14 The cerebellum and vestibular system
Introduction
It is now clear that the cerebellum and vestibular systems also play a role in the integration of sensory information that is essential for generating appropriate responses to environmental stimuli and for a variety of other functions including constructing a perception of ourselves in the universe; controlling muscle movement; maintaining balance; maintaining internal organ and blood flow functionality; maintaining cortical arousal; and developing active plasticity in neural networks which allows environmental conditioning to occur. The importance of the cerebellum in the overall function of the neuraxis is demonstrated by the fact that there are approximately 20 million corticopontocerebellar fibres projecting between the cerebellum and the cortex, compared to only about 1 million corticospinal fibres supplying the cortical output to the voluntary muscles of the body. The integration function of the cerebellum is evident, as the input-to-output or afferent-to-efferent ratio in the cerebellum is approximately 40:1.
Anatomy of the cerebellum
The cerebellum lies behind the pons and medulla in the posterior cranial fossa (Fig. 14.1). It is separated from the cerebrum by an extension of dura mater, the tentorium cerebelli, and from the pons and medulla by the fourth ventricle (Fig. 14.2). It is somewhat smaller than the cerebrum but this difference varies with age, being 1/8 the size of the adult cortex but only 1/20 the size of the infant cortex.
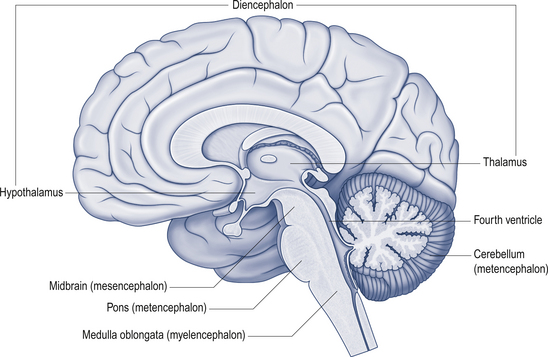
Figure 14.2 The relationship of the cerebellum to the cortex.
(from Drake et al. 2010. Gray’s Anatomy for Students 2nd edn. Churchill Livingstone, with permission)
The cerebellum is derived from the rhombencephalon, along with its homologues the pons and medulla, and is connected to the brainstem via three peduncles. These peduncles, together with the anterior and posterior medullary velum, are the main routes of entry or exit from the cerebellum. The inferior cerebellar peduncle, also known as the restiform body, conveys a number of axon projections into the cerebellum including (Figs 14.3 and 14.4):
1. The posterior spinocerebellar tract, which contains mossy fibres from the spinal cord that project to the cortex of the spinocerebellum;
2. The accessory cuneocerebellar tract, arising from the dorsal external arcuate fibres from the accessory cuneate nucleus;
3. The olivocerebellar tract, which contains climbing fibres of the contralateral inferior olivary nucleus;
4. The reticular cerebellar tract, which is formed from the ventral external arcuate fibres carrying projections from the arcuate and lateral reticular nucleus of the medulla; and
5. The vestibulocerebellar tract, which is formed from the projection fibres of the vestibular nuclei.
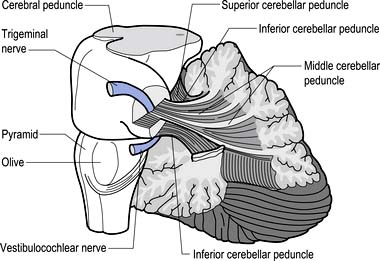
Figure 14.3 The anatomical relationships of the inferior, middle, and superior cerebellar peduncles.
The middle cerebellar peduncle or brachium pontis is the largest of the cerebellar peduncles, and contains the massive afferent corticopontocerebellar pathways. The middle peduncle is composed of axon projection fibres from the pontine nuclei to the contralateral cerebellum (Figs 14.3 and 14.4). The primary projections from the cerebral cortex to the cerebellum include premotor and supplementary motor areas (area 6), primary motor areas (area 4), primary sensory areas (areas 3, 1, and 2), and association and limbic cortices.
1. The dentatorubral tract, which projects from the dentate nucleus of the cerebellum to the opposite red nucleus in the mesencephalon;
2. The dentatothalamic tract, which projects to the contralateral thalamus;
3. The uncinate fasciculus, which contains fibres from the fastigial nucleus en route to the lateral vestibular nuclei in the medulla (Figs 14.3 and 14.4); and
4. Aminergic afferent projections, including noradrenergic, dopaminergic, and serotonergic afferents projecting to all areas of the cerebellum.
