Chapter 1
The Airways and Alveoli
After reading this chapter, you will be able to:
• Differentiate between the structures of the upper and lower airways
• Describe how the upper and lower airways differ in their ability to filter, humidify, and warm inspired gas
• List the goals of artificial airway humidification when natural humidification mechanisms are bypassed
• Describe what keeps the large cartilaginous airways and small noncartilaginous airways patent
• Explain why the larger upper airways normally present more resistance to airflow than the smaller lower airways
• Identify the difference between conducting airways and the respiratory zones of the lung
• Describe how the various lung clearance mechanisms function and interact
• List the optimal conditions for effective mucociliary lung clearance
• Explain the way in which various abnormal physiological processes impair the effectiveness of lung clearance mechanisms
The Airways
Upper Airways
The upper airways consist of the nose, oral cavity, pharynx, and larynx (Figure 1-1). The larynx marks the transition between the upper and lower airways.
Nose
The cartilaginous anterior portion of the nasal septum divides the nasal cavity into two channels called nasal fossae. The vomer and ethmoid bones form the posterior septum (Figure 1-2). The two nasal fossae lead posteriorly into a common chamber (the nasopharynx) through openings called choanae. The nasal septum is often deflected to one side or the other, more often to the left than the right,1 possibly making the passage of a catheter or artificial airway through this side difficult. Three downward-sloping, scroll-shaped bones called conchae project from the lateral walls of the nasal cavity toward the nasal septum. The conchae create three irregular passages—the superior meatus, middle meatus, and inferior meatus (Figure 1-3). Because they create turbulence, the conchae are also called turbinates. The convoluted design of the turbinates greatly increases the surface area of the nasal cavity. The maxillary bone forms the anterior three fourths of the nasal cavity floor, called the hard palate (see Figure 1-3). Cartilaginous structures form the posterior fourth, called the soft palate. Palatal muscles close the posterior openings of the nasal cavities during swallowing or coughing, isolating the nasal cavities from the oral cavity.
Squamous, nonciliated epithelium lines the anterior third of the nose; pseudostratified, ciliated columnar epithelium interspersed with many mucus-secreting glands covers the posterior two thirds, including the turbinates. This mucus-secreting epithelium is called the respiratory mucosa. Immediately under the mucosa is an extensive capillary network adjoining a system of still deeper, high-capacity vessels. These deep vessels can dilate or constrict and change the volume of blood that flows into the capillaries, altering the mucosa’s thickness. The capillaries have tiny openings or fenestrations that allow water transport to the epithelial surface. These fenestrations are not present in the capillaries of lower airways. Countercurrent blood flow and connections between arterial and venous vessels (arteriovenous anastomoses) improve the ability of the nasal mucosa to adjust the temperature and water content of inspired air. Warm arteriolar blood flows parallel with but in the opposite (countercurrent) direction of cooler blood flowing in the venules, lessening the mucosa’s heat and water-vapor loss. Arteriovenous anastomoses and countercurrent blood flow are not present in airways below the larynx.2
The main functions of the nose are the humidification, heating, and filtering of inspired air. As inspired air passes over the richly vascular epithelial surface (made larger by the presence of the turbinates), its temperature and water content increase rapidly. The turbinates disrupt the incoming airstream and create swirling, chaotic flow, increasing the chances that tiny airborne particles will collide with and adhere to the sticky mucous layer covering the nasal epithelium. Nasal secretions contain immunoglobulins and inflammatory cells, which are the first defense against inspired microorganisms. The nose is so efficient as a filter that most particles larger than 5 µm in diameter do not gain entry to the lower airways.3
The process of intubation involves the insertion of an artificial airway or endotracheal tube through the nose or mouth and into the trachea (Figure 1-4), which means the air-conditioning function of the nose is lost, and unmodified cool, dry gas directly enters the trachea. This places a heavy burden on the tracheal mucosa, which is not designed to accommodate cool, dry gases.
Pharynx
The pharynx is the space behind the nasal cavities that extends down to the larynx (see Figure 1-1). The term pharynx stems from the Greek word meaning “throat.” The nasopharynx is the portion behind the nasal cavities that extends down to the soft palate. The oropharynx, the space behind the oral cavity, is bounded by the soft palate above and the base of the tongue below. The laryngopharynx is the space below the base of the tongue and above the larynx.
