CHAPTER 6 The Intervertebral Disc
Normal, Aging, and Pathologic
The intervertebral disc is a fibrocartilaginous structure whose principal function is to act as a shock absorber, transmitting compressive loads between vertebral bodies. Degeneration of the disc is associated with several clinical conditions, including herniation of the nucleus pulposus, mechanical back pain, spinal stenosis, and other spinal deformities such as scoliosis. The human intervertebral disc is considered to undergo more dramatic degenerative changes than any other musculoskeletal tissue in the body1 and to undergo these changes at an earlier age.2
Normal Disc
Disc Anatomy
The intervertebral disc is composed of three main structures: the cartilaginous endplates, the central nucleus pulposus, and the peripherally located anulus fibrosus (Fig. 6–1).
Cartilaginous Endplates
The intervertebral disc is separated from adjacent vertebral bodies by a cartilaginous endplate superiorly and inferiorly. In humans, the endplate serves as the growth plate for the vertebral bodies, having the typical structure of an epiphyseal growth plate.3 In infancy, this growth plate is thick and occupies a substantial fraction of the disc. The endplates thin as growth progresses and eventually consist of only a 1-mm-thick, avascular layer of hyaline cartilage in adults.3 Similar to hyaline cartilage elsewhere in the body, the cartilaginous endplates are composed of rounded chondrocytes.4 Biomechanically, most compressive forces are transmitted through the superior vertebral body to the endplate, to the nucleus pulposus, and to the inferior endplate and vertebral body. The endplates and adjacent trabecular bone can undergo temporary deformation when a load is applied.
Nucleus Pulposus
The nucleus lies between adjacent endplates and forms the gel-like core of the disc. The nucleus consists of a proteoglycan and water matrix held together by an irregular network of collagen type II and elastin fibers. Proteoglycans have numerous highly anionic glycosaminoglycan (GAG) side chains (i.e., chondroitan sulfate and keratan sulfate), which allows the nucleus to imbibe water. This composition is similar to articular cartilage, and the ability of the matrix to imbibe and release water in relation to applied stresses allows the disc to cushion against compressive loads. The primary proteoglycan is aggrecan, and the high concentration of this hydrophilic molecule provides the osmotic properties needed to resist compression.5
Cells in the nucleus are initially notochordal, but their number declines after birth and they eventually become undetectable at about age 4 to 10 years.6 The nucleus is gradually replaced during growth by rounded cells resembling the chondrocytes of articular cartilage.7 These chondrocyte-like cells synthesize mostly proteoglycans and collagen type II in response to changes in hydrostatic pressure. The nucleus functions as a shock absorber, acting as a pressurized, deformable sphere that dissipates compressive forces to the anulus and the adjacent vertebral bodies. As compressive forces on the spine increase, hydrostatic pressure within the nucleus pushes outward from its center in all directions.
Anulus Fibrosus
The anulus fibrosus surrounds the nucleus and is composed of approximately 20 concentric rings (lamellae) of highly organized collagen fibers, primarily collagen type I. The collagen fibers are orientated approximately 60 degrees to the vertical axis of the spine and run parallel within each lamella but perpendicular between adjacent lamellae allowing for maximal tensile strength.8 Fibers of the outer anulus attach to the periphery of the vertebral bodies, whereas inner fibers pass from one endplate to another. Cells in the anulus are found between lamellae, arranged in parallel to the collagen fibers. Outer anulus cells are thin and elongated and phenotypically similar to fibroblasts, whereas cells of the innermost anulus are more spheroid similar to articular chondrocytes.1,9 The anulus contains the nucleus pulposus and maintains its pressurization under compressive loads. The tensile properties of the anulus allow the nucleus to recover its original shape and position when the compressive load is reduced.
Blood Supply, Nutrition, and Innervation
Blood Supply
In early fetal life, vascular channels traverse the endplates, but they diminish in size starting at birth until complete disappearance by approximately 5 years of age. In adults, the blood supply of the disc arises from two capillary plexuses. One plexus penetrates 1 to 2 mm into the outer anulus, supplying only the periphery of the anulus. The other capillary plexus begins in the vertebral body and penetrates the subchondral bone (see Fig. 6–1), terminating in capillary loops at the bone-cartilage junction.10 The density of this capillary network varies in location across the endplate, being greatest in the center and lowest at the periphery. Cells in the center of the adult nucleus pulposus are 8 mm from the nearest blood source, making the disc one of the largest avascular structures in the body.
Nutrition
The limited vascularity of the intervertebral disc has important physiologic implications—mainly that nutrition depends almost entirely on diffusion (Fig. 6–2).11–13 The nutritional environment of the cells varies throughout the disc because of its size; cells in the nucleus are 6 to 8 mm from the nearest blood vessel. Small molecules necessary to maintain cellular function (i.e., glucose and oxygen) readily leave vertebral capillaries and diffuse across the thin cartilaginous endplate and the outermost layers of the anulus into the ECM. Concentration gradients of glucose, oxygen, and other nutrients and metabolites exist across the disc, regulated by the rates of nutrient supply and consumption. The low oxygen tension in the nucleus leads to anaerobic metabolism (i.e., glycolysis), resulting in a high concentration of lactic acid and a lower pH in the nucleus compared with the periphery of the disc.13 Metabolic by-products such as lactic acid are removed from the disc by diffusion in the opposite direction of nutrient entry.