Noradrenergic neurons in the locus ceruleus project to Purkinje dendrites in the molecular layer and with granule cells in the granular layer (Bloom et al. 1971; Kimoto et al. 1981). Dopaminergic fibres arising from the neurons in the ventral mesencephalic tegmentum project to the Purkinje and granule neurons of interposed and lateral cerebellar nuclei (Simon et al. 1979). Serotonergic afferent fibres arise from the raphe nuclei of the brainstem and terminate in both the molecular and granule layers (Takeuchi et al. 1982).
The cerebellar surface is a striking example of natural economics in that it contains parallel convolutions or folia running in a transverse direction on the surface of the cerebellum that increase the surface area of the cerebellar cortex and give the cerebellum a tree-like appearance (Fig. 14.6). There are three primary lobes, anterior, posterior, and flocculonodular, on the cerebellar surface which are further dived into ten lobules. The cerebellum can be divided into nine regions along the vermis, which is a small unpaired structure in the median portion of the cerebellum separating the two large lateral masses. These nine regions are listed in Table 14.1 from anterior to posterior (see also Fig. 14.7). There are two major fissures that divide the cerebellum into three main lobes, and a number of other fissures that divide each lobe into its respective lobules.
Embryological development of the cerebellum
At the same time, the primary fissure begins to cut into the surface of the cerebellum, separating the anterior from the posterior lobe and other smaller fissures develop on the inferior surface.
The archicerebellum is the first region to appear in phylogeny and comprises the flocculi, their peduncles, the nodulus, and the lingula. The archicerebellum is the oldest and most medial portion of the cerebellum. In humans, archicerebellum contributes to the vestibulocerebellum, which comprises the flocculonodular lobe and the lingula (see Fig. 14.7) (Brodal 1981). As its new name would indicate, the vestibulocerebellum is the region of the cerebellum that communicates most intimately with the vestibular system. In fact, the vestibular nuclei of the brainstem share similar relationships with the cortex of the archicerebellum as the deep cerebellar nuclei share with the cortex of the palaeo- and neocerebellum. They therefore serve functionally as a cerebellar nuclear complex. The vestibulocerebellum also contains the only cerebellar cortical cells that leave the body of the cerebellum before synapsing. In the other regions of the cerebellum, the output cells of the cerebellar cortex synapse on neurons of the deep cerebellar nuclei. The stimuli from these neurons evoke inhibitory postsynaptic potentials (IPSPs) in the deep cerebellar nuclei.
Phylogenically, the palaeocerebellum is next to develop. Apart from the lingula, it comprises the anterior lobe, the pyramid, and uvula of the posterior vermis. This separated the archicerebellum into two parts, the lingula anteriorly and the flocculonodulus posteriorly (Brodal 1981). The palaeocerebellum contributes to the spinocerebellum, which is involved in a variety of parameters associated with movement.
The layers of the cerebellar cortex
The next layer, the Purkinje layer, consists of the only output fibres of the cerebellar cortex, the Purkinje cells. The dendrites of Purkinje cells project outwards to the molecular layer. Purkinje cells have recurrent collaterals that inhibit adjacent Purkinje cells and Golgi type II neurons.
Cerebellar cortex is composed of 3 layers and 5 cell types
The cellular interactions of the cortical cells result in one inhibitory output tract, from Purkinje cells which synapses on the deep cerebellar and vestibular nuclei. There are two main input tracts to the cerebellum, each of which is excitatory. The two input tracts consist of climbing fibres, which are actually the axons of neurons which reside in the contralateral inferior olive, and the mossy fibres, which are axons of neurons of a variety of pontomedullary reticular nuclei and axons of neurons in laminae VI and VII of the spinal cord (Ito 1984). As discussed earlier, aminergic neurons in the brainstem also project to the cerebellum.
