CHAPTER13 The Electrodiagnostic Examination
Pathophysiology
Because axon loss and demyelinating conduction block stop nerve impulse transmission across the lesion site rather than merely slowing it, both can result in clinical weakness and sensory abnormalities whenever they affect a sufficient number of motor and sensory axons. Demyelinating conduction slowing does not affect muscle strength, however. This is because all of the nerve impulses ultimately reach their destination, although slightly later in time than they normally would.1
General Concepts of Electrodiagnostic Examination
Nerve Conduction Studies
Three basic types of NCS are available: motor, sensory, and mixed (Fig. 13–1). Motor and sensory NCS are generally performed on every patient. Mixed NCS are typically used in the evaluation of specific disorders, such as carpal tunnel syndrome, and are of limited value in the evaluation of spine-related nerve pathology. NCS protocols vary depending on the diagnosis in question and can be tailored to help exclude other diagnoses in the differential. Most electrodiagnostic laboratories have a routine protocol, however, for a general study of the upper extremity (Table 13–1) and lower extremity (Table 13–2).
Motor | Sensory |
---|---|
Standard | |
Median: thenar (C8, T1) | Median: index (C6, C7) |
Ulnar: hypothenar (C8, T1) | Ulnar: fifth (C8) |
Nonstandard | |
Ulnar: first dorsal interosseus (C8, T1) | Median: thumb (C6) |
Radial: extensor indicis proprius (C8) | Median: middle (C7) |
Radial: brachioradialis (C5, C6) | Ulnar: hand dorsum (C8) |
Musculocutaneous: biceps (C5, C6) | Radial: thumb base (C6, C7) |
Axillary: deltoid (C5, C6) | Lateral antebrachial cutaneous: forearm (C6) |
Medial antebrachial cutaneous: forearm (T1) |
Note: On each line, the nerve being studied is listed first, followed after the colon by the recording site and then, in parentheses, the root innervation (motor) or derivation (sensory). Underlined root provides major innervation.
TABLE 13–2 Nerve Conduction Studies in the Lower Limb
Motor | Sensory |
---|---|
Standard | |
Peroneal: extensor digitorum brevis (L5-S1) | Sural: lateral ankle (S1) |
Tibial: abductor hallucis (S1) | |
Nonstandard | |
Peroneal: tibialis anterior (L5) | Superficial peroneal sensory: dorsum ankle (L5) |
Tibial: abductor digiti quinti pedis (S1) | Saphenous: medial ankle (L4)† |
Tibial: gastrocnemii (S1)* | Lateral femoral cutaneous: lateral thigh (L3, L4)† |
Femoral: quadriceps (L3, L4) |
Note: On each line, the nerve being studied is listed first, followed after the colon by the recording site and then, in parentheses, the root innervation (motor) or derivation (sensory).
† Studies technically difficult to perform.
Motor Nerve Conduction Studies
For motor NCS, the recording electrode is placed over the muscle belly, and the reference electrode is affixed over the tendon. The nerve supplying that muscle is stimulated, and the resulting motor nerve response is a compound muscle action potential (CMAP), a biphasic waveform that represents summated muscle fiber action potentials (Fig. 13–2). In routine motor NCS, small muscles of the hand and feet serve as recording muscles, and the nerves supplying them are stimulated at two separate points along their course. For the upper extremity, the wrist (distal) and elbow (proximal) are used as stimulation sites, and for the lower extremity, the ankle (distal) and knee (proximal) are used as stimulation sites.
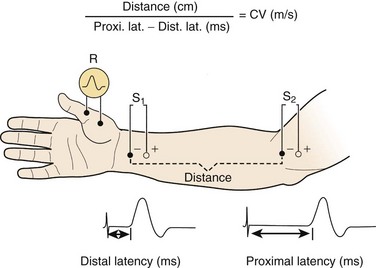
FIGURE 13–2 Various components of motor nerve conduction study. (The median nerve is being assessed.)
(Modified from Isley M, Krauss G, Levin K, et al: Electromyography/Electroencephalography. Redford, WA, Spacelabs Medical, 1993, p 40.)
Numerous parameters are assessed with each CMAP obtained, including amplitude, latency, and conduction velocity (Fig. 13–3). The CMAP amplitude represents the number of nerve fibers that responded to the stimulus and are capable of conducting impulses to the recorded muscle.1,2 It is measured from baseline to negative peak (negative being up) and reported in millivolts. The latency is the time interval between the instant the nerve was stimulated and the onset of CMAPs; these are reported in milliseconds. The conduction velocity is the speed of transmission over the fastest conducting nerve fibers assessed and is reported in meters per second. Conduction velocities are calculated by dividing the distance traveled along a nerve segment (as determined by surface measurements) by the latency difference between the responses to proximal and distal stimulation. Normal conduction velocity in the upper limb is greater than 50 m/sec; in the lower limb, it is greater than 40 m/sec.