As inspired gas abruptly changes its direction of flow at the posterior nasopharynx, inhaled foreign particles collide with and adhere to the sticky mucous membrane. Lymphatic tissues in the nasopharynx and oropharynx provide an immunological defense against infectious agents. These tissues include the pharyngeal (adenoid), palatine, and lingual tonsils (see Figure 1-3). These tissues may become inflamed and swollen and may interfere with nasal breathing especially in children owing to their smaller airways; chronic inflammation of the tonsils may warrant surgical removal.
The eustachian tubes, also called auditory tubes, connect the middle ear with the nasopharynx (see Figure 1-3). These tubes allow pressure equalization between the middle ear and atmosphere. Inflammation and excessive mucus production in the nasopharynx may block the eustachian tubes and hinder the pressure-equalizing process; this can momentarily impair hearing and cause pain, especially during abrupt changes in atmospheric pressure. Children younger than 3 years of age are especially susceptible to this condition because their eustachian tubes are small and easily occluded; artificial pressure-equalizing tubes, also known as myringotomy tubes, are sometimes placed through the ear’s tympanic membrane (eardrum) to create an alternate route for pressure equalization.
Deeply unconscious persons sometimes lose the pharyngeal and laryngeal reflexes and aspirate foreign material into their lungs. In such individuals, an artificial airway (endotracheal tube) with an inflatable cuff may be inserted orally or nasally through the larynx and into the trachea. After it is in place, the cuff is inflated to form a seal between the tracheal wall and tube to minimize aspiration of pharyngeal contents (see Figure 1-4). However, even if the cuff is properly inflated, pharyngeal secretions eventually migrate past the cuff into the lower airway, For this reason, mechanically ventilated patients, in whom endotracheal intubation is required, are susceptible to the development of lung infections, or ventilator-associated pneumonia (VAP); the longer the duration of mechanical ventilation, the greater the risk of VAP. Normal pharyngeal muscle tone prevents the base of the tongue from falling back and occluding the laryngopharynx, even in a person who is supine and asleep. Deep unconsciousness may relax pharyngeal muscles enough to allow the base of the tongue to rest against the posterior wall of the pharynx, occluding the upper airway; this is called soft tissue obstruction and is the most common threat to upper airway patency. If the head droops forward, the oral cavity and pharynx-larynx axis form an acute angle that may partially or completely obstruct the upper airway (Figure 1-5). Partial upper airway obstruction produces a low-pitched snoring sound as inspired air vibrates the base of the tongue against the posterior wall of the pharynx. Complete obstruction causes strong inspiratory efforts without sound or air movement. Soft tissues between the ribs and above the sternum may be sucked inward (intercostal and suprasternal retractions) as the person struggles to inhale.
Both forms of soft tissue upper airway obstruction can be easily removed by extending the neck and pulling the chin anteriorly (see Figure 1-5, C). This maneuver pulls the tongue forward out of the airway and aligns the oral and nasal cavities with the pharynx-larynx axis. This is sometimes called the sniffing position.
Pharyngeal anatomy plays a role in the incidence of obstructive sleep apnea (OSA),4 as do pharyngeal reflexes and muscle tone. The normal pharynx narrows during sleep, greatly increasing upper airway resistance. Abnormal enlargement of soft tissues can further narrow or occlude the airway, and repetitive cessations of breathing (apnea) may occur during sleep.
Larynx
The larynx lies at the level of the fourth through sixth cervical vertebrae in men and is located higher in women and children. The top portion of the larynx is a complex triangular box that is flat posteriorly and composed of an intricate network of cartilages, ligaments, and muscle (Figure 1-6). A mucous membrane continuous with the mucous membrane of the pharynx and trachea lines the interior of the larynx. Nine cartilages (three paired and three unpaired) and many muscles and ligaments form the larynx. The unpaired epiglottis is a thin, flat, leaf-shaped cartilage above the glottis. The lower end of the epiglottis (a long, narrow stem) is attached to the thyroid cartilage. From this attachment, it slants upward and posteriorly to the base of the tongue, where its upper free end is broad and rounded (see Figures 1-3 and 1-6). A vascular mucous membrane covers the epiglottis. The lower base of the tongue is attached to the upper epiglottis by folds of mucous membrane, forming a small space (the vallecula) between the epiglottis and tongue (Figure 1-7). The vallecula is an important landmark used during the insertion of a tube into the trachea (intubation).