Disc Composition
The function of the intervertebral disc depends greatly on the properties of the extracellular matrix (ECM). The ECM provides the biomechanical properties and acts as a filter to regulate the extracellular fluid composition and the rate at which nutrients and metabolites are exchanged. The ECM consists of a complex network of macromolecules whose composition varies in different regions of the disc (Fig. 6–3).4,14 ECM macromolecules are synthesized and maintained by a small population of cells (9000 cells/mm3 in the anulus and 5000 cells/mm3 in the nucleus) occupying less than 1% of the disc volume.4 Disc cells also produce a complex array of cytokines, growth factors, and proteases to maintain equilibrium between the rates of synthesis and degradation of ECM components.15,16
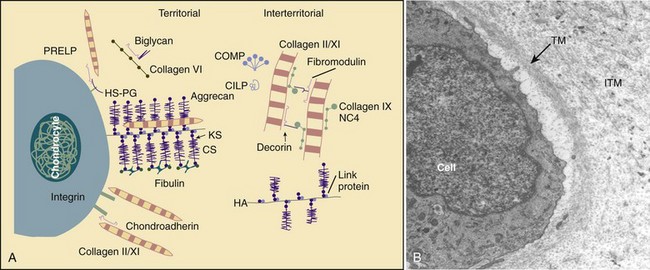
(A, Adapted from Heinegard D, Aspberg A, Morgelin M, et al: Extracellular matrix of cartilage. Section for Connective Tissue Biology, University of Lund, 2003. Available at http://www.cmb.lu.se/ctb.)
Water
The major component of the intervertebral disc is water, and its concentration is regulated by the GAG side chains of proteoglycans. The concentration of water varies with age, location within the disc, and body position.17 The nucleus pulposus is most highly hydrated, and the water concentration may be 90% in an infant, declining to approximately 80% in nondegenerated young adult discs.18 The water content of the anulus is lower than the nucleus, declining to 65% in the outer anulus in adult discs.
Water content varies with load, leading to diurnal changes in disc hydration.19 During the diurnal cycle in young, highly hydrated lumbar discs, 25% of the disc’s water can be lost and regained.20 Water is expressed from the disc during the day because of the increased forces of body weight and muscle contractions, and it is reimbibed at night when the compressive forces are removed. This diurnal cycle results in changes in disc height and affects the disc’s mechanical properties.
Macromolecules
Collagen is one major macromolecular component of the disc. The collagen content of the disc is highest in the outer anulus, and the dry weight decreases significantly in the nucleus of adult discs.21 The concentration of collagen type I is highest in the outer anulus and decreases toward the nucleus, where virtually none is present.21 Collagen type II follows the opposite gradient, with the highest concentration located in the nucleus. Along with collagen types I and II, the ECM contains many other collagens, including types III, V, VI, IX, and XI.
The other major macromolecule of the disc is aggrecan,22 which consists of a protein core with approximately 100 anionic GAG side chains. Many aggrecan molecules covalently attach to hyaluronan chains forming large aggregates. These aggregates are trapped by the surrounding collagen network, imparting a net negative charge to the ECM. The interstitial water contains an excess of cations, which is directly related to the concentration of negative charge (i.e., GAG concentration). The high concentration of cations imparts a high osmotic pressure in the nucleus, which consequently leads to imbibition of water. Changes in proteoglycan concentration and GAG concentration lead to changes in osmotic pressure, affecting the ability of the disc to maintain hydration and turgor when loaded.23
In addition to collagens and aggrecan, the disc contains lower concentrations of numerous other macromolecules,14 including elastin, the smaller proteoglycans decorin and fibromodulin, cartilage oligomeric matrix protein, and cartilage intermediate layer protein. These molecules function either structurally or biomechanically and are important for normal disc function.
Intervertebral Disc: Aging and Degeneration
Aging
Human intervertebral discs undergo very early aging and degeneration, resulting in histomorphologic and functional changes (Fig. 6–4).24 Endplate permeability and vascular supply decrease throughout growth and aging, leading to altered metabolite transport.24 Proteoglycans begin to fragment during childhood, and the overall proteoglycan content decreases with age, especially in the nucleus. There is a corresponding increase in collagen content, with collagen type I fibers replacing collagen type II fibers in the inner anulus and nucleus. In addition, reduced matrix turnover in older discs enables collagen fibrils to become increasingly cross-linked,25 leading to retention of damaged fibers and reduced tissue strength. Synthesis of ECM components decreases steadily throughout life, and this is partly attributable to decreased cell density, although synthesis rates per cell also decrease.
