CHAPTER 40 The eye
The outer surface of the eye is composed of parts of two spheres with different radii. The anterior segment, part of the smaller sphere, is formed by the transparent cornea and accounts for approximately 7% of the ocular surface. The posterior segment of the eyeball is part of the larger sphere formed by the opaque sclera (Fig. 40.1).
Internal to the sclera is a vascular, pigmented layer consisting of three continuous parts that collectively make up the uveal tract: a thin choroid lying posteriorly, a thicker ciliary body and an anterior iris which is displaced from the outer coat and terminates at the pupillary aperture (Fig. 40.1). The internal surface of the choroid is covered by the photosensory retina, which terminates anteriorly at the ora serrata, which also marks the junction between the ciliary body and choroid. The vasculature of the choroid supplies nutrients to the avascular outer retina.
The ocular lens is located immediately behind the iris and is suspended from the ciliary body via zonular fibres (Fig. 40.1). Smooth muscles within the ciliary body regulate the tension exerted on the elastic lens and hence determine its shape, thereby adjusting the focus of the eye in the process of accommodation. The iris, which does not allow the transmission of light due to a heavily pigmented posterior surface, also contains smooth muscle, allowing it to regulate the size of the pupillary aperture.
The iris and lens separate the eye into three chambers. The largest, the vitreous chamber, is filled with the gel-like vitreous humour, and lies posterior to the lens, comprising about two-thirds of the volume of the eye (Fig. 40.1). The spaces between the lens and iris, and the iris and cornea, are the posterior and anterior chambers respectively of the eye. Both are filled with aqueous humour, which is produced by the epithelium of the ciliary body, travels through the pupil, circulates in the anterior chamber and is drained principally through the canal of Schlemm at the iridocorneal filtration angle. Aqueous humour provides metabolic support to the avascular lens and cornea.
The sole purpose of the eyes and their associated structures within the bony orbit is to form a good image on a healthy retina. The photoreceptors of the retina transduce the optical radiation into neurobiological activity and other cells within the retina then begin to process the image. The retinal signal is transferred via the optic nerve, along the visual pathway (see Fig. 40.33) to various regions within the brain, where further processing results in visual perception.
OUTER COAT
The fibrous outer coat of the eye consists of the opaque posterior sclera and the transparent anterior cornea. Together they form a semi-elastic protective capsule enclosing the eye, which when made turgid by intraocular pressure, determines the optical geometry of the eye and ensures its shape is not distorted when it moves. The sclera also provides attachments for the extraocular muscles, and its smooth external surface rotates easily on the adjacent tissues of the orbit when these muscles contract (see Ch. 39). The opacity of the sclera helps ensure that only light that enters the eye through the pupil reaches the retina. The cornea, on the other hand, not only admits light but its covering tear film (see p. 673) is also the major refractive surface of the eye.
SCLERA
The sclera accounts for approximately 93% of the outer coat of the eye. Anteriorly, it is continuous with the cornea at the corneoscleral junction (limbus) (Fig. 40.1). It is punctured by a number of foramina containing nerves and blood vessels, most notably the optic foramen, which lies 3 mm medial to the midline and 1 mm below the horizontal and houses the optic nerve. Smaller openings contain anterior ciliary arteries which penetrate anteriorly, vortex veins which cross the sclera equatorially, and the long and short ciliary nerves and arteries which enter posteriorly. The sclera is thickest posteriorly (1–1.35 mm), decreasing gradually towards the equator to reach a minimum of 300 μm under the tendons of the recti. Going forwards from the insertions of the recti, it gradually increases in thickness once more to 800 μm at the limbus.
The external surface of the sclera is covered by a delicate episcleral lamina of loose fibro-vascular tissue, which contains sparse blood vessels and is in contact with the inner surface of the fascial sheath of the eyeball (see p. 657). Anteriorly the external scleral surface is covered by conjunctiva which is reflected onto it from the deep surfaces of the eyelids. The scleral internal surface adjacent to the choroid is attached to it by a delicate fibrous layer, the suprachoroid lamina, which contains numerous fibroblasts and melanocytes. Anteriorly, the inner sclera is attached to the ciliary body by the lamina supraciliaris. Posteriorly, the sclera is pierced by the optic nerve. Here the outer half of the sclera turns back to become continuous with the dura mater, while the inner half is modified to form a perforated plate, the lamina cribrosa sclerae. The optic nerve fascicles pass through these minute orifices, while the central retinal artery and vein pass through a larger, central aperture. The lamina cribrosa sclerae is the weakest part of the sclera and bulges outwards (a cupped disc) when intraocular pressure is raised chronically as in glaucoma (see p. 686).
Like the cornea, the scleral stroma is composed mainly of densely packed collagen embedded in a matrix of proteoglycans, which are mixed with occasional elastic fibres and fibroblasts. However, in contrast to the cornea, scleral collagen fibrils show a large variation in diameter and spacing, and the lamellae branch and interlace extensively. This arrangement of fibres results in increased light scatter, which is responsible for the opaque dull white appearance of the sclera, and also imparts a high tensile strength to the sclera to resist the pull of the extraocular muscles and contain the intraocular pressure. Collagen fibre bundles are arranged circumferentially around the optic disc and the orifices of the lamina cribrosa. The fibres of the tendons of the recti intersect scleral fibres at right angles at their attachments, and then interlace deeper in the sclera. Collagen fibres of the scleral spur are orientated circularly, and there is an increased incidence of elastic fibres here. Although the sclera acts as a conduit for blood vessels, scleral vessels are few and mainly disposed in the episcleral lamina, especially close to the limbus. Its nerve supply is surprisingly rich, accounting for the intense pain associated with scleral inflammation (Watson & Young 2004).
Filtration angle and aqueous drainage
Aqueous humour is produced by the ciliary epithelium, passes through the pupil and circulates within the anterior chamber supplying the avascular cornea and lens with nutrients. It drains from the eye mainly through the trabecular meshwork into the canal of Schlemm at the iridocorneal (filtration) angle (Figs 40.2, 40.3), formed between the posterior aspect of the corneoscleral limbus and the periphery of the iris.
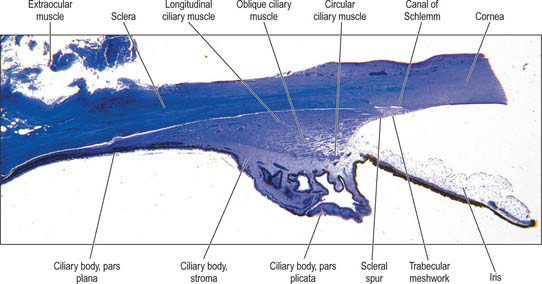
Fig. 40.2 Meridional section through the anterior eye highlighting the ciliary body and drainage apparatus.
The canal of Schlemm (sinus venosus sclerae) is an anular endothelial canal located near the internal surface of the sclera close to the limbus. In section, the canal appears as an oval cleft, with an outer wall that grooves the sclera. Posteriorly, the cleft extends as far as a rim of scleral tissue, the scleral spur, which in section forms a triangle with its apex directed forwards (Figs 40.2, 40.3). The canal of Schlemm may be double or multiple in part of its course, and its walls are constructed of a continuous single thin endothelial layer. Passage of aqueous humour to the canal probably occurs via giant pinocytotic vacuoles, which form on the inner face of the endothelium and discharge into the canal at the outer face, and through intercellular clefts. Aqueous humour passes through a plexus of fine intrascleral vessels which connect the canal of Schlemm with anterior ciliary veins (Fig. 40.4). Normally the canal does not contain blood: pressure gradients prevent the reflux of blood even though the channels between the canal and veins have no valves. However, in venous congestion, blood may enter the canal: the continuous endothelial outer wall of the canal prevents further reflux.
