This increase in carbon dioxide causes the cerebral vasculature to dilate, increasing blood flow to the brain. The opposite occurs when carbon dioxide concentration declines in the brain. This mechanism is highly sensitive, and even small changes from the normal carbon dioxide levels in the brain can cause significant changes in the volume of cerebral blood flow.
Intracranial pressure dynamics
The bones of the skull form a rigid box that surrounds and contains the brain, CSF, and blood that is perfusing the brain. Intracranial pressure (ICP) is the pressure created within the skull by the contents; it usually ranges from 4 to 15 mm Hg. Compensatory mechanisms exist that control for minor variations in the changes of the volumes of blood, CSF, and brain tissue within the skull, keeping the ICP nearly constant. If ICP should increase because of an increase in one or more of the contents of the skull, such as a brain tumor or swelling because of a blow to the head, significant injury can result to the brain from lack of blood flow or death owing to herniation of the brain through the base of the skull.
Cerebral perfusion pressure
From this formula, it is obvious that an increase in ICP or a decrease in MAP can compromise CPP and blood flow to the brain. This situation is a common concern for neurosurgical patients during the perioperative period and a critical concern for PACU nurses. Efforts must be simultaneously made to maintain mean arterial pressure while at the same time control the intracranial pressure. Common steps to control intracranial pressure include decreasing brain activity by administering sedative drugs, controlling blood flow to the brain by decreasing carbon dioxide concentration in the brain by hyperventilating the patient, and maintaining a patient in a semi-Fowler’s position to optimize venous drainage from the brain. Care must always be taken, though, not to sacrifice mean arterial pressure in an attempt to decrease intracranial pressure, as can happen with the overzealous use of diuretics.5,10
Spinal cord
Protection of the spinal cord
Bones of the spine
Between each of the vertebrae and atop the sacrum is an intervertebral disk composed of compressible tough fibrous cartilage concentrically arranged around a soft pulpy substance called the nucleus pulposus. Each disk acts as a cushionlike shock absorber between the vertebrae. When the intervertebral disk is ruptured, the soft nucleus pulposus can protrude into the vertebral canal, where it can exert pressure on a spinal nerve root and can cause significant, debilitating pain and motor function impairment. This herniated nucleus pulposus may require surgical excision through a laminectomy if the herniation is severe enough.
Many important variations exist among the regional vertebrae. For example, the first cervical vertebra, or atlas, is ring shaped and supports the cranium. It has no body or spinous process and allows for a nodding motion of the head. The second cervical vertebra, or axis, is most striking because of the odontoid process, or dens, that arises perpendicularly to meet with the atlas and allows rotation of the head. The cervical spine as a whole is extremely mobile and is therefore particularly susceptible to acceleration-deceleration and twisting injuries that hyperflex or hyperextend the neck. In addition, the spinal cord is relatively large in this area and therefore sustains damage fairly easily after injury to the cervical spine (Fig. 10-13).
The five lumbar vertebrae are large and massive because of their prominent role in weight bearing. They have no transverse foramina. The sacrum, with its five fused vertebrae, is large, triangular, and wedge-shaped. It forms the posterior wall of the pelvis and articulates with L5, the coccyx, and the iliac portions of the hips. The triangular coccyx is formed by four small segments of bone, the most rudimentary part of the vertebral column.1
Spinal meninges
The epidural space is located between the outer surface of the dura and the bones and ligaments of the vertebral canal. It contains a quantity of loose connective tissue, fat, and a plexus of veins. The subdural space is a potential space that lies below the inner surface of the dura and the arachnoid membrane; it contains only a limited amount of CSF.
The third and innermost meningeal layer of the spine is the delicate pia mater. Although it is continuous with the cranial pia mater, it is less vascular, thicker, and denser in structure than the pia mater of the brain. The pia mater intimately invests the entire surface of the cord, and, at the point where the cord terminates, it contracts and continues down as a long slender filament (filum terminale) through the center of the bundle of nerves of the cauda equina and anchors the cord at the base of the coccyx.11
Lumbar puncture
The examination of CSF and determination of CSF pressure are frequently of great value in the diagnosis of neurologic and neurosurgical conditions. The collection of CSF is ordinarily accomplished through the insertion of a long spinal needle between the open spaces between L3 and L4 or L4 and L5, through the dura and arachnoid into the subarachnoid space. Because the spinal cord in adults ends at the level of the disk between L1 and L2, danger of injuring the cord with this procedure is minimal. In children, the spinal cord may extend below L3 so that the subarachnoid space is usually safely entered in the areas between L4 and L5. In both adults and children, flexion of the spine by assuming the fetal position raises the cord superiorly somewhat farther, thus further decreasing the risk of damage to the cord. Because the most superior points of the iliac crests are at the level of the upper border of the spine of L4, they are used as anatomic reference points in selection of the site for lumbar puncture. For a complete description of spinal and epidural anesthesia, see Chapter 25.12,13
Structure and function of the spinal cord and the spinal nerve roots
From that point, the cord continues downward to the upper level of the body of L2, where it narrows to a sharp tip called the conus medullaris. From the end of the conus, an extension of the pia mater known as the filum terminale continues to the first segment of the coccyx, where it attaches (Fig. 10-14).