The climbing fibres first give off collateral projections to the deep cerebellar nuclei before synapsing on granule, Golgi, basket, and Purkinje cells in the cerebellar cortex (Gilman et al. 1981; Van der Want et al. 1989). Only one synapse per Purkinje cell occurs; however, many Purkinje cells are innervated by a single climbing fibre so that a single climbing fibre spike produces a burst of Purkinje cell activity. The mossy fibres influence the Purkinje activity indirectly via synapses on granule cells. The parallel fibres of the granule cells in turn synapse on the Purkinje cells directly or via basket or stellate interneurons. Each parallel fibre excites a long array of about 500 Purkinje neurons, whereas each Purkinje neuron receives input from approximately 200 000 parallel fibres (Gilman 1992) (Fig. 14.12).
All of the cerebellar neurons of the cortex are inhibitory except the granule cells. The Purkinje inhibitory output is exerted on the spontaneously active nuclear cells. Thus, the nuclear cells must have a strong ’pacemaker’ potential or a powerful excitatory input to match the inhibition resulting from the Purkinje cells. The latter excitatory input may be manifested in excitatory impulses from axon collaterals of mossy and climbing fibres that each give off before synapsing in the cerebellar cortex.
Functional regions of the cerebellum
Fastigial efferent fibres can be crossed and uncrossed. The crossed fibres project via the uncinate fasciculus of the superior cerebellar peduncle to the super colliculus bilaterally, and to the interstitial nucleus of Cajal (Ito 1984). Some fibres also project to the ventral lateral and ventral posterior lateral nuclei of the thalamus via the superior cerebellar peduncle. The uncrossed fibres form the fastigiobulbar projections whose bulk forms the smaller juxtarestiform body. These fibres project to all four of the ipsilateral vestibular nuclei and the ipsilateral reticular formation (Batton et al. 1977). The fastigial nuclei fire after the commencement of movement. This nucleus receives large inputs from the periphery and sends few projections to the motor cortex. The fastigial nucleus is involved in the feedback mechanisms of the cerebellum.
The second component originates in the intermediate areas of the cerebellar hemispheres and projects to the interposed nuclei. Some neurons from the interpositus nuclei project through the superior peduncles to synapse on magnocellular neurons of the contralateral red nucleus (Asanuma et al. 1983). The majority, however, synapse on neurons of the contralateral thalamic nuclei of the ventral lateral pars caudalis and ventral posterolateral pars oralis. These thalamic neurons fire almost simultaneously to the primary motor cortex and are involved in efferent copy mechanisms. Efferent copy mechanism compares the intended programme from the cortex to the cerebellum’s knowledge of the state of the organism. Corrections are sent to the brain prior to the movement being carried out. This minimises the time delay in regulating evolving movements in a changing environment.
The inability to carry out a motor programme because of apparent weakness in muscles may in fact be due to a disturbance in midline cerebellar function. An example can illustrate the concept. As an arm or leg is raised or moved away from the body a greater demand is placed on postural mechanisms, which then may fail because of poor reinforcement by medial descending motor pathways from the brainstem and cerebellum.
The third component originates in the lateral cortical areas and projects to the dentate nuclei. The axons of neurons of the dentate nucleus project through the brachium conjunctivum to the contralateral red nucleus where they terminate on the parvocellular neurons in the rostral third of the nucleus (Gilman 1992) as well as via the ventral lateral and ventral anterior nuclei of the thalamus. The dentate nucleus is more heavily activated in tasks requiring the conscious evaluation of sensory information, for example, tasks requiring processing of sensory input to solve or programme complex spatial and temporal motor programmes. The dentate nucleus receives little input from the periphery and is involved in feedforward responses. The feedforward mechanisms are important for fast movements.
The fastigial and vestibular projections control the proximal limb muscles mostly through an excitatory action on proximal extensor muscles. The interposed nuclei control limb movements of the upper and lower extremities via the rubrospinal tract and through ascending fibres that project to motor cortex via the ventral posterior lateral nucleus of the thalamus. The dentate nuclei mainly project to the motor cortex via the ventral lateral and ventral anterior nuclei of the thalamus (Figs 14.14 and 14.15).
The function of the cerebellum may best be described if the various interacting components are considered as a circuit. The cerebellar circuit consists of a system of interconnecting brain parts that transform and combine messages on intent and the results of those actions into an optional set of instructions for motor execution appropriate at that time. Thus the cerebellum may be described as an implementer of higher brain functions which detects the variation between the programme demands and the actual muscular actions. In order to accomplish this, the cerebellum requires a feedforward projection map of the intended actions which emanates from association cortex or other higher centres. This feedback most probably arises via coactivation of descending pathways to alpha motor neurons, and through conventional feedback mechanisms.