Sensory Nerve Conduction Studies
For sensory NCS, a sensory nerve or the sensory component of a mixed nerve is stimulated at one point with recording electrodes placed distally, usually on the fingers or on the ankle with routine studies. This stimulation results in a sensory nerve action potential (SNAP), which is a biphasic or triphasic waveform that represents summated nerve action potentials. In contrast to CMAPs, which are generated by muscle fibers and are measured in millivolts, SNAPs are generated directly by the nerve fibers. SNAPs are 100 times smaller and are measured in microvolts. Generally, only two sensory NCS measurements are reported: (1) the amplitude, which is the height of the response measured from baseline to negative peak and represents the number of sensory axons that depolarize, and (2) the peak latency, which is the time interval between the moment the nerve was stimulated and the negative peak of the response, reported in milliseconds (see Fig. 13–3).1
Late Responses (H Responses and F Waves)
The H response is the electrophysiologic correlate of the Achilles tendon reflex and is named after Hoffmann, who first described it in 1918. To obtain the H response, the tibial nerve is stimulated in the popliteal fossa using low voltage to activate sensory fibers (as opposed to motor fibers), which carry the nerve impulse proximally to the spinal cord (Fig. 13–4). The fibers synapse there with motor neuron cells to complete a monosynaptic reflex arc. The nerve impulse travels down the motor efferent nerve to the gastrocnemius where the recording electrode captures the response. Although the amplitude and the latency of the H response are analyzed, the amplitude is more reliable for diagnostic purposes in the authors’ laboratory.
Needle Electrode Examination
At-Rest Phase
During the at-rest phase, electrical silence ordinarily is noted. Various types of spontaneous activity may appear, however, with neuromuscular pathology. Only three of these are relevant to spine-related nerve disease: fibrillation potentials, fasciculation potentials, and complex repetitive discharges.2–4
Fibrillation potentials are the most reliable and objective manifestation of active or recent motor axon loss. They can be neither produced nor abolished voluntarily by the patient. They are very sensitive indicators of such loss because the degeneration of a single motor axon can result in hundreds of individual muscle fibers fibrillating within a given muscle, depending on the innervation ratio of the latter. Fibrillation potentials objectively can show that motor axon loss has occurred, when the lesion is far too mild in degree to produce clinical muscle weakness, atrophy, or loss of CMAP amplitude on motor NCS.3 Showing fibrillation potentials in a myotome distribution has been the principal method of identifying root lesions in the electrodiagnostic laboratory for more than half a century.5,6
Complex repetitive discharges are produced when a single muscle fiber is depolarized and that depolarization is spread by ephaptic transmission to adjacent muscle fibers, which reactivate the initial muscle fiber. A recurrent cycle of firing is established. These potentials have a bizarre configuration and fire at high frequency. For many years, they were known as bizarre high-frequency discharges. Although they are abnormal, they are nonspecific, being seen with neuropathic and myopathic disorders. Generally, they appear when there is grouped atrophy (i.e., denervation, reinnervation, and subsequent denervation) and are evidence of chronicity. Although these potentials are not helpful in localization, they are frequently encountered on NEE of the cervical paraspinal muscles in patients with chronic cervical root lesions.3
Activation Phase
Recruitment
Reduced MUP recruitment, also known as a neurogenic MUP firing pattern, is observed whenever numerous motor units in the muscle being sampled cannot be activated on maximal effort because either conduction block or axon loss affects their axons. The fewer MUPs seen on maximal effort, the weaker the muscle is clinically. MUPs that are capable of firing are noted to do so in decreased numbers and often faster than their basal firing rate of 5 to 10 Hz.3,7 The rapid rate of firing of the still functioning motor units is important because, similar to fibrillation potentials, it is unequivocal evidence of involuntary interruption of motor axon impulse transmission. Conversely, if the muscle was weak because of an upper motor neuron lesion or because voluntary effort was simply submaximal (e.g., because of malingering or pain on activation), incomplete MUP activation would be seen—that is, MUPs would fire in equally decreased numbers but at a slow to moderate rate.
Morphology
Chronic neurogenic MUP changes generally develop about 4 to 6 months after an axon loss injury has occurred because it takes this much time for such configurational remodeling to occur. After chronic neurogenic MUP changes develop, they can persist indefinitely. With many remote, proximal neurogenic lesions (e.g., radiculopathies and particularly poliomyelitis), they are the sole electrical residuals detected during the entire electrodiagnostic examination.3,7,8
Electrodiagnostic Findings in Radiculopathy
The electrodiagnostic examination has been used to assess patients with possible radiculopathies for more than 50 years. Root lesions were one of the first focal peripheral nerve fiber disorders for which the diagnostic utility of NEE was shown.5,6 For many years, lumbosacral radiculopathies were the most common reason for referral to the electrodiagnostic laboratory.9,10 Although several other electrodiagnostic procedures have been introduced over the past half-century, NEE remains the mainstay for diagnosing radiculopathies. The amplitudes of motor NCS are also helpful when root damage is severe, extensive, or both.8,9
Nerve Conduction Studies
Routine Studies
Axon loss occurs when the axon is disconnected from its cell body. The motor cell body (anterior horn cell) resides in the anterior zone of the spinal cord; the sensory cell body (DRG) resides outside the spinal cord, either within individual intervertebral foramina or within the spinal canal (intradural and intra-arachnoid) (Fig. 13–5). A disc protrusion causing severe compression of a motor and sensory nerve root within the spinal canal disconnects the anterior horn cell from its motor axon, but if the DRG is distal to the point of compression, the extra spinal sensory axons remain connected to their DRG and do not undergo degeneration (Fig. 13–6). In that setting, motor NCS show amplitude loss, but sensory NCS are normal despite marked clinical sensory impairment with few exceptions.