In infants, the nucleus contains approximately 90% water and appears translucent.18 The disc dehydrates slowly with aging, with water content of the nucleus declining to around 80% in young adults.24 The nucleus also accumulates yellow pigmentation and becomes less distinguishable from the surrounding anulus.18,24 As the disc water content decreases, the nucleus becomes smaller and decompressed, often condensing into several fibrous lumps. Dehydration of the nucleus leads to altered biomechanical properties of the disc, forcing the anulus to act as a fibrous solid to resist compression directly. The proteoglycan content of the anulus also decreases with aging, and the anulus becomes stiffer and weaker, resisting compressive loads in a haphazard manner.
Degeneration
Intervertebral disc degeneration mimics age-related changes of the disc, but the process occurs prematurely or at an accelerated rate26,27 and usually results in symptoms. There are no widely accepted definitions of disc degeneration in the literature, reflecting the difficulty in distinguishing degeneration from the physiologic processes of growth, aging, and remodeling. More recent definitions describe degeneration as an aberrant, cell-mediated response to progressive damage, with combined structural failure and accelerated or advanced signs of aging. These proposed definitions also suggest that structurally intact discs with accelerated age-related changes be classified as early degenerative discs, whereas the term degenerative disc disease should be applied if the disc is also painful.26
Matrix Macromolecule Changes
The most physiologically important changes of disc degeneration start in the nucleus.18 Early changes include increased degradation of aggrecan and other aggregating proteoglycans coupled with an increased concentration of nonaggregating proteoglycans. The accumulation of degraded proteoglycans further impairs diffusion of nutrients and oxygen through the disc. A change in the proportions of the GAGs chondroitan sulfate, heparan sulfate, and keratan sulfate also occurs, with increasing amounts of heparan sulfate and keratan sulfate as degeneration progresses. These changes diminish the hydroscopic properties of the ECM further, resulting in decreased water content and decreased ability to imbibe water. Loss of proteoglycans and GAGs leads to decreased swelling pressure,23 loss of hydration, and loss of disc height. The changes result in altered responses to applied biomechanical loads, ultimately leading to the structural features of degeneration.
Intervertebral disc degeneration also results in disorganization and destruction of the collagen network.28 As the overall proteoglycan and water content decreases, there is a corresponding increase in collagen content. Collagen type I replaces collagen type II in the inner anulus and nucleus, and there is a tendency for collagen type I fibrils throughout the disc to become coarser. The highly organized collagen fiber arrangements of the anulus are also disrupted, and collagen and elastin networks become more haphazard. When the collagen network has been damaged, disc biomechanics are markedly altered, and the potential for structural damage increases.
The overall ECM content in the nucleus is a well-controlled equilibrium between degradative and synthetic pathways involving numerous proteins. In disc degeneration, there is an imbalance between degradative and synthetic pathways and a predominance of catabolic enzyme activity. Proteinases of the matrix metalloproteinase (MMP) and ADAMTS families cleave collagens and other macromolecules and have been implicated in the breakdown of the ECM.29 The degradative enzymes MMP-3 and MMP-13 (also known as stromelysin-1 [MMP-3] and collagenase 3 [MMP-13]) have been found at increased levels in degenerated human discs.
The regulation of MMP and ADAMTS production and ECM macromolecule production is achieved by numerous cytokines and growth factors. Of particular importance in disc ECM homeostasis are members of the interleukin (IL) family (catabolism) and transforming growth factor-β (anabolism) superfamily.30,31 Mediators of inflammation such as nitric oxide and prostaglandin E2 and the cytokines IL-1 and IL-6 are found at increased levels in degenerated discs.30–32 The synthetic capabilities of nucleus cells are unable to sustain appropriate levels of aggrecan and collagen production in the face of this increased catabolism, which contributes to further degeneration of the disc.
Cellular Changes
It has long been recognized that there is a slowly progressive loss of cells during disc degeneration,33 leading to further loss of the ECM. An increasing body of literature has shown that apoptosis, or programmed cell death, may be responsible for many of the features of degeneration.34–36 More recent literature has also shown an increase in lacunae containing cell clusters,37,38 possibly causing an overall increased number of cells as disc degeneration progresses. This increased cell proliferation may be an attempt to offset the progressive destruction and loss of the ECM. One reason for increased cellularity may be the focal increase in nutrient supply owing to the ingrowth of blood vessels in degenerating discs, as discussed elsewhere in this chapter.
Structural Changes
As disc hydration decreases, the distinction between anulus and nucleus becomes less defined and disc height decreases (Fig. 6–5).24 In later stages, gross tissue changes become increasingly apparent, including loss of lamellae organization, fissuring of the anulus,39 and discoloration and solidification of the nucleus.40,41 Radial and circumferential annular tears are often evident, sometimes extending to the disc periphery.39 These changes are accompanied by ingrowth of nerves and blood vessels into the disc and deposition of granulation tissue and calcification within the endplates. These structural changes ultimately lead to altered, abnormal biomechanical properties of the disc. Damage to one area of the disc increases load bearing by adjacent tissues, making it more likely for damage to spread. Although a healthy intervertebral disc equalizes pressure within it, the decreased shock-absorbing capacity of the decompressed nucleus leads to high compressive stresses in the anulus.42 Other gross morphologic changes of degeneration include disc bulging, disc space narrowing, endplate irregularities, and osteophyte formation and arthritis.