CORNEA
The avascular cornea is the anterior transparent part of outer coat. Convex anteriorly, it projects from the sclera as a dome-shaped elevation with an area of 1.1 cm2, forming approximately 7% of the external tunic area. Since it is more curved (average radius, r = 7.8 mm) than the sclera (r = 11.5 mm), a slight sulcus sclerae marks the corneoscleral junction (limbus). The cornea is approximately 670 μm thick close to the corneoscleral junction, and 520 μm at its centre. At the nasal and temporal limbus the transition from cornea to sclera occurs in a line that is approximately perpendicular to the cornea: this transition occurs more obliquely superiorly and inferiorly, with the sclera overlapping the cornea to a greater extent anteriorly. Consequently, when viewed from in front, the corneal perimeter is slightly elliptical, as its horizontal diameter (11.7 mm) is a little greater than its vertical (10.6 mm). Its posterior perimeter is circular (diameter 11.7 mm).
Microscopically, the cornea consists of five layers, namely, corneal epithelium, anterior limiting lamina (Bowman’s layer), substantia propria (stroma), posterior limiting lamina (Descemet’s membrane) and endothelium, arranged anteroposteriorly (Fig. 40.5A).
Corneal epithelium
The corneal epithelium accounts for approximately 10% of the corneal thickness (50 μm). It usually consists of 5–6 layers of cells (Fig. 40.5B) that protect the ocular surface from mechanical abrasion, form a permeability barrier (to small molecules, water and ions) and prevent the entry of pathogens. The deepest cells are columnar with flat bases, rounded apices, and large round or oval nuclei. Outside these are 2–3 layers of polyhedral (often wing-shaped) cells. In the more superficial layers the cells become progressively flatter, and present a smooth, optically perfect surface. Scanning electron microscopy of surface cells reveals extensive finger-like and ridge-like projections (microvilli and microplicae). A complex network of tight junctions links the superficial cells, consistent with their barrier function.
Anterior limiting lamina (Bowman’s layer)
The anterior limiting lamina lies behind the corneal epithelium. It contains a dense mass of collagen fibrils set in a matrix similar to that of the substantia propria. The lamina is 12 μm thick, is readily distinguishable from the substantia propria because it does not contain fibroblasts, and appears amorphous by light microscopy (Fig. 40.5B).
Substantia propria (stroma)
The substantia propria is approximately 500 μm thick and forms the bulk of the cornea. It is a compact and transparent layer, composed of 200–250 sequential lamellae, each made up of fine parallel collagen fibrils mainly of type I collagen. Flat dendritic interconnecting fibroblasts (keratocytes) form a coarse mesh between the lamellae. Alternate lamellae are typically orientated at large angles to each other (Fig. 40.6). X-ray diffraction studies indicate that they run along two preferred directions, superior-inferior and nasal-temporal, to account for the additional tensile stress exerted by the recti along these meridians (Boote et al 2005). Each lamella is approximately 2 μm thick and of variable width (10–250 μm, or, rarely, more). In the central cornea fibrils within the lamellae have a similar diameter of approximately 31 nm. This increases slightly with age and approaching the limbus. The small size of the fibrils (much smaller than the wavelength of light), along with the regularity of their spacing (maintained by collagen: proteoglycan interactions), and the careful control of corneal hydration, are the principal factors which determine stromal transparency. Light scattered by the collagen fibrils is eliminated by destructive interference in all directions other than forwards.
Endothelium
The endothelium covers the posterior surface of the cornea and consists of a single layer of squamous cells (Fig. 40.5C) with prominent interdigitations between adjacent cells, which are also connected by tight and gap junctions. When viewed en face, the endothelium appears as a mosaic of polygonal (typically hexagonal) cells. As these cells have a limited capacity for mitosis, in response to pathology, trauma, age and prolonged contact lens wear, the endothelial mosaic becomes less regular, and shows a greater variation in cell size and shape, as cells spread to fill gaps caused by cell loss. The numerous mitochondria and prominent rough ER within these cells reflects their high metabolic activity. Thus, for example, active pumping mechanisms largely control the degree of corneal hydration.
Corneal innervation
The cornea is innervated by numerous branches of the ophthalmic nerve (see p. 667) which either form an anular plexus around the periphery of the cornea, or pass directly from the sclera and enter the corneal stroma radially as 70–80 small groups of fibres. Upon entering the cornea, the few myelinated nerves lose their myelin sheaths and ramify throughout the corneal matrix in a delicate reticulum, their terminal filaments forming an intricate subepithelial plexus. Axon bundles from this plexus cross the anterior limiting membrane and form a subbasal plexus from which individual beaded axons pass to more superficial epithelial layers, eventually terminating as free nerve endings. Corneal nerves provide the afferent arm of the blink and lacrimal (see p. 673) reflexes and may also have a neurotrophic function.
UVEA
The vascular tunic of the eye consists of the choroid, ciliary body and iris, which collectively form a continuous structure, the uvea (Fig. 40.1). The choroid covers the internal scleral surface, and extends forwards to the ora serrata. The ciliary body continues forward from the choroid to the circumference of the iris, which is a circular diaphragm behind the cornea and in front of the lens, presenting an almost central aperture, the pupil.
CHOROID
Four layers can be identified in transverse section (Fig. 40.7, see also Fig. 40.23): suprachoroid, vascular stroma, choriocapillaris and lamina vitrea (Bruch’s membrane).
Vessel layer (stroma)
Internal to the suprachoroid lies a layer composed mainly of arteries and veins, but also some loose connective tissue containing scattered pigment cells. These melanocytes limit the passage of light through the sclera to the retina. More importantly, like the retinal pigment epithelium (see p. 689), they also absorb light traversing the retina that is not absorbed by the photoreceptors, so preventing internal reflection.
The blood supply of the choroid, as well as the rest of the uvea, is summarized in Figure 40.10. Short posterior ciliary arteries enter the eye through the sclera near the optic disc and supply the posterior choroid. These vessels branch and gradually decrease in size as they approach the retinal border. The choroidal stroma can be divided into layers based on the change in the calibre of these vessels; an outer layer of larger vessels (Haller’s layer), an inner layer of smaller vessels (Sattler’s layer) which eventually give rise to the choriocapillaris (Fig. 40.7). Long posterior ciliary arteries and recurrent branches of anterior ciliary arteries supply the anterior part of the choriocapillaris.
The vessels of the choroid have a rich autonomic vasomotor supply.
CILIARY BODY
Externally, the ciliary body may be represented by a line which extends from approximately 1.5 mm posterior to the limbus of the cornea (corresponding also to the scleral spur) to a line approximately 7.5–8 mm posterior to this on the temporal side, and 6.5–7 mm on the nasal side. The ciliary body is thus slightly eccentric. It projects posteriorly from the scleral spur, which is its attachment, with a meridional width varying from 5.5 to 6.5 mm. Internally, it exhibits a posteriorly crenated or scalloped periphery, the ora serrata, where it is continuous with the choroid and retina (Figs 40.8, 40.9). Anteriorly, it is confluent with the periphery of the iris, and externally bounds the iridocorneal angle of the anterior chamber.
In cross section the ciliary body is composed of four layers (from internal to external), namely a double layer of epithelial cells, the stroma, ciliary muscle, and a supraciliary layer (Fig. 40.2).