One exception is seen with nerve root pathology that extends beyond the intraspinal canal. A mass lesion (e.g., meningioma) or infiltrative process (e.g., malignancy, inflammatory cause, or infection) that progresses distally along the nerve root to involve the DRG can result in decreased SNAP amplitudes. The other exception is when the DRG resides inside the intraspinal canal, proximal to the intervertebral foramina; this has been found to occur in the lumbosacral region. Based on cadaveric, radiographic, and magnetic resonance imaging (MRI) studies, 3% of L3 and L4 DRG are intraspinal, about 11% to 38% of L5 DRG are intraspinal, and 71% of S1 DRG are intraspinal.11 As a result, root lesions in the lower spine, particularly lesions involving the L5 root, can affect the corresponding SNAP amplitude, which in the case of an L5 lesion is the superficial peroneal SNAP (see later). SNAP peak latency and nerve conduction velocity are never involved in radiculopathy, however.
Late Responses
Although the H response and F wave are theoretically helpful in the evaluation of the damaged proximal nerve root segment, there are technical limitations to each procedure that can hamper their utility in the evaluation of a radiculopathy.7,9 Because the H response is elicited by stimulating the tibial nerve in the popliteal fossa while recording from the gastrocnemius/soleus muscle group as described previously, it is highly sensitive and very useful in the evaluation of S1 radiculopathy. In axon loss lesions affecting the S1 nerve root, the amplitude may be either reduced or absent. The normal value of the H amplitude as defined by the authors’ electrodiagnostic laboratory is 1 mV, with abnormal values being either less than 1 mV or reduced by 50% compared with the contralateral response. Additionally, the H response may become abnormal at the onset of nerve root injury and remain so until the injury is resolved or may remain abnormal despite resolution of clinical symptoms.7
A major limitation of the H responses is that they are frequently absent bilaterally in patients older than 60 years; in patients with polyneuropathies; and in patients who have had lumbar laminectomies, even when the S1 roots reportedly were not within the operative field. Also, when the H responses are abnormal, they do not localize to the S1 root because the lesion could be at many other points along the extended neural pathway that the impulses traverse (e.g., S1 spinal cord segment, sacral plexus, sciatic nerve, and proximal tibial nerve). When H responses are abnormal, they remain so indefinitely in many cases.8,9
Despite these limiting and confounding factors, H responses are very helpful in the evaluation of a possible lumbosacral radiculopathy because they are seldom normal with S1 root lesions. Part of their high sensitivity may be because, in contrast to all other constituents of the electrodiagnostic examination, they evaluate the preganglionic components of the S1 sensory root fibers.8,9 Although most electrodiagnostic physicians agree on the value of H responses, they disagree regarding which component (amplitude or latency) of the H response is likely to be abnormal.9,12–14
Ideally, F waves should be able to detect demyelinating conduction slowing along the motor fibers at the root level.9,15,16 This is not the case, however, in practical application. They are often normal in unequivocal cases of radiculopathy, and even when abnormal, they do not provide any additional information because the abnormalities are already clearly seen on NEE.4,7 F waves are of no significant value in the evaluation of root lesions.
Needle Electrode Examination
Numerous myotome charts derived from radiographic, cadaveric, and electrodiagnostic studies have been established to help guide the electrodiagnostic physician in choosing the best muscles to examine for each patient (Figs. 13-7 through 13-9). A radiculopathy screen in the authors’ laboratory consists of an examination of at least seven muscles, including the paraspinals to help with localization in the upper extremity (Table 13–3) and lower extremity (Table 13–4). The presence of fibrillation potentials in the paraspinals is typically indicative of an axon loss lesion localized to or near the intraspinal canal, excluding the possibility of a plexopathy or more distal lesion. Paraspinal fibrillation potentials are most valuable for the support of radiculopathy when they are present at only one or two contiguous segmental levels and absent at levels above, below, and contralaterally.