Neovascularization and Ingrowth of Sensory Nerves
As stated previously, the disc is largely avascular in adults with blood vessels normally restricted to only the outermost layers of the anulus. Likewise, only the outer 1 to 2 mm of the anulus is innervated in the normal human disc. The ingrowth of blood vessels and sensory nerves is an important feature of degenerated discs, however, and seems to be associated with pain.43 Ingrowth of capillaries is facilitated by the loss of hydrostatic pressure in the inner regions of the disc, which would normally collapse small vessels. These newly formed microvessels release neurotrophic growth factors such as nerve growth factor, allowing the ingrowth of small, nonmyelinated nerve fibers.44–46 It has been hypothesized that discogenic pain arises because these nociceptive nerve fibers grow into areas of the disc that previously had no neurons.
Etiology of Intervertebral Disc Degeneration
The incidence of intervertebral disc degeneration increases with age and is most common in the lumbar spine.47,48 Multiple risk factors have been hypothesized as the underlying cause, including age-related factors, genetic predisposition, and numerous environmental factors. Biomechanical studies have shown that excessive mechanical loading causes disruption of disc structure including endplate defects, fissures, bulging, disc prolapse, and annular collapse.49 Further experiments have confirmed that structural damage precipitates a cascade of cell-mediated responses, leading to further damage. Although mechanical loading may precipitate degeneration, the most important cause may be processes that weaken the disc before structural damage or processes that impair the healing response. The combined effects of aging, unfavorable genetics, altered nutrition and metabolite transport, excessive or repetitive loading, and the resulting cascade of cellular events all contribute to the process of degeneration.
Aging
Aging causes progressive changes in disc nutrient supply and ECM composition, and these changes decrease tissue strength and alter cell metabolism. The alterations of proteoglycans and GAGs, decreased hydration, and changes in collagen distribution and cross-linking make the disc physically more vulnerable to injury. The altered vascular supply to the disc has been hypothesized as the primary cause of disc degeneration. Experimental endplate damage leads to degeneration33 despite enhanced metabolite transport into the disc, however, suggesting that structural damage more strongly influences the degenerative process. Inadequate nutrition likely predisposes the disc to degeneration by compromising its ability to respond to increased loading or injury.
Genetic Predisposition
Some authors suggest that genetic predisposition is the greatest risk factor for disc degeneration, accounting for approximately 50% to 70% of the variability in identical twin studies.50–52 Individual gene polymorphisms associated with disc degeneration include aggrecan,53 cartilage intermediate layer protein,54 collagen type IX,55,56 MMP-3,57 and vitamin D receptor.58,59 The products of these genes alter the ECM composition, decrease tissue strength, impair regenerative capability, and undoubtedly influence disc cell function. Degeneration develops after many decades, however, and preferentially affects the lumbar spine, even though the unfavorable genetic predisposition is present since birth. This suggests that genetic inheritance and polymorphic variations in susceptibility genes predispose the disc toward degeneration, but further insults such as excessive loading or structural damage are necessary to trigger the cascade of degenerative events.
Nutrition
The failure of nutrient supply is hypothesized to be a primary cause of disc degeneration.60 The metabolic activity of disc cells in vitro is sensitive to extracellular oxygen and pH, with matrix synthesis rates decreasing at acidic pH and low oxygen concentrations.61,62 A decrease in nutrient supply causing decreased oxygen concentration or pH could negatively affect the ability of disc cells to synthesize and maintain the ECM, ultimately leading to disc degeneration.
A relationship between loss of cell viability and a decrease in nutrient transport in scoliotic discs has been found,63 and there is evidence that nutrient transport is affected in disc degeneration in vivo.64,65 Likewise, the transport of solutes from bone to disc measured in vitro was significantly lower in degenerated discs compared with normal discs.60 Other factors affecting the blood supply to the vertebral body that may lead to an increased incidence of disc degeneration include atherosclerosis,66,67 sickle cell anemia, caisson disease (decompression sickness), and Gaucher disease. In addition, calcification of the cartilaginous endplates can cause decreased nutritional supply even if the blood supply remains undisturbed, as seen in scoliotic discs.60,68 This evidence supports the hypothesis that a decrease in nutrient supply ultimately leads to degeneration of the disc.