Meridionally, the ciliary body can be divided into two parts (Figs 40.2, 40.9). Anteriorly, the ridged anterior pars plicata (corona ciliaris) surrounds the base of the iris and accounts for about ⅓ rd of the ciliary body. Posteriorly, the relatively smooth and thin pars plana (orbicularis ciliaris) lies adjacent to the ora serrata, and forms a convenient access point for instruments during vitreo-retinal surgery and for intraocular injection. The whole ciliary body is covered by a double epithelium, in which the inner layer is unpigmented, while the outer layer contains melanin. At the ora serrata the retinal pigment epithelium is continuous with the outer pigmented epithelium of the pars plana, while the neural retina is replaced by inner unpigmented ciliary epithelium. Anteriorly, this double epithelium continues over the pars plicata until it merges with the double epithelium on the posterior surface of the iris (where the inner layer of cells also accumulates melanin) (see p. 682). The anterior pars plicata is ridged meridionally by 70–80 ciliary processes radiating from the base of the iris (Figs 40.2, 40.9). In the young eye these process are approximately parallel-sided structures, but in the adult their flanks become less regular and appear thicker. A minor ridge, or ciliary plica, lies in the valley between most of the processes. The crests of the processes are less pigmented, giving them the appearance of white (or light) striae, from which the name ciliary is derived. Fibres of the zonule (the suspensory ligaments of the lens) extend into the valleys. They pass beyond the ciliary processes to fuse with the basal lamina of the superficial epithelial layer of pars plana. Their sites of attachment are marked by striae which pass back from the valleys of the pars plicata, across the pars plana, almost as far as the apices of the dentate processes of the ora serrata (Fig. 40.8).
Ciliary epithelium
The ciliary epithelium is bilaminar, consisting of two layers of simple epithelium which are derived embryonically from the two layers of the optic cup. The superficial layer consists of columnar cells over the pars plana, and cuboidal cells over the ciliary processes of the pars plicata: it becomes irregular and more flattened between the processes. These cells contain little or no pigment. The outer layer of the ciliary epithelium, in contrast contains cuboidal cells which are loaded with melanin. The two layers are normally firmly united, but fluid may separate them pathologically. The pigment layer is attached to the stroma of the ciliary body by its basal lamina, which continues back into the basal lamina of the choroid. As a consequence of the invagination of the optic cup during development, a basal lamina covers the free surface of the bilayer which is continuous with the internal limiting membrane of the retina (see p. 692).
The ciliary epithelium is responsible for the secretion of aqueous humour (see p. 685), while the outer pigmented epithelium additionally contributes to the eye’s ‘black box effect’, like the retinal pigment epithelium, choroidal pigment and posterior iris epithelium, absorbing stray light to enhance image quality.
Ciliary stroma
The ciliary stroma is composed largely of loose bundles of collagen, which form a considerable mass between the ciliary muscle and overlying processes, and extend into both of them. This inner layer of connective tissue contains numerous larger branches of the ciliary vessels. A dense reticulum of large (up to 35 μm diameter) fenestrated capillaries is concentrated in the ciliary processes. This facilitates the passage of substances from the blood plasma during aqueous production (see p. 685). Anteriorly, near the periphery of the iris, the major arterial circle (Fig. 40.10; see also Fig. 40.14) is formed chiefly by long posterior ciliary branches of the ophthalmic artery. These enter the eye some distance behind the ocular equator and pass between the choroid and sclera to the ciliary body. Ciliary veins, also draining the iris, pass posteriorly to join the vortex veins of the choroid.
Ciliary muscle
Ciliary muscle is composed of smooth muscle cells, most of which are attached to the scleral spur and arranged in three different orientations. The outermost fibres are meridional or longitudinal, and pass posteriorly into the stroma of the choroid. The innermost fibres swerve acutely from the spur to run circumferentially as a sphincter near the periphery of the lens. Obliquely interconnecting radial fibres run between these two muscular strata, frequently forming an interweaving lattice (Figs 40.2, 40.11).
Accommodation reflex
At rest distant objects are focused on the retina in an emmetropic eye (see p. 687). In order to focus closer objects the dioptric power of the eye has to be increased, which is achieved by increasing the curvature of the lens. At rest the lens is under tension from the zonular ligaments and hence flattened. On accommodation, the ciliary muscle contracts, moving the ciliary body forwards and inwards towards the optic axis. All parts of the muscle act in concert, and tension on the zonular ligaments is relaxed. As the lens is covered by an elastic capsule (see p. 686), once tension on it is released it assumes a more convex shape suitable for focusing closer objects. The radius of curvature of the anterior lens surface changes most during accommodation.
Information from the retina passing to the visual cortex does not constitute the afferent limb of a simple reflex in the usual sense of the term, but permits the visual areas to assess the clarity of objects in the visual field. Cortical efferent information passes to the pretectal area and thence to the Edinger–Westphal nucleus, which contains preganglionic parasympathetic neurones whose axons travel in the oculomotor nerve (Fig. 40.12). Efferent impulses pass in the oculomotor nerve to the orbit where they synapse in the ciliary ganglion. Postganglionic fibres (short ciliary nerves) innervate the ciliary muscle causing it to contract. There is also a sparse sympathetic innervation of ciliary muscle, which has a very limited capacity to relax the muscle.
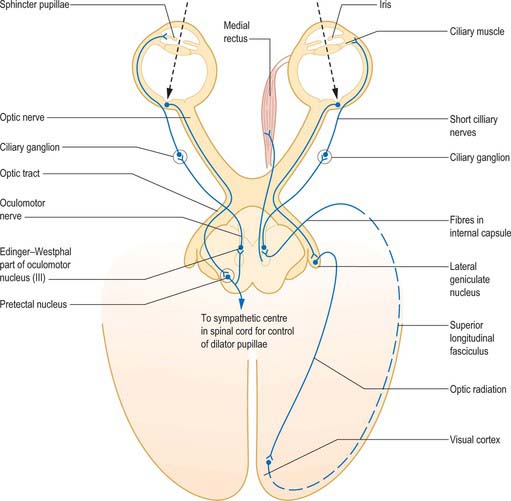
Fig. 40.12 The neural pathways of the pupillary light reflex (left) and the accommodation reflex (right).
(From Oxford Textbook of Functional Anatomy, Vol 3 Head and Neck, MacKinnon P, Morris J (eds) 1990. By permission of Oxford University Press.)
Accommodation is usually accompanied by constriction of the pupil brought about by contraction of the sphincter pupillae (see p. 682) and convergent eye movements caused by contraction of the medial, superior, and inferior recti (all innervated by the oculomotor nerve). This is the ‘near triad’ which may become disrupted in various diseases.
Supraciliary layer
The thin supraciliary layer separates the sclera from the ciliary muscle and is largely composed of collagen fibres derived from the two layers it divides. It also forms an alternative ‘unconventional’ route (other than the canal of Schlemm) for the drainage of aqueous humour (see p. 686).
IRIS
The iris is an adjustable diaphragm around a central aperture (slightly medial to true centre), the pupil. It lies between the cornea and lens and is immersed in aqueous fluid (Fig. 40.1), partially dividing the anterior segment into an anterior chamber, enclosed by the cornea and iris and a posterior chamber, which lies between the iris and the lens anterior to the vitreous. The efficacy of the iris as a light stop is mainly due to a densely pigmented posterior double epithelium. The pupillary aperture is adjusted by the action of two muscles, dilator and sphincter pupillae.
Seen from the front the iris is divided into a large ciliary zone adjacent to the ciliary body and a smaller, inner, pupillary zone (Fig. 40.13). The two regions join at the collarette. The anterior surface of the iris often contains large depressions and at the pupillary margin the posterior pigmented epithelium is visible as the pupillary ruff (Fig. 40.13).
In transverse section several subdivisions of the iris are evident (Fig. 40.14). From anterior to posterior they are an anterior border layer, the stroma (which contains the sphincter pupillae), and two pigmented epithelial layers, the most anterior of which contains the dilator pupillae. It is thinnest at its root (approximately 200 μm), where the ciliary body is attached, and thickest at the collarette.
Epithelial layers
The layer of epithelial cells nearest the stroma is, somewhat confusingly, termed the anterior epithelium, although it lies posterior to the stroma. Its cells are pigmented, as are those of the same layer in the ciliary epithelium. They give rise to the dilator pupillae (see below). Vitreal to this stratum is a layer of heavily pigmented cells, the posterior epithelium, which is continuous with the inner non-pigmented layer of the ciliary epithelium. Its free surface bears numerous radial ridges which facilitate the movement of aqueous humour from the posterior to the anterior chamber (because the front surface of the lens rests on the iris). Adjacent epithelial cells are extensively joined by various junctions which ensure that the layer can withstand the excursions of the iris during changes in pupillary size (Fig. 40.15).