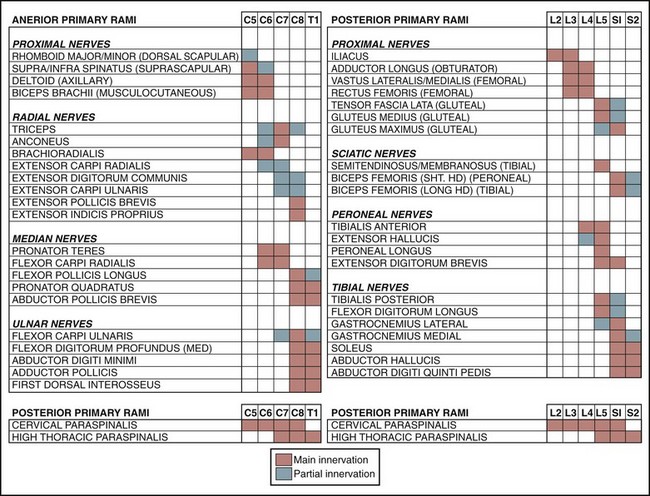
FIGURE 13–7 Traditional myotome chart.
(From Wilbourn AJ, Aminoff MF: Radiculopathies. In Brown WF, Bolton CF [eds]: Clinical Electromyography, 2nd ed. Boston, Butterworth-Heinemnann, 1993, p 192.)
TABLE 13–3 Screening Needle Electrode Examination for the Arm
Muscle | Root Level | Nerve Trunk |
---|---|---|
First dorsal interosseus | C8 | Ulnar |
Extensor indicis proprius | C8 | Posterior interosseous (radial) |
Flexor pollicis longus | C8 | Anterior interosseous (median) |
Pronator teres | C6-7 | Median |
Triceps | C6-7 | Radial |
Biceps | C5-6 | Musculocutaneous |
Deltoids | C5-6 | Axillary |
C7 paraspinal | Overlap |
TABLE 13–4 Screening Needle Electrode Examination for the Leg
Muscle | Root Level | Nerve Trunk |
---|---|---|
Abductor hallucis | S1 | Tibial |
Medial gastrocnemius | S1 | Tibial |
Biceps femoris (short head) | S1 | Peroneal |
Extensor digitorum brevis | L5-S1 | Peroneal |
Flexor digitorum longus | L5 | Tibial |
Gluteus medius | L5 | Superior gluteal |
Tibialis anterior | L4-5 | Peroneal |
Rectus femoris | L2, L3, L4 | Femoral |
S1 paraspinal | Overlap |
The timing of needle EMG is also crucial. Fibrillation potentials do not appear in a denervated muscle until 2 to 3 weeks after the onset of the initial injury and in some patients may require 4 to 6 weeks to develop.11 Consequently, the findings on NEE performed earlier than 3 weeks after onset of a radiculopathy are likely to be false-negative or, at best, indeterminate, even if subsequently they would be positive for a root lesion. It is optimal to wait at least 3 weeks after the onset of symptoms before performing NEE. Guidelines that help the clinician decide the best timing of a study to obtain maximal information are provided in Table 13–5. These are based on the neurophysiologic concepts of axon loss as described in Table 13–6.
TABLE 13–5 Appropriate Timing of the Electrodiagnostic Examination
When aiming for single comprehensive study, reliable interpretations can be made from NCS and NEE obtained any time after 3 wk from onset of symptoms |
For earliest possible information about axon loss lesion, reliable interpretations can be made from NCS obtained after 10 days from onset of symptoms |
For earliest possible information about demyelinating conduction block lesion (neurapraxia), such as might be the case for perioperative peroneal or ulnar neuropathy owing to positioning on operating table, reliable interpretations can be made from NCS obtained any time after onset of symptoms |
In setting of preexisting symptoms of peripheral nerve disease, such as diabetic polyneuropathy, it is reasonable to consider baseline electrodiagnostic examination (NCS and NEE) immediately after onset of new symptoms of potential iatrogenic cause. This study is to assess the nature of preexisting abnormalities, before acute changes from new symptoms are visible on electrodiagnostic examination. This is especially useful if a medicolegal issue may arise from new symptoms because it would be valuable to differentiate preexisting nerve pathology from any procedure-related changes. A second study is necessary when sufficient time has elapsed to assess new lesion |
NCS, nerve conduction studies; NEE, needle electrode examination.
TABLE 13–6 Timing of Nerve Pathology: Neurophysiologic Concepts
After transection of motor nerve trunk, nerve conduction response amplitude from electrical stimulation distal to transection point decreases from day 3 through day 5-8 after transection. For sensory nerve fibers, response amplitude decreases progressively from day 5 through day 9-11, coinciding with evolution of wallerian degeneration of nerve fibers. For this reason, identifying maximum axon loss cannot be assessed by nerve conduction studies until at least 11 days have elapsed since date of nerve injury or onset of symptoms |
As wallerian degeneration of motor nerve fiber reaches completion, attached muscle fiber becomes denervated, leading to breakdown of neuromuscular junction. Over 2-3 wk, membrane changes occur along muscle fiber, resulting in spontaneous, continuous action potential propagation along muscle fiber, recognized during NEE as fibrillation potentials. About 3 wk must elapse after acute axon loss event before fibrillation potentials can be reliably visualized on NEE |
Process of reinnervation of denervated muscle fibers can occur as the result of regeneration of nerve trunk from point of nerve transection, or (when nerve transection is not total) by collateral nerve branch sprouting from remaining intact nerve fibers close to denervated muscle fibers. The latter process is much faster because nerve fiber regeneration occurs at rate of about 1 mm/day. On NEE, manifestations of reinnervation include resolution of fibrillation potentials, return of activation of motor unit action potentials with voluntary muscle contraction, and appearance of polyphasic motor unit potential changes. |
NEE, needle electrode examination.