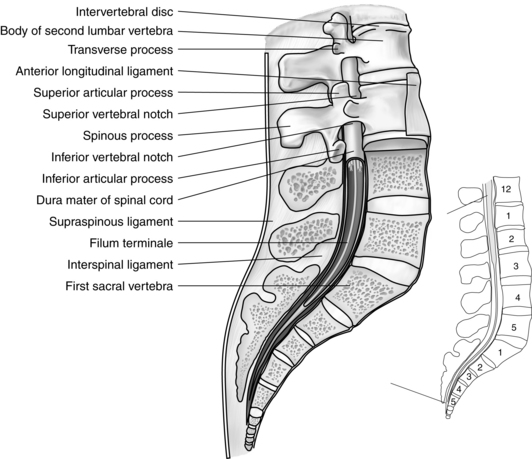
FIG. 10-14 Vertebral column showing structure of vertebrae, filum terminale, and termination of dura mater.
The spinal cord (Fig. 10-15) is composed of 31 horizontal segments of varying lengths. It comprises 8 cervical, 12 thoracic, 5 lumbar, 5 sacral, and 1 coccygeal segment, each with a corresponding pair of spinal nerves attached.
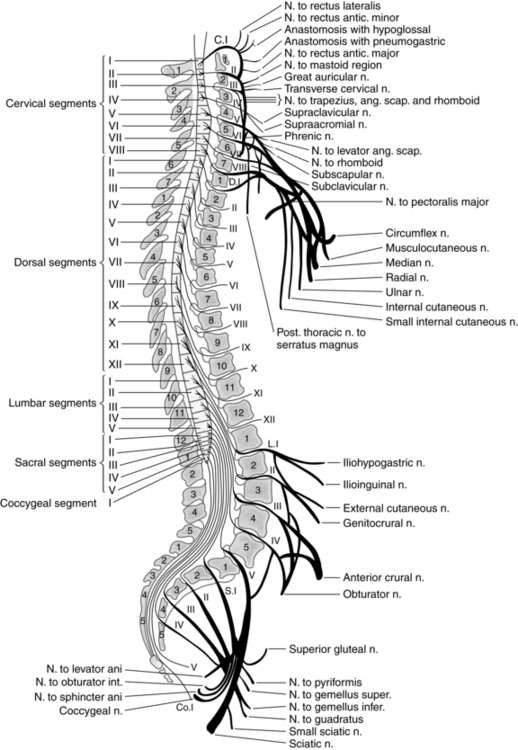
FIG. 10-15 Relationship of segments of spinal cord and their nerve roots to bodies and spinous processes of vertebrae.
During the growth of the fetus and young child, the spinal cord does not continue to lengthen as the vertebral column lengthens. Consequently, the cord segments, from which spinal nerves originate, are displaced upward from their corresponding vertebrae. This discrepancy becomes greater with each downward segment. For example, the cervical and thoracic nerve roots take an almost horizontal course as they leave the spinal cord and emerge through the intervertebral foramina. The lumbar and sacral nerve roots, however, are extremely long and take an oblique downward course before finally emerging from their appropriate lumbar or sacral intervertebral foramina. The large bundle of nerves lying within the inferior vertebral canal is called the cauda equina for its resemblance to a horse’s tail (see Fig. 10-15). Several longitudinal grooves divide the spinal cord into regions. The deepest of these grooves is the anterior median fissure. Opposite this, on the posterior surface of the cord, is the posterior median fissure. These fissures divide the cord into symmetric right and left halves that are joined in the central midportion (Fig. 10-16).
When viewed in cross section, the gray matter of the cord looks like the letter H, two crescent-shaped halves joined together by the gray commissure surrounded by white matter. For descriptive purposes, the four segments of the H are called right and left anterior (ventral) and posterior (dorsal) horns. The anterior motor (efferent) neurons lie within the anterior (ventral) gray horns and send fibers through the spinal nerves to the skeletal muscle. The nerve cell bodies that compose the posterior (dorsal) gray horns receive sensory (afferent) signals from the periphery via the spinal nerve roots. The lateral gray horns project from the intermediate portion of the H. The nerve cells in these horns (called preganglionic autonomic neurons) give rise to fibers that lead to the autonomic nervous system.