Iris muscles
Sphincter pupillae
The sphincter pupillae is a flat anulus of smooth muscle approximately 750 μm wide and 150 μm thick. Its densely packed fusiform muscle cells are often arranged in small bundles, as in the ciliary muscle, and pass circumferentially around the pupil (Fig. 40.13). Collagenous connective tissue lies in front of and behind the muscle fibres. It is very dense posteriorly, where it binds the sphincter to the pupillary end of the dilator muscle, and is attached to the epithelial layer at the pupil margin. Small axons, mostly non-myelinated, ramify in the connective tissue between bundles.
Dilator pupillae
The dilator (dilatator) pupillae is a thin layer which lies immediately anterior to the epithelium of the posterior surface of the iris. Its ‘fibres’ are the muscular processes of the anterior layer of this epithelium, whose cells are therefore myoepithelial. Myofilaments are present throughout these cells, but are more abundant in their fusiform basal muscular processes, which are approximately 4 μm thick, 7 μm wide and 60 μm in length. They form a layer 3–5 elements thick through most of the iris, from its periphery to the outer perimeter of the sphincter, which it slightly overlaps. Here the dilator thins out, and sends spurs to blend with the sphincter. Unlike the apical parts of the myoepithelial cells, these have a basal lamina and are joined by gap junctions like those between the sphincteric muscle cells. Small non-myelinated axons pass between, and terminate on, their muscular processes (Fig. 40.15).
Innervation of muscles of the iris
The iris is innervated mainly by the long and short ciliary nerves. Short ciliary nerves, which contain parasympathetic postganglionic myelinated axons derived from the ciliary ganglion (see Fig. 39.17) innervate the sphincter pupillae, losing their myelin well before entering the muscle. The dilator is supplied with non-myelinated postganglionic fibres from the superior cervical ganglion; their routes are less well established. Some go via the ciliary ganglion and reach the eye in the short ciliary nerves, whereas other fibres may travel in the long ciliary nerves, which are branches of the nasociliary nerve. Both the sphincter and the dilator may have a double autonomic supply. An additional small fraction of nerve endings in the dilator and sphincter muscles have been identified as parasympathetic and sympathetic respectively in experimental animal studies, including those on primates. Although ganglion cells have been noted in the iris, the majority of axons are probably postganglionic. They form a plexus around the periphery of the iris, from which fibres extend to innervate the two muscles, the vessels, and the anterior border layer: some fibres may be afferent and others are vasomotor.
Pupillary light reflex
Pupillary diameter varies from around 2 mm when fully constricted (miosis) in bright light to at least 8 mm when dilated in darkness (mydriasis), and has an even wider range under the influence of drugs. The resulting variation in pupil area (maximally a factor of 16) will obviously affect the amount of light impinging on the retina. However, compared to the total range of illumination where humans can maintain some degree of vision (approximately 10 log units), this effect, though important, is small. Most mechanisms for dark/light adaptation are neural or biochemical. Enhancing visual acuity by restricting light to the centre of the lens, and thereby decreasing the amount of spherical aberration, is at least as important a function of pupillary constriction.
In pupillary constriction, light acting on both traditional retinal photoreceptors (rods and cones) and on intrinsically photosensitive retinal ganglion cells, gives rise to activity in retinal ganglion cells. This activity is conducted along the optic nerve, through the optic chiasma and along the optic tract. Although the majority of tract fibres end in the lateral geniculate nucleus of the thalamus, a small number leave the optic tract before it reaches the thalamus, at the superior brachium, and synapse in the olivary pretectal nucleus. The information is relayed from the pretectal nucleus by short neurones which synapse bilaterally on preganglionic parasympathetic neurones in the Edinger–Westphal nucleus (in the oculomotor nerve complex in the rostral midbrain). Efferent impulses pass along parasympathetic fibres carried by the oculomotor nerve to the orbit, where they synapse in the ciliary ganglion. Postganglionic fibres travel in the short ciliary nerves to the sphincter pupillae, which reduces the size of the pupil when it contracts (Fig. 40.12).
Vascular supply of the iris
The iris receives its blood supply from the long posterior and anterior ciliary arteries (Fig. 40.10). On reaching the attached margin of the iris, both long ciliary arteries divide into an upper and a lower branch. The branches anastomose with the corresponding contralateral arteries, and with the anterior ciliary arteries, to form the major arterial (iridic) circle (circulus arteriosus major) at the base of the iris (Figs 40.10, 40.13, 40.14). Vessels converge from this circle to the free margin of the iris, where they anastomose to form an incomplete circulus arteriosus minor: there is a view that these vessels are venous. The smaller arteries and veins are very similar in their structure and are often slightly helical, which allows them to adapt to changes in iridial shape as the pupil varies in size. All of the vessels, including the capillaries, have a non-fenestrated endothelium and a prominent, often thick, basal lamina. There is no elastic lamina in the arteries or veins, and there are few smooth muscle cells, especially in the veins. Connective tissue in the tunica media is loose, whereas the adventitia is remarkably dense and collagenous, so that it appears to form almost a separate tube.
LENS AND HUMOURS
AQUEOUS HUMOUR
Aqueous humour is derived from the plasma within the fenestrated capillaries of the ciliary processes. The major component of aqueous, like plasma, is water and the composition of the two fluids is broadly similar, although they do differ in the concentration of some electrolytes and organic solutes. In the interests of optical clarity, the blood–aqueous barrier also ensures a very low concentration of protein in the aqueous (generally <1 per cent of the level in plasma). Inflammation of the anterior uvea can lead to a breakdown of this barrier and the presence of protein in the aqueous, resulting in light scatter that is manifest clinically as ‘flare’.
LENS
The lens is a transparent, encapsulated, biconvex body bathed in aqueous humour, which serves to adjust the focus of the eye. Posteriorly, it contacts the hyaloid fossa of the vitreous body. Anteriorly, it forms a ring of contact with the posterior border of the iris, but further away from the axis of the lens the gap between the two increases to form the posterior chamber of the eye (Figs 40.1, 40.16). The lens is encircled by the ciliary processes, and is attached to them by the zonular fibres which issue mainly from the pars plana of the ciliary body. Collectively, the fibres form the zonule which holds the lens in place and transmits the forces which stretch the lens.
The lens consists of three layers (Fig. 40.17). Most of the lens is composed of elongated cells known as lens fibres. The anterior surface, as far as the equator, is covered by a layer of epithelial cells and the whole is surrounded by the lens capsule. The lens is avascular and devoid of nerve fibres or other structures which might affect its transparency. Its surface forms a very effective barrier against invasion by cells or elements of the immune system, and so creates an immunologically sequestered environment. It is unique because it retains all the cells formed throughout its life.
Lens capsule
The lens capsule is a basement membrane that covers the surface of the lens. It consists of various classes of collagen fibre (I, III and IV), as well as a range of glycosaminoglycans and glycoproteins. It is derived from the anterior lens epithelial cells and their fetal precursors and is firmly attached to the epithelium anteriorly and to lens fibres posteriorly. The capsule is elastic, a property it owes to the arrangement of its collagen, and which is central to the process of accommodation. Capsule thickness varies with position and age. One view is that it is thicker at the anterior pole and equator than at the posterior pole, with a band of increased thickness between the pole and equator (Fig. 40.16). Zonular fibres insert into the capsule at the equator. They are composed of thin (4–7 nm) fibrils with hollow centres, and resemble fibrils associated with elastic connective tissue.