Determining Duration of Radiculopathy: Acute versus Chronic
Finally, when fibrillation potentials and chronic neurogenic MUP changes are found in a myotome distribution, the diagnostic possibilities include a chronic, progressive radiculopathy or an acute root lesion superimposed on a remote lesion. The latter possibility is the more likely choice if fibrillation potentials are found in proximal muscles (e.g., the glutei and hamstrings with L5 or S1 root lesions), in addition to more distal muscles in the same myotome.7–9
When the previous factors are considered, it is apparent that whenever the classic NEE presentation of a radiculopathy is encountered—fibrillation potentials in most or all of the muscles constituting the myotome—the root lesion in question usually is of more recent onset, and motor root axon loss has been substantial. Whenever other circumstances prevail, as is far more commonly the case, fibrillation potentials usually are found in only some, if any, of the muscles of the myotome. They are typically seen in the more distal muscles. Fibrillation potentials generally are important only if they are present; their absence in any specific muscle does not exclude the diagnosis.8,9
Electrodiagnostic Findings at Specific Root Levels
Cervical Radiculopathy
Lesions of the cervical nerve roots account for 36% of all radiculopathies.9 In clinical and radiographic studies, the most common root affected is at the C7 level (70% of the time) followed by C6 (19% to 25%), C8 (4% to 10%), and C5 (2%).9,16–18 The electrodiagnostic examination presentations with C5 radiculopathies are typically manifested as abnormalities in the spinati, deltoid, biceps, and brachioradialis muscles. NCS are typically unhelpful because proximal muscles are not assessed during routine studies, although the biceps and deltoid muscles are amenable to NCS and may show reduced CMAP amplitudes when axon loss is sufficiently severe.
C6 radiculopathies do not have a single, discrete appearance. Rather, they have two very different ones, which imitate those of C5 and C7 root lesions. Manifestations of C5 root lesions may also be seen with some C6 radiculopathies.19 C7 lesions are diagnosed by the presence of abnormalities in some muscles innervated by radial and median nerves: the triceps and anconeus (radial) and the pronator teres and flexor carpi radialis (median). As stated before, NEE abnormalities sometimes are seen in the same combination of upper limb muscles with C6 root lesions as well.
In contrast, C8 radiculopathies have a very characteristic electrodiagnostic presentation, manifesting as abnormalities in ulnar-innervated muscles, the extensor indicis proprius, and the flexor pollicis longus.19 Nonetheless, they can sometimes be confused with combined axon loss lesions of the posterior interosseous nerve and the ulnar nerve whenever the ipsilateral ulnar SNAP is of low amplitude or cannot be elicited (e.g., because of advanced age or a coexisting polyneuropathy). For uncertain reasons, the axon loss that occurs with many C8 radiculopathies is exceptionally severe, so much so that the CMAPs recorded from the ulnar nerve–innervated hand muscles, particularly the hypothenar, are low in amplitude. Some of these patients never regain normal hand strength.
Differential Diagnoses
Findings on NEE of cervical radiculopathies can look identical to brachial plexopathies (Table 13–7). In particular, lesions affecting the C5 and C6 roots may resemble upper trunk plexus lesions, whereas lesions of the C8 and T1 roots can mimic lower trunk lesions. There are two critical parameters on the electrodiagnostic examination that can discern the two types of lesions. The first parameter is NEE findings in the paraspinals. With nerve root lesions, the paraspinal muscles show fibrillation potentials but are spared in a lesion of the brachial plexus. The second parameter is the assessment of the SNAPs. In radiculopathies, the lesion is located within the intraspinal canal and proximal to the DRG, which results in normal SNAPs. In plexopathies, the lesion is distal to the DRG, producing reduced amplitude or absent SNAPs.