Environmental Factors
Environmental risk factors hypothesized to influence disc degeneration include heavy or repetitive mechanical loading (i.e., occupational physical loading and whole-body vibration),51,69 obesity, and cigarette smoking.70 Heavy physical loading, particularly related to occupation, was previously suspected to be a major risk factor for degeneration and commonly viewed as a “wear and tear” phenomenon. Results of identical twin studies on physical loading specific to occupation or sport suggest, however, that repetitive physical loading plays a relatively minor role in disc degeneration.69
Facet Joints, Ligaments, and Vertebral Bodies
Facet Joints
Degeneration of the facet joints resembles osteoarthritic changes occurring at other synovial joints, starting with synovitis and progressing to articular cartilage loss, capsular redundancy, and eventually degenerative spondylolisthesis. Hypertrophic osteophytes at the joint margins and periarticular fibrosis can also result in reduced mobility and pain at the facet joint. Osteoarthritis of the facet joints parallels degenerative changes of the disc, possibly resulting from abnormal loading and narrowing of the disc in the early stages of degeneration.71
Vertebral Bodies
Osteoarthritic changes of the vertebral body are also associated with intervertebral disc degeneration.72 The cartilaginous endplates are normally the weakest structure under compressive loads, and thinning and calcification with aging further compromise endplate strength. The endplates accumulate trabecular microdamage73 and undergo remodeling in response to altered loads, and the nucleus bulges into the vertebral body as degeneration progresses. Endplate damage decompresses the nucleus further, and loss of disc height transfers forces onto the anulus, causing it to bulge into the nucleus.49,74 The nucleus may eventually herniate through a damaged endplate, and subsequent calcification of the herniated nucleus is called a Schmorl node. The loss of disc height and annular laxity leads to formation of osteophytes at the vertebral body margins, decreased separation of the posterior neural arches, and eventual bony ankylosis (Fig. 6–6).
Intervertebral Disc Disorders and Treatment
Disc Herniation
The most common intervertebral disc disorder spinal surgeons encounter is a herniated or prolapsed nucleus pulposus, resulting in nerve root compression and radiculopathic pain. Although herniation is often thought to result from a mechanically induced rupture, it can be induced in vitro only in healthy discs by forces greater than normally encountered in vivo.75 Examination of postmortem and surgical disc specimens suggests sequestration or herniation results from the migration of isolated, degenerated fragments of the normally central nucleus pulposus through preexisting tears in the anulus fibrosus.76
Herniation-induced pressure of the nerve root alone may not account for pain associated with this condition because more than 70% of asymptomatic people have disc prolapses but no pain.77,78 Researchers have hypothesized that the nerves in symptomatic patients are sensitized to pressure,79 possibly by molecules such as prostaglandin E2, thromboxane, phospholipase A2, tumor necrosis factor-α, ILs, and MMPs. These molecules are produced by cells of herniated discs30 and may sensitize the affected nerve root because of the close proximity between the nerve and herniated disc material.80
Spinal Deformities
With scoliosis and kyphosis, whether congenital, neuromuscular, or idiopathic, there is wedging of the intervertebral discs and vertebral bodies. Several biochemical changes have been identified in scoliotic discs, which have been shown to differ from discs without scoliosis.25 These alterations include alterations in collagen production and cross-linking patterns,81 marked endplate calcification,82 and alterations in nutrient pathways.63
Treatment
Currently, no treatment is available to prevent, slow, or reverse intervertebral disc degeneration. The conservative and surgical treatments currently offered to patients are aimed at treating the end-stage manifestations of the disease rather than altering the course. The major surgical treatments available to spine surgeons include discectomy, spinal arthrodesis, and disc replacement. These procedures can produce pain relief, but they also change the biomechanics of the spine, possibly accelerating degeneration at adjacent levels.83,84
1 Boos N, Weissbach S, Rohrback H, et al. Classification of age-related changes in lumbar intervertebral discs. 2002 Volvo Award in basic science. Spine. 2002;27:2631-2644.
2 Battié MC, Videman T, Gibbons LE, et al. 1995 Volvo Award in clinical sciences. Determinants of lumbar disc degeneration: A study relating lifetime exposures and magnetic resonance imaging findings in identical twins. Spine. 1995;20:2601-2612.
3 Boden SD, Davis DO, Dina TS, et al. Abnormal magnetic-resonance scans of the lumbar spine in asymptomatic subjects: A prospective investigation. J Bone Joint Surg Am. 1990;72:403-408.
This study confirms that a significant number of pain-free subjects have herniated discs.
4 Le Maitre CL, Freemont AJ, Hoyland JA. Localization of degradative enzymes and their inhibitors in the degenerate human intervertebral disc. J Pathol. 2004;204:47-54.
5 Kang JD, Stefanovic-Racic M, McIntyre LA, et al. Toward a biochemical understanding of human intervertebral disc degeneration and herniation: Contributions of nitric oxide, interleukins, prostaglandin E2, and matrix metalloproteinases. Spine. 1997;22:1065-1073.
1 Buckwalter JA. Aging and degeneration of the human intervertebral disc. Spine. 1995;20:1307-1314.
2 Miller JA, Schmatz C, Schultz AB. Lumbar disc degeneration: Correlation with age, sex, and spine level in 600 autopsy specimens. Spine. 1988;13:173-178.
3 Bernick S, Cailliet R. Vertebral end-plate changes with aging of human vertebrae. Spine. 1982;7:97-102.
4 Maroudas A, Stockwell RA, Nachemson A, et al. Factors involved in the nutrition of the human lumbar intervertebral disc: Cellularity and diffusion of glucose in vitro. J Anat. 1975;120(Pt 1):113-130.
5 Watanabe H, Yamada Y, Kimata K. Roles of aggrecan, a large chondroitin sulfate proteoglycan, in cartilage structure and function. J Biochem. 1998;124:687-693.