The 31 pairs of spinal nerves are symmetrically arranged. Each nerve contains several types of fibers and arises from the spinal cord by two roots: a posterior (dorsal) and an anterior (ventral) root (Fig. 10-17). The axons that make up the fibers in the anterior roots originate from the cell bodies and dendrites in the anterior and lateral gray horns. The anterior (ventral) root is the motor root, which conveys impulses from the CNS to the skeletal muscles. The posterior (dorsal) root is known as the sensory root. Sensory fibers originate in the posterior root ganglia of the spinal nerves. Each ganglion is an oval enlargement of the root that lies just outside the intervertebral foramen and contains the accumulated cell bodies of the axons that compose the sensory fibers. One branch of the ganglion extends into the posterior gray horn of the cord. The other branch is distributed to both visceral and somatic organs and mediates afferent impulses to the CNS. The cutaneous (skin) area innervated by a single posterior root is called a dermatome. Knowledge of dermatome levels is useful clinically in determination of the level of anesthesia after spinal or regional anesthesia (see Chapter 25).1
Anterior rami from the thoracic area do not form a plexus but lead instead to the skin of the thorax and to the intercostal muscles directly. The thoracic and upper lumbar spinal nerves also give rise to white rami (visceral efferent branches), or preganglionic autonomic nerve fibers. Parts of this ramus join the spinal nerves to the sympathetic trunk. The gray ramus is present in all spinal nerves.14
Vascular network of the spinal cord
The venous supply inside and outside the entire length of the vertebral canal is derived from a series of venous plexuses (Fig. 10-18) that anastomose with each other and end in intervertebral veins. The intervertebral veins leave the cord through the intervertebral foramina with the spinal nerves.
Autonomic nervous system
Sympathetic nervous system
The sympathetic nervous system originates from the thoracolumbar (T1 to L2) segments of the spinal cord. This system is mainly excitatory in physiologic function; however, research indicates that some inhibitory function in the sympathetic nervous system does exist. Because the sympathetic nervous system involves the cardiovascular system and cardiovascular drugs, it is discussed in detail in Chapter 11.
Parasympathetic nervous system
The parasympathetic nervous system basically functions as an inhibitor of the sympathetic nervous system. It originates in the cranium via cranial nerves III, V, VII, IX, and X. Cranial nerve X, or the vagus nerve, is the most important nerve because it carries about 75% of the parasympathetic nerve impulses. The parasympathetic nervous system also originates in the sacral portion of the spinal cord. Consequently, the parasympathetic nervous system uses the craniosacral outflow tracts.15 Acetylcholine (ACh) is the main neurotransmitter for the parasympathetic nervous system. The ACh acts on two types of receptors, the muscarinic and nicotinic cholinergic receptors. Because of the pharmacologic implications of the parasympathetic nervous system, it is discussed in detail in Chapters 11 and 23.
Summary
This chapter demonstrates the physiologic concept of regulation and control. The nervous system is highly complex, with both central and peripheral components working in concert. It has electric and chemical neurotransmitters that in many ways functions best in a homeostatic environment. Should the body suffer injury or change in fluid status or temperature, to name a few examples, the nervous system does not function appropriately. Consequently, evaluation of the nervous system by the perianesthesia nurse is an important component of nursing care in the PACU.
1. Hanson JT. Netter’s clinical anatomy, ed 2. Philadelphia: Saunders; 2010.
2. Benumof J, Saidman L. Anesthesia and perioperative complications, ed 2. St. Louis: Mosby; 1999.
3. Evers A, Maze M. Anesthetic pharmacology: physiologic principles and clinical practice. ed 2. Philadelphia: Churchill Livingstone; 2011.
4. Cottrell J, Smith D. Anesthesia and neurosurgery, ed 4. St. Louis: Mosby; 2001.
5. Hall J. Guyton and Hall textbook of medical physiology, ed 12. St. Louis: Saunders; 2010.
6. Fitzgerald MJT, et al. Clinical neuroanatomy and neuroscience, ed 6. St. Louis: Saunders; 2012.
7. Drake R, et al. Gray’s anatomy for students, ed 2. Philadelphia: Churchill Livingstone; 2009.
8. Brunton L, et al. Goodman and Gilman’s the pharmacological basis of therapeutics, ed 12. New York: McGraw-Hill; 2010.
9. Stoelting R. Pharmacology and physiology in anesthetic practice, ed 4. Philadelphia: Lippincott Williams & Wilkins; 2005.
10. Ganong W. Review of medical physiology, ed 23. New York: McGraw-Hill; 2009.
11. Miller R. Anesthesia, ed 7. St. Louis: Saunders; 2009.
12. Barash P, et al. Clinical anesthesia, ed 6. Philadelphia: Lippincott Williams & Wilkins; 2009.
13. Nagelhout J, Plaus K. Nurse anesthesia, ed 4. St. Louis: Saunders; 2010.
14. Miller RD, Pardo M. Basics of anesthesia, ed 6. St. Louis: Saunders; 2011.
15. Aitkenhead A, et al. Textbook of anaesthesia, ed 5. London: Churchill Livingstone; 2007.