TABLE 13–7 Disorders Commonly Confused with Compressive Radiculopathies
Roots | Entity |
---|---|
Cervical | |
C5, C6 | Upper trunk brachial plexopathy |
Neuralgic amyotrophy | |
Axillary/suprascapular neuropathies | |
Motor neuron disease | |
Rotator cuff tear | |
C6, C7 | Carpal tunnel syndrome |
C8, T1 | Lower trunk brachial plexopathy |
Ulnar neuropathy | |
Motor neuron disease | |
Thoracic | |
T1 | Neurogenic thoracic outlet syndrome |
Lumbosacral | |
L2-4 | Diabetic amyotrophy |
Lumbar plexopathy | |
Femoral neuropathy | |
L5 | Sacral plexopathy |
Peroneal neuropathy | |
Motor neuron disease | |
S1, S2 | Sacral plexopathy |
Sciatic neuropathy | |
Tibial neuropathy | |
Bilateral (L5), S1, S2 | Polyneuropathy |
Thoracic Radiculopathy
Radiculopathies in this region are difficult to assess by electrodiagnostic examination because there are relatively few muscles in each myotome, and only some of them can be sampled. With suspected thoracic radiculopathies, only the paraspinal and abdominal muscles are sampled routinely; the intercostal muscles are typically not studied for fear of entering the pleural space. Generally, if NEE abnormalities are seen, no attempt is made to identify a specific root lesion. Instead, the localization is limited to upper thoracic, mid-thoracic, or lower thoracic root involvement. Most patients found to have thoracic radiculopathies have diabetes mellitus, and the pathology is probably root infarction or ischemia rather than compression. In any case, these radiculopathies often produce very severe axon loss and frequently apparently involve two or more adjacent roots.9,20,21 T1 radiculopathies are quite rare and typically produce changes only in the lateral thenar muscles.22
Differential Diagnoses
Although neurogenic thoracic outlet syndrome may technically be considered an extraspinal radiculopathy affecting the T1 nerve root and to a lesser extent C8, it has classically been categorized as a lower trunk brachial plexopathy (see Table 13–7). The preferential involvement of the T1 nerve root leads to prominent abnormalities of the abductor pollicis brevis muscle and the medial antebrachial cutaneous sensory response, both of which are heavily innervated by T1. In contrast, the ulnar innervated segments, which are predominantly innervated by C8, are sometimes spared or only mildly affected. Abnormalities in the abductor pollicis brevis are evident on motor NCS (manifested as decreased CMAP amplitude) and NEE (fibrillation potentials or neurogenic recruitment pattern), whereas the medial antebrachial cutaneous SNAP is reduced or absent. The latter abnormality is helpful in distinguishing this syndrome from a typical T1 radiculopathy.
Lumbosacral Radiculopathy
Nerve root lesions are most commonly seen in the lumbosacral spine: More than two thirds of all radiculopathies occur in this region.7 In contrast to lesions involving the cervical roots, it is difficult sometimes to localize lumbosacral radiculopathies accurately to a vertebral level with the electrodiagnostic examination. This difficulty is primarily due to anatomic reasons. Given their long intraspinal course, lumbosacral nerve roots may be injured anywhere along their tract from the T12-L1 vertebral level where they are formed, down through the canal into the cauda equina, and the site where they exit from their respective foramina. The L5 nerve root can be compressed by a central disc herniation at the L3-4 level, a posterolateral disc herniation at the L4-5 level, or foraminal stenosis at the L5-S1 level. Additionally, when nerves are affected at the level of the cauda equina where the fibers are compact, a single lesion in this location can result in injury to multiple roots bilaterally. It is important to perform comparison NEE of the contralateral limb when any abnormalities are seen to exclude the possibility of subclinical nerve root involvement.
The most common lumbosacral radiculopathies involve the L5 and S1 roots. Lesions of these two roots are most amenable to recognition on electrodiagnostic examination. In addition, the L5 nerve root is the most common single radiculopathy seen.3 L5 radiculopathies produce abnormalities in the tibialis anterior, flexor digitorum longus, and posterior tibialis in greater than 75% of surgically proven cases.23 In a more recent study, 100% of patients with L5 radiculopathies, which were also surgically proven, showed abnormalities in the peroneus longus and tensor fascia lata.24 Changes may also be seen in the extensor digitorum brevis, gluteus medius, and semitendinosis.
An exception to the rule that SNAPs are not affected in radiculopathies has been found to occur with some L5 root lesions. As stated before, SNAPs are typically spared in radiculopathies because the lesion is situated proximal to sensory cell bodies (DRG), which lie in the intervertebral foramina outside of the intraspinal canal. At the level of the lumbosacral spine, the DRG is sometimes found proximal to the intervertebral foramina, however, within the intraspinal canal, leaving them vulnerable to injury from a herniated disc or other degenerative spine condition. Based on cadaveric, radiographic, and MRI studies, 3% of L3 and L4 DRG are intraspinal, 11% to 38% of L5 DRG are intraspinal, and up to 71% of S1 DRG are intraspinal.24–26
The L5 nerve root in some cases may be affected distal to the DRG, resulting in an abnormal superficial peroneal SNAP. In one retrospective study, six patients with clinical and radiographic evidence of an L5 radiculopathy were found to have reduced amplitude of the ipsilateral superficial peroneal SNAP along with denervation changes in the L5 myotome.27 This condition has not been found with S1 nerve root lesions, in which the sural SNAP remains normal despite the higher percentage of DRG located within the intraspinal canal.