6 Pazzaglia UE, Salisbury JR, Byers PD. Development and involution of the notochord in the human spine. J R Soc Med. 1989;82:413-415.
7 Sive JI, Baird P, Jeziorsk M, et al. Expression of chondrocyte markers by cells of normal and degenerate intervertebral discs. Mol Pathol. 2002;55:91-97.
8 Inoue H. Three-dimensional architecture of lumbar intervertebral discs. Spine. 1981;6:139-146.
9 Errington RJ, Puustjarvi K, White IR, et al. Characterisation of cytoplasm-filled processes in cells of the intervertebral disc. J Anat. 1998;192(Pt 3):369-378.
10 Urban JP, Holm S, Maroudas A. Diffusion of small solutes into the intervertebral disc: As in vivo study. Biorheology. 1978;15:203-221.
11 Holm S, Maroudas A, Urban JP, et al. Nutrition of the intervertebral disc: Solute transport and metabolism. Connect Tissue Res. 1981;8:101-119.
12 Ferguson SJ, Ito K, Nolte LP. Fluid flow and convective transport of solutes within the intervertebral disc. J Biomech. 2004;37:213-221.
13 Urban JP, Smith S, Fairbank JC. Nutrition of the intervertebral disc. Spine. 2004;29:2700-2709.
14 Feng H, Danfelter M, Strömqvist B, et al. Extracellular matrix in disc degeneration. J Bone Joint Surg Am. 2006;88(Suppl 2):25-29.
15 Roberts S, Caterson B, Menage J, et al. Matrix metalloproteinases and aggrecanase: Their role in disorders of the human intervertebral disc. Spine. 2000;25:3005-3013.
16 Melrose J, Ghosh P, Taylor TK. Neutral proteinases of the human intervertebral disc. Biochim Biophys Acta. 1987;923:483-495.
17 Roberts S, Menage J, Urban JP. Biochemical and structural properties of the cartilage end-plate and its relation to the intervertebral disc. Spine. 1989;14:166-174.
18 Antoniou J, Steffen T, Nelson F, et al. The human lumbar intervertebral disc: Evidence for changes in the biosynthesis and denaturation of the extracellular matrix with growth, maturation, ageing, and degeneration. J Clin Invest. 1996;98:996-1003.
19 Nachemson A, Elfstrom G. Intravital dynamic pressure measurements in lumbar discs: A study of common movements, maneuvers and exercises. Scand J Rehabil Med Suppl. 1970;1:1-40.
20 Boos N, Wallin A, Gbedegbegnon T, et al. Quantitative MR imaging of lumbar intervertebral disks and vertebral bodies: Influence of diurnal water content variations. Radiology. 1993;188:351-354.
21 Eyre DR, Muir H. Quantitative analysis of types I and II collagens in human intervertebral discs at various ages. Biochim Biophys Acta. 1977;492:29-42.
22 Johnstone B, Bayliss MT. The large proteoglycans of the human intervertebral disc: Changes in their biosynthesis and structure with age, topography, and pathology. Spine. 1995;20:674-684.
23 Urban JP, McMullin JF. Swelling pressure of the intervertebral disc: Influence of proteoglycan and collagen contents. Biorheology. 1985;22:145-157.
24 Boos N, Weissbach S, Rohrbach H, et al. Classification of age-related changes in lumbar intervertebral discs. 2002 Volvo Award in basic science. Spine. 2002;27:2631-2644.
25 Duance VC, Crean JK, Sims TJ, et al. Changes in collagen cross-linking in degenerative disc disease and scoliosis. Spine. 1998;23:2545-2551.
26 Adams MA, Roughley PJ. What is intervertebral disc degeneration, and what causes it? Spine. 2006;31:2151-2161.
27 Le Maitre CL, Pockert A, Buttle DJ, et al. Matrix synthesis and degradation in human intervertebral disc degeneration. Biochem Soc Trans. 2007;35(Pt 4):652-655.
28 Roberts S, Evans H, Trivedi J, et al. Histology and pathology of the human intervertebral disc. J Bone Joint Surg Am. 2006;88(Suppl 2):10-14.
29 Le Maitre CL, Freemont AJ, Hoyland JA. Localization of degradative enzymes and their inhibitors in the degenerate human intervertebral disc. J Pathol. 2004;204:47-54.
30 Kang JD, Georgescu HI, McIntyre-Larkin L, et al. Herniated lumbar intervertebral discs spontaneously produce matrix metalloproteinases, nitric oxide, interleukin-6, and prostaglandin E2. Spine. 1996;21:271-277.
31 Kang JD, Stefanovic-Racic M, McIntyre LA, et al. Toward a biochemical understanding of human intervertebral disc degeneration and herniation: Contributions of nitric oxide, interleukins, prostaglandin E2, and matrix metalloproteinases. Spine. 1997;22:1065-1073.
32 Le Maitre CL, Freemont AJ, Hoyland JA. The role of interleukin-1 in the pathogenesis of human intervertebral disc degeneration. Arthritis Res Ther. 2005;7:R732-R745.