Lens epithelium
The anterior surface of the lens between the outer capsule and the underlying lens fibres is covered by a layer of simple, roughly cuboidal (10 μm high and 13 μm wide), epithelial cells which, in surface view, are polygonal (Fig. 40.17). These cells differentiate into lens fibres: they undergo mitosis at a germinative zone just anterior to the equator and are displaced towards the equator, where they synthesize characteristic lens fibre proteins and undergo extreme elongation. As other cells follow suit, the earlier cells come to occupy a deeper position within the lens.
Lens fibres
Variations in lens fibre structure and composition make it possible to distinguish a softer cortical zone, representing younger fibres and a firmer central part, representing the older nucleus (Fig. 40.16). The nucleus can be further subdivided into layers representing the age at which the fibres within them were formed.
In cross-section, individual fibres are flattened hexagons measuring approximately 10 μm by 2 μm (Fig. 40.17). They are tightly packed, and fibres are firmly attached to their neighbours by a variety of mechanical junctions (Fig. 40.18). Lens fibres are also in contact through desmosomes and numerous gap junctions.
All lens fibres cross the equator (or the plane passing through it) and terminate on both the anterior and posterior lens surfaces at lens ‘sutures’. These radiate out from the poles towards the equator and represent lines of linearly registered interlocking junctions between terminating lens fibres. In fetuses, the sutures on the anterior surface of the lens form a triradiate pattern centred on the anterior pole resembling the limbs of an upright letter Y (Fig. 40.19A). Posteriorly, the sutural configuration is similar but inverted. The sutures increase in number and complexity as a consequence of lens growth and other changes in the arrangement of lens fibres (Fig. 40.19B). Fibres which start near the central axis of the lens anteriorly, terminate posteriorly on a suture near the periphery, and vice versa.
Ocular refraction
Disorders of refraction
A relaxed eye is said to be emmetropic when the refracting structures are so related to its length that the retina receives a focused image of a distant object. Although the majority of eyes are emmetropic, a large minority have errors of refraction or ametropia that can take three different forms. In myopia, the eye is too long for its refractive power and distant objects are focused in front of the retina when relaxed: closer objects will be in focus and consequently the eye is said to be ‘short-sighted’. Conversely, in hyperopia (long sight), the eye is too short for its refractive power and, when relaxed, distant objects are focused behind the retina. In astigmatism, the refractive power of the eye is not the same in different meridians, which are approximately 90 degrees apart in regular astigmatism. There is a hereditable factor in ametropia, and a relationship between myopia and the demands of close work in the young is widely accepted. These errors of refraction are amenable to correction using spectacle or contact lenses and by various forms of refractive surgery.
VITREOUS HUMOUR
The vitreous body occupies about four-fifths of the eyeball. Posteriorly it is in contact with the retina, while further forward it abuts the ciliary body, zonule and lens. Its anterior surface is hollowed into a deep concavity, the hyaloid fossa, fitting the shape of lens (Fig. 40.1). It is colourless, consisting of approximately 99% water, but is not entirely structureless. At its perimeter it has a gel-like consistency (100–300 μm thick); nearer the centre it contains a more liquid zone. Hyaluronan, in the form of long glycosaminoglycan chains, fills the whole vitreous. In addition, the peripheral gel or cortex contains a random loose network of type II collagen fibrils which are occasionally grouped into fibres. The cortex also contains scattered cells, the hyalocytes, which possess the characteristics of mononuclear phagocytes and may contribute to the production of hyaluronan. While they are normally in a resting state, they have the capacity to be actively phagocytic in inflammatory conditions. Hyalocytes are not present in the cortex bordering the lens.
A narrow hyaloid canal runs from the optic nerve head to the central posterior surface of the lens (Fig. 40.1). In the fetus this contains the hyaloid artery which normally disappears about 6 weeks before birth. The canal persists in adult life as a very delicate fibrous structure and is of no functional importance.
RETINA
The retina is a thin sheet of cells, ranging from less than 100 μm at its edge, to a maximum around 300 μm at the foveal rim. It lines the inner posterior surface of the eyeball, sandwiched between the choroid externally and the vitreous body internally, and terminates anteriorly at the ora serrata (Fig. 40.1).
When viewed with an ophthalmoscope to show the fundus oculi, the most prominent feature is the blood vessels emanating from and entering the optic disc (Fig. 40.20). Centred temporal and inferior to the disc lies the ‘central retina’ or macula (approximate diameter 5–6 mm), the middle of which is composed of the fovea and foveola, and easily identified with an ophthalmoscope as an avascular area with a yellow tinge (Fig. 40.20). The lack of blood vessels at the foveola is even more apparent in a fluorescein angiogram (Fig. 40.21). The peripheral retina lies outside the central retina.
MICROSTRUCTURE
The retina is composed of a variety of epithelial, neural and glial cell types whose distribution conventionally divides it into 10 layers (Fig. 40.22). These are usually apparent in conventional histological sections (Fig. 40.23), but can also be seen in vivo using techniques such as optical coherence tomography, which uses backscattered light to visualize layers by differences in their optical scattering properties (Fig. 40.24). Embryologically, the retina is derived from the two layers of the invaginated optic vesicle. The outer layer becomes a layer of cuboidal pigment cells which separates the choroidal lamina vitrea from the neural retina, and therefore forms the outermost layer of the retina, the retinal pigment epithelium (RPE – layer 1). The other nine strata of the retina develop from the inner layer of the optic vesicle and form the neural retina.
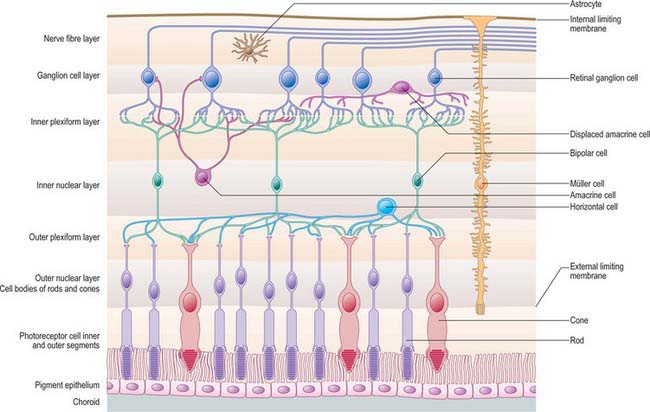
Fig. 40.22 Neural cells whose cell bodies and interconnections account for the layered appearance of the retina in histological section (compare with Fig. 40.23). Also shown are the two principal types of neuroglial cell in the retina (although microglia are also present they are not shown).
The classic ten layered appearance of the retina is absent in the optic nerve head, the fovea and foveola, and the ora serrata. At the optic nerve head, the axons of the retinal ganglion cells leave the retina to form the optic nerve and all the other neural cell types of the retina are missing. At the fovea and foveola, the inner five layers of the retina are ‘pushed aside’. At the ora serrata, where the retina borders the ciliary body (Fig. 40.9), the retinal pigment epithelium merges with the outer pigmented epithelium of the ciliary body, while the neural retina borders the inner unpigmented ciliary epithelium: the retina is thinnest at this point. The normal layered arrangement of the neural retina approaching the ora serrata is frequently disrupted by cysts in older individuals (Fig. 40.25).
Cells of the retina
Rods and cones
Rods and cones are the ‘image forming’ photoreceptors of the outer retina and function at low (scotopic) and higher (photopic) light levels respectively. Both are long, radially orientated structures with a similar organization, although details differ (Fig. 40.26). From the choroidal end inwards, the cells consist of outer and inner segments connected by a thin connecting cilium (together making up layer 2 of the retina), a cell body containing the nucleus, and a synaptic terminal (either a more complex pedicle for cones or a simpler rod spherule) where they make synaptic connections with adjacent bipolar and horizontal cells and with other cone or rod cells within the OPL.