Differential Diagnoses
As seen in the cervical spine, it is often difficult to distinguish clinically lesions of the lumbosacral nerve roots from lesions of the lumbar and sacral plexuses (see Table 13–7). L2-4 radiculopathies can look identical to lumbar plexopathies, whereas L5-S1 nerve root lesions closely resemble lesions of the sacral plexus. In both cases, the combination of fibrillation potentials in the lumbosacral paraspinals and normal sensory nerve conduction responses (lateral femoral cutaneous and saphenous SNAPs for L2-4 lesions and sural and superficial peroneal SNAPs for L5-S1 lesions) points toward the diagnosis of radiculopathy.
Electrodiagnostic Findings of Other Spine-Related Disorders
Cauda Equina Syndrome
Multiple lumbosacral radiculopathies are encountered with some frequency. Typically, the involvement is bilateral and often asymmetrical.10,20 Most of these lesions are attributable to midline lumbar disc protrusions or lumbar canal stenosis. Characteristically, S1 and S2 roots, being the most medial of the roots supplying the lower limbs, are affected. In many patients, more extensive lumbosacral root involvement occurs; a common combination is bilateral S1 and S2 root compromise accompanied by unilateral or bilateral L5 root involvement.
The electrodiagnostic findings most commonly seen consist of a mixture of low-amplitude CMAPs and normal SNAPs on NCS, along with fibrillation potentials and MUP dropout on needle EMG (Fig. 13–10). On NEE, the abnormalities often are more severe in muscles located distal to the knees. With some substantial lesions of recent onset, they are just as prominent in the more proximal muscles. Whenever the disorder is subacute or chronic, fibrillation potentials usually are accompanied by chronic neurogenic MUP changes. Low lumbar or high sacral paraspinal fibrillation potentials often are found bilaterally with more acute lesions, but are undetectable with many chronic ones. Typically, the H responses cannot be elicited, and even the M components of the H responses, recorded from the gastrocnemius/soleus muscles, are quite low in amplitude.
Lumbar Canal Stenosis
Between these two extremes are numerous different electrodiagnostic patterns: (1) two or more radiculopathies, far more often bilateral than strictly unilateral; (2) a single radiculopathy, typically S1, that is sometimes detected in the less symptomatic or asymptomatic limb; (3) unilateral or bilateral absent H responses alone; (4) NEE changes restricted to just one or two limb muscles, most commonly those innervated by the S1 roots; or (5) fibrillation potentials limited to the paraspinal muscles.9,19
Myelopathy
In contrast, if the anterior horn cells or the intramedullary fibers derived from them are involved, the electrodiagnostic findings are those of a focal intraspinal canal lesion that are characteristically bilateral, but often asymmetric. How prominent the electrodiagnostic changes are with such focal disorders depends mainly on where the lesions are located along the spinal cord. Lesions situated in C5-T1 segments and L4-S2 segments produce substantial abnormalities on motor NCS and NEE and generally are readily recognized as intraspinal canal lesions. All such disorders result in low-amplitude CMAPs or CMAPs that cannot be elicited and normal SNAPs on NCS, accompanied by fibrillation potentials, MUP dropout, and, depending on lesion duration, chronic neurogenic MUP changes on needle EMG. Conversely, lesions involving T2 through L3 segments result only in NEE changes (i.e., motor NCS using various limb muscles as recording sites are normal). Finally, lesions involving the upper cervical cord segments (C1-4) have essentially no electrodiagnostic manifestations because that region of the spinal cord cannot be assessed.27
Cervical Root Avulsion
Root avulsions, which are usually restricted to the cervical region, differ from the typical single compressive radiculopathy principally in the degree of axon loss that results. Because the entire motor supply from one or both roots innervating the particular muscle has been disrupted, that muscle is severely or totally denervated. If it is used as a recorded muscle during motor NCS, the CMAP obtained is of very low amplitude, if it can be elicited. Similarly, during needle EMG of that muscle, fibrillation potentials are abundant, and MUPs are either absent or, if present, quite sparse and show reduced recruitment. Sensory NCS responses derived from the same roots are normal because the sensory roots are interrupted proximal to their DRG. Fibrillation potentials are not found in the appropriate paraspinal muscles in patients with cervical avulsion injuries, so their absence does not exclude this diagnosis.9
Acknowledgments
Key Points
1 Wilbourn AJ, Aminoff MJ. AAEM Minimonograph #32: The electrodiagnostic examination in patients with radiculopathies. Muscle Nerve. 1998;21:1612-1631.
2 Wilbourn AJ. Nerve conduction studies: Types, components, abnormalities, and value in localization. Neurol Clin North Am. 2002;20:305-338.
3 Shea PA, Woods WW, Werden DH. Electromyography in diagnosis of nerve root compression syndrome. Arch Neurol Psychiatry. 1950;64:93-104.
4 Woods WW, Shea PA. The value of electromyography in neurology and neurosurgery. J Neurosurg. 1951;8:595-607.
5 Yoss RE, Corbin KB, MacCarty CS, et al. Significance of symptoms and signs in localization of involved root in cervical disc protrusion. Neurology. 1957;7:673-683.