33 Holm S, Holm AK, Ekström L, et al. Experimental disc degeneration due to endplate injury. J Spinal Disord Tech. 2004;17:64-71.
34 Gruber HE, Hanley ENJr. Analysis of aging and degeneration of the human intervertebral disc: Comparison of surgical specimens with normal controls. Spine. 1998;23:751-757.
35 Trout JJ, Buckwalter JA, Moore KC. Ultrastructure of the human intervertebral disc: II. Cells of the nucleus pulposus. Anat Rec. 1982;204:307-314.
36 Zhao CQ, Wang LM, Jiang LS, et al. The cell biology of intervertebral disc aging and degeneration. Ageing Res Rev. 2007;6:247-261.
37 Hastreiter D, Ozuna RM, Spector M. Regional variations in certain cellular characteristics in human lumbar intervertebral discs, including the presence of alpha-smooth muscle actin. J Orthop Res. 2001;19:597-604.
38 Johnson WE, Eisenstein SM, Roberts S. Cell cluster formation in degenerate lumbar intervertebral discs is associated with increased disc cell proliferation. Connect Tissue Res. 2001;42:197-207.
39 Osti OL, Vernon-Roberts B, Fraser RD. 1990 Volvo Award in experimental studies. Annulus tears and intervertebral disc degeneration: An experimental study using an animal model. Spine. 1990;15:762-767.
40 Thompson JP, Pearce RH, Schechter MT, et al. Preliminary evaluation of a scheme for grading the gross morphology of the human intervertebral disc. Spine. 1990;15:411-415.
41 Yasuma T, Koh S, Okamura T, et al. Histological changes in aging lumbar intervertebral discs: Their role in protrusions and prolapses. J Bone Joint Surg Am. 1990;72:220-229.
42 Adams MA, McMillan DW, Green TP, et al. Sustained loading generates stress concentrations in lumbar intervertebral discs. Spine. 1996;21:434-438.
43 Roberts S, Eisenstein SM, Menage J, et al. Mechanoreceptors in intervertebral discs: Morphology, distribution, and neuropeptides. Spine. 1995;20:2645-2651.
44 Freemont AJ, Peacock TE, Goupille P, et al. Nerve ingrowth into diseased intervertebral disc in chronic back pain. Lancet. 1997;350:178-181.
45 Freemont AJ, Watkins A, Le Maitre C, et al. Nerve growth factor expression and innervation of the painful intervertebral disc. J Pathol. 2002;197:286-292.
46 Kauppila LI. Ingrowth of blood vessels in disc degeneration: Angiographic and histological studies of cadaveric spines. J Bone Joint Surg Am. 1995;77:26-31.
47 Luoma K, Riihimäki H, Luukkonen R, et al. Low back pain in relation to lumbar disc degeneration. Spine. 2000;25:487-492.
48 Videman T, Battié MC, Gill K, et al. Magnetic resonance imaging findings and their relationships in the thoracic and lumbar spine: Insights into the etiopathogenesis of spinal degeneration. Spine. 1995;20:928-935.
49 Adams MA, Freeman BJ, Morrison HP, et al. Mechanical initiation of intervertebral disc degeneration. Spine. 2000;25:1625-1636.
50 Battié MC, Videman T, Gibbons LE, et al. 1995 Volvo Award in clinical sciences. Determinants of lumbar disc degeneration: A study relating lifetime exposures and magnetic resonance imaging findings in identical twins. Spine. 1995;20:2601-2612.
51 Battie MC, Videman T, Parent E. Lumbar disc degeneration: Epidemiology and genetic influences. Spine. 2004;29:2679-2690.
52 Sambrook PN, MacGregor AJ, Spector TD. Genetic influences on cervical and lumbar disc degeneration: A magnetic resonance imaging study in twins. Arthritis Rheum. 1999;42:366-372.
53 Kawaguchi Y, Osada R, Kanamori M, et al. Association between an aggrecan gene polymorphism and lumbar disc degeneration. Spine. 1999;24:2456-2460.
54 Seki S, Kawaguchi Y, Chiba K, et al. A functional SNP in CILP, encoding cartilage intermediate layer protein, is associated with susceptibility to lumbar disc disease. Nat Genet. 2005;37:607-612.
55 Ala-Kokko L. Genetic risk factors for lumbar disc disease. Ann Med. 2002;34:42-47.
56 Paassilta P, Lohiniva J, Göring HH, et al. Identification of a novel common genetic risk factor for lumbar disk disease. JAMA. 2001;285:1843-1849.
57 Takahashi M, Haro H, Wakabayashi Y, et al. The association of degeneration of the intervertebral disc with 5a/6a polymorphism in the promoter of the human matrix metalloproteinase-3 gene. J Bone Joint Surg Br. 2001;83:491-495.
58 Kawaguchi Y, Kanamori M, Ishihara H, et al. The association of lumbar disc disease with vitamin-D receptor gene polymorphism. J Bone Joint Surg Am. 2002;84:2022-2028.