During learning of new tasks, feedback input is utilised first until the dentate and lateral cerebellum can begin firing to promote feedforward processes. In other words, we learn by trial and error. This is why during the learning phase our execution of tasks is slower and it takes more conscious effort to perform the task. The cerebellum provides a signal to the brain that promotes the closest learned response and this is constantly updated, based on judgement of degree of error and evolving changes in the environment, posture, etc. (Fig. 14.16).
Cerebellar lesions
1. Muscles that normally act together lose the capacity to do so, with muscles contracting out of sequence to perform the desired movement.
2. There are new errors in force, velocity, and timing with loss of the ability to hit a target.
3. There are disturbances in weight discrimination, which is thought to be the only sensory disturbance in cerebellar disease; however, it is now understood that cerebellar deficits can result in global sensory processing disturbances or distortions in sensory awareness because of alterations in cerebellar projections to the central lateral nuclei and other non-specific nuclei of the thalamus.
4. Cerebellar lesions may also clearly result in disturbances of spatial orientation and sensory perception of motion because of intimate connections with the vestibular system.
Cerebellar influences on eye movements
The cerebellum is involved in two basic operations involving eye control. The first involves its role in both real-time positional eye control with respect to visual acquisition and the second involves long-term adaptive control mechanisms regulating the oculomotor system (Leigh & Zee 1991). The cerebellum functions to ensure that the movements of the eye are appropriate for the stimulation that they are receiving. The flocculus of the vestibulocerebellum contains Purkinje cells that discharge in relation to the velocity of eye movements during smooth pursuit tracking, with the head either stationary or moving. For example, you can keep your head still and fixate your gaze on a moving object, in which case your eyes should smoothly follow the object across your visual field, or you could keep your eyes fixed on a stationary object and rotate your head, in which case your eyes should still smoothly track in the opposite direction and at the same speed as the rotation of your head to maintain the target in focus (Zee et al. 1981). Other neurons discharge during saccadic eye movement in relation to the position of the eye in the orbit. Individual control of eye movement is accomplished for the most part by the contralateral cerebellum although intimate bilateral integration is also important. For example, the smoothness of pursuit activity and the return to centre function of saccadic movement of the right eye are under left cerebellar modulatory control.
Lesions of the dorsal vermis and fastigial nucleus of the cerebellum result in saccadic dysmetria, especially hypermetria of centripetal saccades (Optican & Robinson 1980). Lesions of the flocculus result in a variety of eye movement dysfunctions including (Berthoz & Melvil-Jones 1985; Optican et al. 1986):
The vestibular system
Peripheral vestibular system
The function of the semicircular canals is to detect rotational movements of the head. The separate canals are orientated in different planes so as to detect the different directions of movement (Fig. 14.17). They are paired with a canal from the opposite side, that is the horizontal canals, the right anterior/left posterior, and the left anterior/right posterior are paired so as to detect movement in their respective planes. Rotating the head horizontally to the right increases the rate of firing of receptors in the right horizontal canal, and decreases the rate of firing in the left. Similar paired responses are produced in the other opposing canals when they are moved in their planes. The canals are surrounded by a thin layer of fluid called perilymph, which is essentially the same composition as cerebrospinal fluid that cushions them from the surrounding bony labyrinth. They are filled with another fluid referred to as endolymph, which is very high in potassium. The horizontal canal is orientated 30° from the horizontal in the neutral position and is thus in the vertical plane when the head is tilted backwards by about 60°.
Each canal has an expansion called the ampulla, which contains a specialised structure called the cupola and hair cells (Fig. 14.17). The receptors on the hair cells are polarised to respond to movement in one direction by firing, and to hyperpolarise (decrease firing) with movement in the other, with respect to the single kinocilium present on the hair cell (Fig. 14.18). As the head rotates, the fluid within the canal stays still due to inertia. This exerts pressure on the cupola in the ampulla, distorting it and its associated hair cells, either increasing or decreasing the rate of firing, depending on the direction of movement.