The inner segment of both rods and cones is divided into an outer, mitochondria-rich, ellipsoid and an inner myoid which contains endoplasmic reticulum (Fig. 40.26). In most of the retina these inner segments are much wider in cones (5–6 μm at their widest point) than rods (1.5 μm) (Fig. 40.27). In both rods and cones proteins are manufactured within the myoid and incorporated into the newly formed discs at the base of the outer segment. In rods, as new discs are added, old discs are pushed up the outer segment and eventually phagocytosed by the RPE. Cone discs are also phagocytosed but the incorporation of new proteins within the discs is more diffuse. While all rods within the retina have a similar structure, the cones at the foveola are highly modified compared to those situated more peripherally, and in many ways resemble rods with a longer outer segment and a thinner inner segment.
The human retina contains on average 4.6 million cones and 92 million rods, although there is significant inter-individual variation (Curcio et al 1990). Although cones populate the whole retina, their density is highest in the foveola, where approximately 7000 cones reach an average density of 199,000 cones/mm2: this area is entirely rod free. Going outwards from the foveola, rod numbers rise, reaching a peak density in a horizontal elliptical ring at the eccentricity of the optic disc, before declining once more toward the periphery. Cone density is 40–45% higher in the nasal compared to the temporal retina, and slightly higher inferiorly than superiorly.
The number of S cones in all human retinae is similar, making up less than 10% of all cones (Curcio et al 1991; Hofer et al 2005). The distribution of S cones is relatively even throughout the retina, although they are absent from the central fovea. The relative proportions of L and M cones shows a much greater degree of variation between individuals, the L : M cone ratio varying from close to unity to over 10. The distribution of L and M cones is more irregular than that of S cones, and appears random with some indication of clumping (Bowmaker et al 2003; Hofer et al 2005).
The high packing density of cones at the foveola, achieved by decreasing inner segment size, ensures maximal resolution, while the presence of more than one spectral cone type allows colour vision. S cones probably contribute little to spatial resolution because they are absent from the foveola. Rod-based vision provides high sensitivity, but with relatively low spatial discrimination and no ability to distinguish wavelengths. Although many of the functional differences between rods and cones rely on the different properties of the photoreceptors themselves, their connectivity to other retinal neurones is equally important.
Horizontal cells
Horizontal cells are inhibitory interneurones. Their dendrites and axons extend laterally within the OPL, making synaptic contacts with cone pedicles and rod spherules, and, via gap junctions at the tips of their dendrites, with each other. Their cell bodies lie in the outer part of the inner nuclear layer (INL – layer 6). Three morphological types of horizontal cell can be distinguished in the human retina (Kolb et al 1992). The dendrites of HI and HIII cells contact cones, and their axons terminate on rods. Both the axons and dendrites of HII cells synapse only with cones.
Bipolar cells
Bipolar cells are radially orientated neurones. Their dendrites synapse on photoreceptors, horizontal cells and interplexiform cells in the OPL. Their somata are located in the INL, and axonal branches in the IPL synapse with dendrites of ganglion cells or amacrine cells. Golgi staining has identified nine distinct types of bipolar cell in the human retina (Kolb et al 1992), eight of which contact cones exclusively, and the remaining type synapses only on rods.
The IPL can be divided into two main layers, an outer layer containing the synaptic endings of ‘OFF’ cone bipolar cells, and an inner layer of ‘ON’ cone and rod bipolar cell synapses.
Amacrine cells
The various classes of amacrine cell serve a number of important functions in vision. AII cells play an essential role in the rod pathway (see above). Other cells appear to be important modulators of photoreceptive signals, and serve to adjust or maintain relative colour and luminosity inputs under changing light conditions. They are probably also responsible for some of the complex forms of image analysis known to occur within the retina, such as directional movement detection. Up to 24 different morphological types are recognized in humans (Kolb et al 1992): coupled to their neurochemical complexity, this makes them perhaps the most diverse neural cell type in the body.
Ganglion cells
The human retina contains 0.7–1.5 million ganglion cells, the output neurones of the retina (Curcio & Allen 1990). Their dendrites synapse with processes of bipolar and amacrine cells in the IPL. Ganglion cell bodies, together with displaced amacrine cells, form the ganglion cell layer of the retina (GCL – layer 8). Throughout most of the retina they form a single layer; they become progressively more numerous near the macula, where they are ranked in up to 10 rows, reaching a peak density of up to 38,000/mm2 in a horizontally orientated elliptical ring 0.4–2.0 mm from the foveal centre. Their number diminishes again towards the fovea, from which they are almost totally excluded.
Up to 15 ganglion cell types have been identified in the mammalian retina based on morphology, physiology, and target area in the brain, each of them presumably functionally distinct. For example, some project to different regions of the LGN and form three parallel visual pathways involved in conscious visual perception, namely the magnocellular and parvocellular systems and a pathway carrying the S cone signal (Wässle 2004). Midget ganglion cells (P cells) contact only single midget bipolar cells in the central retina, which in turn connect to single cones, giving each cone a ‘private line’ out of the retina and ensuring optimal acuity. The large dendritic field of parasol (M cells) is consistent with a role in motion detection. Parasol and midget ganglion cells together make up around 80% of human retinal ganglion cells. The remaining cells (approximately 200,000) project to the superior colliculus of the midbrain, the thalamic pulvinar, the pretectum and the accessory optic system, and contribute to various subconscious visual reflexes such as the pupillary and accommodation responses. In addition, a population of around 3000 large, intrinsically light-sensitive ganglion cells form a network composed of extensive overlapping dendrites (Dacey et al 2005). Such ‘inner retinal photoreceptors’ contain a retinal-based visual pigment (melanopsin; λmax approximately 479 nm) which resembles an invertebrate-type visual pigment in many of its characteristics. These light-sensitive ganglion cells are part of a pathway parallel to the rod and cone-mediated ‘image-forming’ system that monitors overall levels of illumination. This ‘non-imaging’ pathway is the major route by which the eye influences circadian rhythms via the suprachiasmatic nucleus; it also contributes to light-evoked pupillary constriction via projections to the olivary pretectal nucleus.
Ganglion cell axons, which form the NFL on the inner surface of the retina, run parallel to the surface of the retina, and converge on the optic nerve head where they leave the eye as the optic nerve. Fibres from the medial (nasal) retina approach the disc in a simple radial pattern (Fig. 40.28), whereas axons from the lateral (temporal) retina take an arcuate route as they avoid the fovea. Axons from the macula form a papillomacular fasciculus which passes almost straight to the disc. The thickness of the NFL increases dramatically near the optic disc as fibres from the peripheral retina traverse more central areas. Towards the edge of the disc the other retinal layers thin, and at the disc all neural elements of the retina other than ganglion cell axons are excluded.
Retinal glial cells
Müller cells span almost the entire thickness of the neural retina, ensheathing and separating the various neural cells except at synaptic sites. They constitute much of the total retinal volume, and almost totally fill the extracellular space between neural elements. Their nuclei lie within the INL, and from this region each cell body extends a single thick fibre that runs radially outwards, giving off complex lateral lamellae which branch among the processes of the OPL. Apically, each central process terminates at the ELM from which microvilli project for a short distance into the space between the rod and cone inner segments (fibre baskets) (Fig. 40.22). On the inner surface of the retina, the main Müller cell process expands into a terminal foot plate which contacts those of neighbouring glial cells and forms part of the internal limiting membrane (ILM; see below).
The inner border of the retina is formed by the internal limiting membrane (ILM – layer 10) which consists of collagen fibres and proteoglycans from the vitreous, a basement membrane (which is continuous with the basal lamina of the ciliary epithelium), and the plasma membrane of expanded Müller cell terminal foot plates. It is 0.5–2 μm thick in the posterior retina and thickens with age. The ILM is involved in fluid exchange between the vitreous and the retina and, perhaps through the latter, with the choroid. It also has various other functions including anchorage of retinal glial cells, and inhibition of cell migration into the vitreous body.