1 Wilbourn AJ. Nerve conduction studies: Types, components, abnormalities, and value in localization. Neurol Clin North Am. 2002;20:305-338.
2 Preston DC, Shapiro BE. Electromyography and Neuromuscular Disorders. Boston: Butterworth-Heinemann; 1998.
3 Wilbourn AJ, Ferrante MA. Clinical electromyography. In: Joynt RJ, Greggs RC, editors. Baker’s Clinical Neurology on CD-Rom. Philadelphia: Lippincott Williams & Wilkins, 2000.
4 Dimitru D, Amato AA, Awarts MJ. Electrodiagnostic Medicine, 2nd ed. Philadelphia: Hanley & Belfus; 2002.
5 Shea PA, Woods WW, Werden DH. Electromyography in diagnosis of nerve root compression syndrome. Arch Neurol Psychiatry. 1950;64:93-104.
6 Woods WW, Shea PA. The value of electromyography in neurology and neurosurgery. J Neurosurg. 1951;8:595-607.
7 Wilbourn AJ, Aminoff MJ. Radiculopathies. In: Brown WF, Bolton CF, editors. Clinical Electromyography. 2nd ed. Boston: Butterworth-Heinemann; 1993:177-209.
8 Wilbourn AJ. The value and limitations of the electromyographic examination in the diagnosis of lumbosacral radiculopathy. In: Hardy RW, editor. Lumbar Disc Disease. New York: Raven Press; 1982:65-109.
9 Wilbourn AJ, Aminoff MJ. AAEM Minimonograph #32: The electrodiagnostic examination in patients with radiculopathies. Muscle Nerve. 1998;21:1612-1631.
10 Raynor EM, Kleiner-Fisman G, Nardin RA. Lumbosacral and thoracic radiculopathies. In: Kitirji B, Kaminski HJ, Preston DC, et al, editors. Neuromuscular Disorders in Clinical Practice. Boston: Butterworth-Heinemann; 2002:859-883.
11 Levin KH. Radiculopathy. In: Levin KH, Luders HO, editors. Comprehensive Clinical Neurophysiology. Philadelphia: WB Saunders; 2000:189-200.
12 Johnson EW. Electrodiagnosis of radiculopathy. In: Johnson EW, editor. Practical Electromyography. 2nd ed. Baltimore: Williams & Wilkins; 1988:229-245.
13 Braddom RI, Johnson EW. Standardization of “H” reflex and diagnostic use in S1 radiculopathies. Arch Phys Med Rehabil. 1974;55:161-164.
14 Schuchmann J. H-reflex latency in radiculopathy. Arch Phys Med Rehabil. 1978;59:185-187.
15 Eisen A, Schomer D, Melmad C. An electrophysiological method for examining lumbosacral root compression. Can J Neurol Sci. 1977;4:117-123.
16 Fisher MN, Shidve AJ, Terxera C, et al. The F response—a clinically useful physiological parameter for the evaluation of radicular injury. Electromyogr Clin Neurophysiol. 1979;19:65-75.
17 Yoss RE, Corbin KB, MacCarty CS, et al. Significance of symptoms and signs in localization of involved root in cervical disc protrusion. Neurology. 1957;7:673-683.
18 Marinacci AA. A correlation between operative findings in cervical herniated disc with the EMGs and opaxuq myelograms. EMG. 1966;6:5-20.
19 Levin KH, Maggiano HJ, Wilbourn AJ. Cervical radiculopathies: Comparison of surgical and EMG localization of single-root lesions. Neurology. 1996;46:1022-1025.
20 Wilbourn AJ. The electrodiagnostic examination. In: Herkowitz HN, Garfin SR, Barlderston RA, et al, editors. The Spine. 4th ed. Philadelphia: WB Saunders; 1999:135-150.
21 Wilbourn AJ. Diabetic neuropathies. In: Brown WF, Bolton CF, editors. Clinical Electromyography. 2nd ed. Boston: Butterworth-Heinemann; 1993:447-515.
22 Levin KH. Neurological manifestations of compressive radiculopathy of the first thoracic root. Neurology. 1999;53:1149-1151.
23 Bodner RA, Levin KH, Wilbourn AJ. Lumbosacral radiculopathies: comparison of surgical and EMG localization. Muscle Nerve. 1995;18:1071.
24 Tsao BE, Levin KH, Bodner RA. Comparison of surgical and electrodiagnostic findings in single root lumbosacral radiculopathies. Muscle Nerve. 2003;27:60-64.
25 Hamanishi C, Tanaka S. Dorsal root ganglia in the lumbosacral region observed from the axial view of MIR. Spine. 1993;18:1753-1756.
26 Sato K, Kikuchi S. An anatomic study of foraminal nerve root lesions in the lumbar spine. Spine. 1993;18:2246-2251.
27 Levin KH. L5 radiculopathy with reduced superficial peroneal sensory responses: Intraspinal and extraspinal causes. Muscle Nerve. 1998;21:3-7.