The otolithic organs are designed to detect linear or translational movements. The utricle is orientated approximately horizontally and detects lateral and anterior/posterior linear movements. The saccule is vertically orientated and detects inferior/superior (vertical) and anterior/posterior linear movements. They consist of hair cells with their stereo and kinocilia embedded in a gelatinous membrane. Embedded in the top of this membrane are otoliths. During linear movements of the head, the inertia of these otoliths causes the cilia on the hair cells to be distorted, thus increasing or decreasing the firing rate of the hair cells, depending on the direction of movement. Sometimes the otoliths can become dislodged, usually due to trauma or degeneration of the gelatinous membrane. If they become trapped in the semicircular canals they will produce the condition known as benign paroxysmal positional vertigo.
The central vestibular system
The vestibular nuclei receive afferent information from a variety of sources including:
1. The vestibular apparatus, which includes the semicircular canals and otolithic organs and vestibular ganglia;
2. Proprioceptive information, which is relayed to the cerebellum via the climbing fibres which arise in Clark’s column of the spinal cord;
3. Visual information from the striate cortex, the superior colliculus, and lateral geniculate nucleus of the thalamus;
4. Tactile information, which projects from the thalamus and somatosensory cortex; and
5. Auditory information from the inferior colliculus and medial geniculate body of the thalamus.
The vestibular projections from cranial nerve VIII travel primarily to the vestibular nuclei and the flocculonodular lobe of the cerebellum. There are four vestibular nuclei on each side located at the pontomedullary junction in the floor of the fourth ventricle, just inferior and medial to the inferior cere-bellar peduncles (Fig. 14.19). They are the:
Projections from the medial vestibular nucleus ascend in the medial longitudinal fasciculus to synapse in the ipsilateral abducent, and contralateral trochlear, and oculomotor nuclei. Descending projections from the medial vestibular nucleus form the medial vestibulospinal tract, which descends in the anterior spinal cord and synapses on ventral horn neurons in the cervical and thoracic spinal cord areas. These projections probably do not reach the lumbar areas of the cord and thus are involved with the postural corrections of neck and upper limb muscles exclusively (Fig. 14.20).
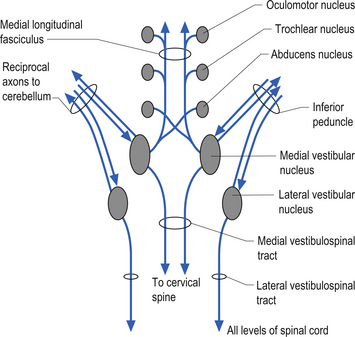
Figure 14.20 The functional projections between the vestibular nuclei and other structures of the brainstem and cerebellum.
Projections from the lateral vestibular nuclei descend in the lateral vestibulospinal tracts to all levels of the cord (Fig. 14.20). It is thought this tract is involved in postural responses.
The integration system is composed of a complex array of projection systems involving (see Fig. 14.19):
The subcortical output projections of the vestibular system
The output mechanisms are also complex and wide ranging and may include:
1. Ocular movements controlled through areas of the brainstem and cortex;
2. Control of axial musculature via the lateral vestibular nucleus, the descending medial longitudinal fasciculus, and the reticulospinal tract (these tracts combine to provide the appropriate amount of inhibition and excitation to ventral horn neurons to provide postural stability);
3. Autonomic nervous system via fastigial nuclear and vestibular nuclear projections to the pontomedullary reticular nuclei including the nucleus tractus solitarius, which controls vagus nerve activity and;
4. Emotional components mediated via cerebellar-limbic projections (Brodal 1981; Robinson et al. 1994; Wessel et al. 1998).
Cortical projections of the vestibular system
The vestibular systems projects bilaterally to the cerebral cortex, more so to the non-dominant cortex (usually the right). The vestibular cortex is thought to be located at the junction of the parietal and insular cortices (Brodman’s areas 3a in the central sulcus and 7) (Dieterich & Brandt 2008). It is thought the contralateral vestibular information ascends multisynaptically to the thalamus, and ipsilaterally through an uncrossed pathway that runs adjacent to or in the medial lemniscus (Zwergal et al. 2008).