Modifications of the central retina
The central retina, clinically referred to as the macula, is composed of four concentric areas, which, starting with the innermost, are: the foveola (0.35 mm diameter, equivalent of an angular subtense at the nodal point of around 1.25°), the fovea (1.5 mm, 5.2°), the parafovea (2.5 mm, 8.6°), and the vaguely defined perifovea (approximately 5–6 mm, 20°). The foveola, which contains no rods or S cones, is centred about 3 mm temporal and 1 mm inferior to the optic disc (Fig. 40.20). In the foveola and surrounding fovea all the inner layers of the neural retina beyond the ONL have been displaced peripherally resulting in a retinal thickness around half of that elsewhere in the retina (Figs 40.24, 40.29). This foveal pit is created by the cone ‘axons’, known here as Henle fibres, running almost parallel to the retinal surface before connecting to post-receptoral retinal neurones outside the fovea. The Henle fibres contain two xanthophyll carotenoid pigments (lutein and zeaxanthin) which create an elliptical yellowish area (approximately 2 mm horizontally and 1 mm vertically), the macula lutea. Macular pigment density varies by more than an order of magnitude between individuals, is influenced by several environmental factors including diet, and is negligible in the central foveola.
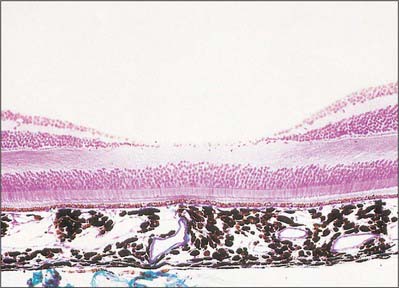
Fig. 40.29 Section through the fovea centralis
(By permission from Young B, Heath JW 2000 Wheater’s Functional Histology. Edinburgh; Churchill Livingstone.)
The absence of the inner retinal layers, including blood vessels (Figs 40.20, 40.21), reduces light scatter, which, along with the increased packing density of cones in the foveola and their lack of convergence with ganglion cells, ensures visual resolution is highest in this part of the retina. Acuity may be further enhanced by the macular pigment, which apart from having antioxidant properties and removing potentially harmful short-wave radiation, will absorb those wavelengths most prone to chromatic aberration and Rayleigh scatter.
VASCULAR SUPPLY
The retina has a dual arterial supply and both parts are necessary to maintain retinal function. The outer five layers of the retina are avascular and rely on an indirect supply from the choriocapillaris in the choroid. The inner retina receives a direct blood supply through capillaries connected to branches of the central retinal artery and vein. Only the inner retinal circulation is described here. The choroidal blood supply is discussed on p. 680.
The central retinal artery enters the optic nerve as a branch of the ophthalmic artery approximately 1.2 cm behind the eyeball, and travels within the optic nerve to its head, where it passes through the lamina cribrosa. At this level the central artery divides into equal superior and inferior branches, which, after a few millimetres, divide into superior and inferior nasal, and superior and inferior temporal, branches, each supplying a ‘quadrant’ of the retina (Fig. 40.28). Although similar retinal veins unite to form the central retinal vein, the courses of the arteries and veins do not correspond exactly. These vessels mainly run within the nerve fibre and ganglion cell layers of the retina, accounting for their clarity when seen through an ophthalmoscope (Fig. 40.20). Arteries often cross veins, usually lying superficial to them: in severe hypertension the arteries may press on the veins and cause visible dilations distal to these crossings. The vitreal location of arteries, and their lighter, bright red colouration and smaller diameter in comparison to veins, allows the two vessel types to be distinguished ophthalmoscopically.
From the four major arteries subsequent dichotomous branches run from the posterior pole to the periphery, supplying the whole retina (Zhang 1994). Arteries and veins ramify in the nerve fibre layer, near the ILM, and arterioles pass deeper into the retina to supply capillary beds. Venules return from these beds to larger superficial veins which converge towards the disc to form the central retinal vein.
Retinal capillary networks can occur in three different layers, the number of layers depending on location (Zhang 1994). Radial peripapillary capillaries are the most superficial of the capillary networks and lie within the inner nerve fibre layer. A layer of inner capillaries runs within the nerve fibre and ganglion cell layers, while an outer capillary layer is located in the inner plexiform and inner nuclear layers (Fig. 40.30). Approaching the fovea, capillaries are restricted to two layers, and terminal capillaries eventually join to form a single-layered macular capillary ring, producing a capillary-free zone 450–500 μm in diameter at the fovea. This avascular region is clearly visible in a fluorescein angiogram (Fig. 40.21). Capillaries become less numerous in the peripheral retina and are absent from a zone approximately 1.5 mm wide adjoining the ora serrata.
The territories of the arteries which supply a particular quadrant do not overlap, nor do the branches within a quadrant anastomose with each other, and consequently a blockage in a retinal artery causes loss of vision in the corresponding part of the visual field. The only exception to this end-arterial pattern is in the vicinity of the optic disc. Here, the posterior ciliary arteries enter the eye near the disc (Figs 40.10, 40.31), and their rami not only supply the adjacent choroid, but also form an anastomotic circle in the sclera around the head of the optic nerve (Fig. 40.31). Branches from this ring join the pial arteries of the nerve, and small cilioretinal arteries from any arteries in this region may enter the eye and contribute to the retinal vasculature, possibly resulting in the preservation of visual function following central retinal artery occlusion. Similarly, small retinociliary veins may sometimes also be present.
RETINOPETAL INNERVATION OF THE RETINA
The human retina receives modulating input from the brain via fibres within the optic nerve which emerge at the optic disk as large axons, and then course through the NFL, gradually diminishing in size. Although there are only a small number of these retinopetal axons (usually less than 10), they branch extensively before terminating in the IPL (Reperant et al 2006). One set of such axons arises from perikarya in the posterior hypothalamus and uses histamine as a neurotransmitter, while other, serotoninergic, fibres arise from cell bodies in the dorsal raphé. These neurones project to many targets in the central nervous system including the retina, forming components of an ascending arousal system. Such fibres allow higher central areas to modulate aspects of retinal activity; in man they may, among other things, affect sensitivity and modulate retinal blood flow (Gastinger et al 2006).
OPTIC NERVE HEAD
Histologically the ONH can be divided into three zones (Fig. 40.32). These are prelaminar (the anterior part terminating at the vitreous); laminar (formed by the lamina cribrosa); postlaminar (continuous with the retrobulbar optic nerve). The surface view of the ONH, usually seen with an ophthalmoscope, is referred to as the optic disc.
Prelaminar zone
The inner surface of the ONH is covered by an astroglial membrane (of Elschnig) that is continuous with the ILM of the retina. At the centre of the disc, the layer of astrocytes thickens into a central meniscus (of Kuhnt). Retinal ganglion cells turn into the ONH accompanied by astrocytes, which gradually increase in number posteriorly, eventually forming a sieve-like structure, the glial lamina cribrosa, through which the nerve fibres pass as separate fasciculi. At the perimeter of the ONH, a collar of astrocytes several cells thick (the intermediary tissue of Kuhnt) separates the ON from the terminating outer layers of the retina. This layer continues posteriorly and forms a barrier between the ONH and the choroid (the border tissue of Jacoby) (Fig. 40.32).
Optic disc
As it is visible by ophthalmoscopy (Fig. 40.20), the disc is a region of much clinical importance. Oedema of the disc (papilloedema) may be the first sign of raised intracranial pressure, which is transmitted into the subarachnoid space around the ON. The disc is also sensitive to the raised intra-ocular pressure which occurs in glaucoma and shows characteristic structural changes due to retinal ganglion cell loss.