59 Videman T, Gibbons LE, Battié MC, et al. The relative roles of intragenic polymorphisms of the vitamin D receptor gene in lumbar spine degeneration and bone density. Spine. 2001;26:E7-E12.
60 Nachemson A, Lewin T, Maroudas A, et al. In vitro diffusion of dye through the end-plates and the annulus fibrosus of human lumbar inter-vertebral discs. Acta Orthop Scand. 1970;41:589-607.
61 Ishihara H, Urban JP. Effects of low oxygen concentrations and metabolic inhibitors on proteoglycan and protein synthesis rates in the intervertebral disc. J Orthop Res. 1999;17:829-835.
62 Ohshima H, Urban JP. The effect of lactate and pH on proteoglycan and protein synthesis rates in the intervertebral disc. Spine. 1992;17:1079-1082.
63 Urban MR, Fairbank JC, Bibby SR, et al. Intervertebral disc composition in neuromuscular scoliosis: Changes in cell density and glycosaminoglycan concentration at the curve apex. Spine. 2001;26:610-617.
64 Bartels EM, Fairbank JC, Winlove CP, et al. Oxygen and lactate concentrations measured in vivo in the intervertebral discs of patients with scoliosis and back pain. Spine. 1998;23:1-7. discussion 8
65 Rajasekaran S, Babu JN, Arun R, et al. ISSLS prize winner. A study of diffusion in human lumbar discs: A serial magnetic resonance imaging study documenting the influence of the endplate on diffusion in normal and degenerate discs. Spine. 2004;29:2654-2667.
66 Kauppila LI. Prevalence of stenotic changes in arteries supplying the lumbar spine: A postmortem angiographic study on 140 subjects. Ann Rheum Dis. 1997;56:591-595.
67 Kauppila LI, McAlindon T, Evans S, et al. Disc degeneration/back pain and calcification of the abdominal aorta: A 25-year follow-up study in Framingham. Spine. 1997;22:1642-1647. discussion 1648-1649
68 Roberts S, Urban JP, Evans H, et al. Transport properties of the human cartilage endplate in relation to its composition and calcification. Spine. 1996;21:415-420.
69 Videman T, Sarna S, Battié MC, et al. The long-term effects of physical loading and exercise lifestyles on back-related symptoms, disability, and spinal pathology among men. Spine. 1995;20:699-709.
70 Battié MC, Videman T, Gill K, et al. 1991 Volvo Award in clinical sciences. Smoking and lumbar intervertebral disc degeneration: An MRI study of identical twins. Spine. 1991;16:1015-1021.
71 Boden SD, Riew KD, Yamaguchi K, et al. Orientation of the lumbar facet joints: Association with degenerative disc disease. J Bone Joint Surg Am. 1996;78:403-411.
72 Simpson EK, Parkinson IH, Manthey B, et al. Intervertebral disc disorganization is related to trabecular bone architecture in the lumbar spine. J Bone Miner Res. 2001;16:681-687.
73 Vernon-Roberts B, Pirie CJ. Healing trabecular microfractures in the bodies of lumbar vertebrae. Ann Rheum Dis. 1973;32:406-412.
74 Urban JP, Roberts S. Degeneration of the intervertebral disc. Arthritis Res Ther. 2003;5:120-130.
75 Adams MA, Hutton WC. Prolapsed intervertebral disc: A hyperflexion injury. 1981 Volvo Award in Basic Science. Spine. 1982;7:184-191.
76 Moore RJ, Vernon-Roberts B, Fraser RD, et al. The origin and fate of herniated lumbar intervertebral disc tissue. Spine. 1996;21:2149-2155.
77 Boden SD, Davis DO, Dina TS, et al. Abnormal magnetic-resonance scans of the lumbar spine in asymptomatic subjects: A prospective investigation. J Bone Joint Surg Am. 1990;72:403-408.
78 Boos N, Rieder R, Schade V, et al. 1995 Volvo Award in clinical sciences. The diagnostic accuracy of magnetic resonance imaging, work perception, and psychosocial factors in identifying symptomatic disc herniations. Spine. 1995;20:2613-2625.
79 Cavanaugh JM. Neural mechanisms of lumbar pain. Spine. 1995;20:1804-1809.
80 Kawakami M, Tamaki T, Weinstein JN, et al. Pathomechanism of pain-related behavior produced by allografts of intervertebral disc in the rat. Spine. 1996;21:2101-2107.
81 Crean JK, Roberts S, Jaffray DC, et al. Matrix metalloproteinases in the human intervertebral disc: Role in disc degeneration and scoliosis. Spine. 1997;22:2877-2884.
82 Roberts S, Menage J, Eisenstein SM. The cartilage end-plate and intervertebral disc in scoliosis: calcification and other sequelae. J Orthop Res. 1993;11:747-757.
83 Bao QB, McCullen GM, Higham PA, et al. The artificial disc: Theory, design and materials. Biomaterials. 1996;17:1157-1167.
84 Eck JC, Humphreys SC, Hodges SD. Adjacent-segment degeneration after lumbar fusion: A review of clinical, biomechanical, and radiologic studies. Am J Orthop. 1999;28:336-340.