The functions of the vestibular system
The functions of the vestibular system include:
1. The sensation and perception of position and motion—The vestibular system detects the position of the head only. In order to function appropriately, this information must also be integrated with information depicting the orientation of the head to the rest of the body. This is accomplished by the vestibulo-cervical reflexes, which integrate the information transmitted by the joint receptors, tendon organs, and muscle spindles of the muscles and joints of the neck in relation to vestibular activation. For example, when you bend your head and neck to the left, the semicircular canals and the proprioceptors of the neck both fire, giving you the perception that you are bending your neck to the left but the rest of your body is stationary. However, if your entire body falls to the left, only your semicircular canals would fire, giving you the perception that your whole body is falling to the left.
2. Orientation of the head and body to the vertical via the eye-righting reflexes—The eyes maintain a parallel with the horizon despite deviations of the head. Visual clues are also important in postural and balance control. People with severe lesions to their vestibular systems can still maintain very good balance and posture until they close their eyes. When the visual cues are absent they become disoriented and fall almost immediately.
3. Dynamic and static positioning of the body’s centre of mass—For example, if you are suddenly and unexpectedly pushed to the right, your right leg extensor muscles and left paraspinal muscles will contract so you do not fall to the right. All this happens before you perceive that you have been pushed.
4. Head stabilisation during body movements (Horak & Shupert 1999)—The head is a relatively heavy object supported by a flexible narrow structure. The position of the head during movement is reflexively controlled to support the changing centre of gravity of the body.
5. Stabilisation of the eyes while the head is moving—In order to focus on a target while the head is in motion a reflex feedback mechanism is necessary. The vestibulo-ocular reflex sends information to the extraocular muscles that cause them to move the eyes in an equal and opposite direction to that of the head.
Vestibulo-ocular reflexes
As described above, stabilisation of gaze during head movements is one of the main roles of the vestibular system. It accomplishes this through vestibulo-ocular reflexes. The semicircular canals of the ear are associated with certain eye muscles, such that increased firing of a particular canal results in increased excitability of the given muscles. They are, as such (Brodsky et al. 2006):
1. The horizontal canals activate the ipsilateral medial rectus and contralateral lateral rectus. Thus horizontal canal activation results in movement of the eyes to the opposite side.
2. The posterior canals activate the ipsilateral superior oblique and contralateral inferior rectus muscle. Thus the posterior canals drive the eyes down to the opposite side.
3. The anterior canals activate the ipsilateral superior rectus and the contralateral inferior oblique. Thus the anterior canals drive the eyes up to the same side.
Otolithic ocular reflexes are more complex. Generally, they are responsible for convergence/divergence eye movements in the lateral and anterior/posterior plane, and for ocular counter-rolling movements. The purpose of the ocular counter-roll is to keep the eyes vertically aligned when the head rolls to the side (such as is lateral flexion of the neck). In this situation the eye to the side of the roll intorts (the 12 o’clock position of the eye rolls toward the midline) and the eye to the side opposite the roll extorts (the 12 o’clock position of the eye rolls away from the midline). It is worth noting the superior muscles of the eye act as intortors, and the inferior muscles as extortors (Brodsky et al. 2006) (Table 14.2).
Afferent stimulus to the vestibular system can be accomplished through a variety of mechanisms
1. Large moving visual scenes (Lestienne et al. 1977);
2. Electrical stimulation of the vestibular nerve (Nashner & Wolfson 1974);
3. Vibrations of tendons and muscles in the extremities (Lackner 1978);
4. Altering support surfaces and environmental stimuli (Shumway-Cook & Horak 1986);
5. Translating or rotating platforms (Nashner 1977; Keshner 1999); and
6. Vertically dropping subjects (Melvill-Jones & Watt 1971; Greenwood & Hopkins 1976).
Vestibulo-autonomic reflexes
Being the earliest to arise in evolutionary history and embryological development, the vestibulocerebellum serves the most primitive function of the cerebellum. It receives extensive inputs from sensory receptors throughout the head and body that provide us with spatial coordinates for the purpose of spatial orientation and self-awareness. This includes:
1. Information from the retina and advanced visual-processing systems of the brain;
2. Information from auditory and vestibular neurons via monosynaptic and polysynaptic connections;
3. Information from the inner ear; and
4. Muscle and joint receptors, particularly from the spine via the vestibular nuclei.
Assessment of the vestibular system
1. Assessment of posture—Head tilts and certain postural reactions can be due to altered vestibulo-ocular and vestibulo-spinal reflexes. In lesions of the graviceptive pathways from the otoliths and semicircular canals, patients may present with ocular tilt reactions which involves head tilt, skew deviation, and ocular torsion (Brodsky et al. 2006).