The optic disc is superomedial to the posterior pole of the eye, and so lies away from the visual axis. It is round or oval, usually approximately 1.6 mm in transverse diameter and 1.8 mm in vertical diameter, and its appearance is very variable. In light-skinned subjects, the general retinal hue is a bright terracotta-red, with which the pale pink of the disc contrasts sharply; its central part is usually even paler and may be light grey. These differences are due in part to the degree of vascularization of the two regions, which is much less at the optic disc, and also to the total absence of choroidal or retinal pigment cells. In subjects with strongly melanized skins, both retina and disc are darker. The optic disc rarely projects sufficiently to justify the term papilla, although it is usually a little elevated on its lateral side, where the papillomacular nerve fibres turn into the optic nerve (Fig. 40.28). There is usually a slight depression where the retinal vessels traverse its centre.
Vascular supply
The blood supply to the three regions of the ONH differs. The prelaminar region is supplied mainly by branches of the central retinal artery (Fig. 40.31). Branches from the short posterior ciliary arteries form an often incomplete circle within the sclera around the ONH (circle of Zinn/Haller); centripetal branches from this structure supply the laminar region of the ONH. The short posterior ciliary arteries may also give off centripetal branches directly to supply the lamina, and branches that pass anteriorly to augment the prelaminar blood supply (Fig. 40.31). In the postlaminar region, arteries from the prepapillary choroid and circle of Zinn pass retrogradely as pial vessels, providing centripetal branches that supply the optic nerve. More posteriorly, the optic nerve receives pial arterioles directly from the posterior ciliary arteries. The central retinal artery may also contribute some centrifugal branches in this region.
The central retinal vein drains the ONH at all levels; other drainage pathways are minor.
VISUAL PATHWAY
The visual pathway includes the interneurones of the retina, retinal ganglion cells whose axons project via the optic nerve, chiasma, and optic tract to the lateral geniculate nucleus (LGN) and neurones within the LGN which project via the optic radiation to the primary visual cortex (Fig. 40.33).
The retina can be divided by a horizontal line bisecting the fovea. Axons arising from the nasal half of this line within each retina cross in the chiasma to enter the contralateral optic tract. Fibres from the temporal hemiretinas do not cross in the chiasma. Upper and lower temporal fibres pass laterally in the chiasma and shift respectively to medial and inferolateral positions in the ipsilateral optic tract. They are joined by the crossed fibres, the upper and lower nasal quadrants sharing the same positions as their uncrossed counterparts. Thus, each tract carries a binocular representation of the contralateral half fields as shown in Figure 40.33. It is important to remember that visual space is optically inverted by the crystalline lens when relating the spatial location of neurones within the visual pathway to corresponding visual field locations.
Axons from the LGN run in the retrolenticular part of the internal capsule and form the optic radiation. This curves dorsomedially to the primary visual cortex, located around and within the depths of the calcarine sulcus in the occipital lobe (also known as the striate cortex, Brodmann area 17, or V1; see Ch. 23). The visual cortex also has a strict retinotopic organization. Fibres representing the lower half of the visual field sweep superiorly to reach the visual cortex above the calcarine sulcus, while those representing the upper half of the visual field curve inferiorly into the temporal lobe (Meyer’s loop) before reaching the visual cortex below the calcarine sulcus. The periphery of the retina is represented anteriorly within the visual cortex, and the macula is represented towards the posterior pole, occupying a disproportionately large area that reflects the high number of foveal retinal ganglion cells that subserve the enhanced acuity of this region.
The primary visual cortex is connected to pre-striate and other cortical regions where further processing of visual stimuli occurs (see Ch. 23).
VISUAL FIELD DEFECTS
The basis for clinical assessment of damage to the visual pathway is an understanding of the retinotopic projections within the pathway. Moreover, plotting visual field loss frequently reveals the approximate location of the causative lesion and sometimes its nature (Fig. 40.33). Since retinal lesions can be visualized with an ophthalmoscope, field testing might appear to be redundant for such defects, but visual field measurement is still helpful in assessing the extent of the damage and may be the key factor in confirming a diagnosis. Glaucoma serves as an example. Field defects in glaucoma, occurring as a consequence of damage to the nerve fibre bundles at the optic nerve head, may be detectable ophthalmoscopically, but confirmation of the diagnosis frequently depends on field assessment. Early defects consist of one or more areas of paracentral focal field loss, progressing to arcuate scotomas. The shape of the defect corresponds to the anatomical arrangement of ganglion cell axons (Fig. 40.28).
So far as the location of lesions central to the retina is concerned, deficits in the vision of one eye are usually attributable to optic nerve lesions. Lesions of the optic chiasma, involving crossing nerve fibres, produce a bilateral field loss as exemplified by a pituitary adenoma (Fig. 40.33). The tumour expands upwards from the pituitary fossa, compressing the inferior midline of the chiasma, and eventually produces bitemporal hemianopia, starting with an early loss in the upper temporal quadrants. Field defects in the rare instances of optic tract lesions are distinctive. The tract contains contralateral nasal and ipsilateral temporal retinal projections and damage will cause a homonymous contralateral loss of field with substantial incongruity (dissimilar defects in the two fields). Incongruity probably results from a delay in achieving coincidence between retinal topographical projections of the two inputs of the visual pathway, as contiguous projections adjust their location, gradually achieving coincidence. It also probably reflects the reorganization of fibres which occurs normally in the optic tracts, as some fibres leave the tract in the superior brachium and others progress to the lateral geniculate nucleus. Incongruity is most marked in defects of the optic tract, less obvious in optic radiation defects, and is usually absent in cortically induced field defects, thus providing an additional clue in assessing location of the cause.
Boote C, Dennis S, Huang YF, Quantock AJ, Meek KM. Lamellar orientation in human cornea in relation to mechanical properties. J Struct Biol. 2005;149:1-6.
Bowmaker JK, Parry JWL, Mollon JD. The arrangement of L and M cones in human and a primate retina. In: Mollon JD, Pokorny J, Knoblauch K. Normal and Defective Colour Vision. Oxford: Oxford University Press, 2003.
Curcio CA, Allen KA. Topography of ganglion cells in human retina. J Comp Neurol. 1990;300:5-25.
Curcio CA, Sloan KR, Kalina RE, Henrickson AE. Human photoreceptor topography. J Comp Neurol. 1990;292:497-523.
Curcio CA, Allen KA, Sloan KR, Lerea CL, Hurley JB, Klock IB, Milam AH. Distribution and morphology of human cone photoreceptors stained with anti-blue opsin. J Comp Neurol. 1991;312:610-624.
Dacey DM, Liao H-S, Peterson BB, Robinson FR, Smith WC, Pokorny J, Yau K-W, Gamlin PD. Melanopsin-expressing ganglion cells in primate retina signal colour and irradiance and project to the LGN. Nature. 2005;433:749-754.
Gastinger MJ, Tian N, Horvath T, Marshak D. Retinopetal axons in mammals: emphasis on histamine and serotonin. Curr Eye Res. 2006;31:655-667.
Hofer H, Carroll J, Neitz J, Neitz M, Williams DR. Organization of the human trichromatic cone mosaic. J Neurosci. 2005;25:9669-9679.
Kolb H, Linberg KA, Fisher SK. Neurons of the human retina – a Golgi study. J Comp Neurol. 1992;318:147-187.
Repérant J, Ward R, Miceli D, Rio JP, Médina M, Kenigfest NB, Vesselkin NP. The centrifugal visual system of vertebrates: a comparative analysis of its functional anatomical organization. Brain Res Rev. 2006;52:1-57.
Strauus O. The retinal pigment epithelium in visual function. Phys Rev. 2005;85:845-881.
Wässle H. Parallel processing in the mammalian retina. Nature Rev Neurosci. 2004;5:1-11.
Watson PG, Young RD. Scleral structure; organisation and disease. A review. Exp Eye Res. 2004;278:609-623.
Zhang HR. Scanning electron-microscopic study of corrosion casts on retinal and choroidal angioarchitecture in man and animals. Prog Retinal Eye Res. 1994;13:243-270.