2. Stabilometry—The practitioner assesses the patient’s ability to stand unaided with eyes open and closed. By closing the eyes, the practitioner forces that patient to rely on vestibular and proprioceptive information to determine balance. If either of those systems are defective, the patient should experience noticeably greater difficulty staying upright and may fall (mildly increased sway is normal). If their balance improves with the eyes closed, they may have a visually mediated balance problem. By having the patient stand on a compliant surface, such as a block of high density foam, proprioceptive information is reduced, and the patient further must rely on vestibular information. Stabilometry may be done with force plates to further measure and quantify postural and balance changes.
3. Stepping test of Fukuda (Fukuda 1953) —The practitioner has the patient march on the spot for 50 steps with the eyes closed. The patient will usually deviate to the side of vestibular hypofunction. More than 30° rotation is considered a positive test.
4. Headthrust test—The patient is asked to look at a point in the distance in front of them. The practitioner then quickly moves the head to the side. The vestibulo-ocular (VOR) reflex should keep the eyes on the target straight ahead. A deficiency of the VOR will mean the eyes travel with the head and the patient must make a quick saccade eye movement back to the target.
5. Nystagmus—Nystagmus can be due to many causes, but in vestibular dysfunction a discrepancy in the tonic vestibular discharge will result in the eyes being driven relatively slowly to the side of lower vestibular output. This will then result in a quick saccade eye movement back to the target. Nystagmus is named according to the direction of the fast movement. Normally, vestibular lesions result in decreased vestibular output; hence the nystagmus is usually away from the lesion side. In cases of subtle nystagmus, it can be best seen by viewing the movement of the blood vessels in the retina with an ophthalmoscope. Nystagmus due to peripheral lesions can usually be reduced with having the patient fixate their vision on something. Classically, this does not occur with central vestibular lesions. Hence the ability to fixate should be removed through Freznal or video-electronystagmography goggles, or by having the patient cover their other eye when using the ophthalmoscope.
6. Dix Hallpike test—This is a test for Benign Positional Paroxysmal Vertigo (BPPV). The test is designed to move any loose otoliths in the posterior semicircular canals, and thereby induce vertigo and nystagmus. The nystagmus should be toward the affected ear, normally down toward the floor. Steps A to C of Fig. 14.21 demonstrate the Dix Hallpike test for the right posterior canal.
Testing for cerebellar dysfunction
A variety of tests have been developed to test cerebellar function (also see Chapter 4). A selection of these tests includes the following:
1. Walk in tandem, on heels, on toes; and backwards.
2. Accentuate dysmetria by increasing inertial load of limb, which may result in overshooting and undershooting of targets.
3. Rapidly alternating finger opposition, pronation, and supination of the elbow, and heel/toe floor tapping are all tests designed to expose dysdiadochokinesia, which is the inability to perform rapidly alternating movements with consistency and coordination.
4. The performance of finger to nose, toe to finger, heel to shin, and figure-of-eight with the foot are all tests to disclose kinetic tremor and dysmetria.
• Saccadic dysmetria (hypermetric) and macrosaccadic oscillations;
• Glissadic, postsaccadic drift;
• Disturbance in adjusting the gain of the VOR;
Common vestibular disorders
Menière’s disease
Migraine
Migraine-associated dizziness occurs with a prevalence of 6.5%, and can occur in conjunction with a headache or in isolation (not associated with a headache) (Furman & Whitney 2000). Diagnosis of this condition may be difficult because it is still largely a diagnosis of exclusion. This should be considered in all cases of dizziness associated with headache and without hearing loss.
References
Fukuda T. Statokinetic Reflexes in Equilibrium and Movement. Tokyo: Tokyo University Press, 1983.
Furman J.M., Whitney S.L. Central causes of dizziness. Phys. Ther.. 2000;80(2):179-187.
Gilman S., Bloedel J., Lechtenberg R. Disorders of the Cerebellum. Philadelphia, PA: Davis, 1981.
Ito M. The Cerebellum and Neural Control. New York: Raven Press, 1984.
Leigh R.J., Zee D.S. The neurology of eye movements, second ed. Philadelphia: Davis, 1991.
Uemura T. Neuro-otological Examination. Baltimore: University Park Press, 1977.