Besides speech, the major function of the larynx is preventing the lower airway from aspirating solids and liquids during swallowing and breathing. The epiglottis does not seal the airway during swallowing.5 Instead, the upward movement of the larynx toward the base of the tongue pushes the epiglottis downward, which causes it to divert food away from the glottis and into the esophagus. The free portion of the upper epiglottis in an adult lies at the base of the tongue, but in a newborn it lies much higher, behind the soft palate. This position of the upper epiglottis helps
to account for preferential nose breathing in newborns and why it is difficult to achieve effective deposition of inhaled aerosolized medications in the lower airways of a newborn.6
The thyroid cartilage is the largest of all laryngeal cartilages, enclosing the main cavity of the larynx anteriorly (see Figure 1-6). The lower epiglottis attaches just below the notch on its inside upper anterior surface.
The cricoid cartilage, just below the thyroid, is the only complete ring of cartilage that encircles the airway in the larynx or trachea. The cricothyroid ligament connects the cricoid and thyroid cartilages (see Figure 1-6). The cricoid limits the endotracheal tube size that can pass through the larynx. The cricoid ring is the narrowest portion of the upper airway in an infant. Inside the larynx, the vocal cords lie just above the cricoid cartilage.
The membranous space between the thyroid and cricoid cartilages, the cricothyroid membrane (see Figure 1-6), is sometimes the puncture site for an emergency airway opening when structures above it are occluded. A longer term surgical opening into the airway (tracheostomy) is generally located 1 to 3 cm below the cricoid cartilage.
The remaining cartilages (arytenoid, corniculate, and cuneiform) are paired. These cartilages are in the lumen of the larynx and serve as attachments for ligaments and muscles (see Figure 1-6). The arytenoids are attachment points for vocal ligaments that stretch across the lumen of the larynx and attach to the thyroid cartilage.
The vocal folds consist of two pairs of membranes that protrude into the lumen (inner cavity) of the larynx from the lateral walls (see Figures 1-3 and 1-7). The upper pair is called the false vocal cords; the lower pair is called the true vocal cords because only these folds play a part in vocalization. The true vocal folds form a triangular opening between them that leads into the trachea below. This opening is called the rima glottidis, or glottis (see Figure 1-7).
This nerve passes downward around the aorta and returns upward to the larynx. Intrathoracic disease or surgery may injure this nerve, causing partial or complete paralysis of the vocal cords. Paralyzed vocal cords move to the midline, increasing airway resistance.7 Sensory innervation of the larynx is also supplied by the vagus nerve except for the sensory nerves of the anterior surface of the epiglottis, which are supplied by the ninth cranial (glossopharyngeal) nerve. The laryngeal reflex, which has sensory and motor components in the vagus nerve, causes the vocal cords inside the larynx to close the tracheal opening (laryngospasm). Laryngospasm occurs if anything except air enters the trachea. Drowning victims often have little water in their lungs because of laryngospasm.
Lower Airways
The lower airways (airways below the larynx) divide in a pattern known as dichotomous branching, in which each airway divides into two smaller “daughter” airways. Each division or bifurcation gives rise to a new generation of airways. The branches of the trachea and bronchi resemble an inverted tree—hence the term “tracheobronchial tree” (Figure 1-8).
Trachea and Main Bronchi
The trachea begins at the level of the sixth cervical vertebra and in an adult extends for about 11 cm to the fifth thoracic vertebra. It divides there into the right and left mainstem bronchi, one for each lung (Figure 1-9). The point of division is called the carina. Inspired air becomes 100% saturated with water vapor and is warmed to body temperature (37° C) after it passes through two or three airway subdivisions below the carina8; the point at which this occurs is known as the isothermic saturation boundary (ISB) (Figure 1-10). Above the ISB, the temperature and humidity of gas in the airways fluctuates, decreasing with inspiration and increasing with exhalation. Below the ISB, gas temperature and humidity remain constant at body temperature and 100% relative humidity. Cold air or mouth breathing moves the ISB deeper into the airways but never by more than a few generations.
The anterior (ventral) part of the trachea is formed primarily by 8 to 20 regularly spaced, rigid, horseshoe-shaped cartilages. Stretched across the open posterior ends of the tracheal cartilages, the ligamentous membrane forms a flat dorsal surface contacting the esophagus (see Figures 1-6, B, and 1-9). This membrane contains horizontally oriented smooth muscle, the trachealis. Contraction of the trachealis pulls the ends of the horseshoe-shaped cartilages closer together, slightly narrowing the trachea and making it more rigid. Rigidity of the trachea is important for preventing collapse from external pressure, especially during vigorous coughing. Coughing exerts a collapsing force only on the part of the trachea inside the thoracic cavity, the part below about the sixth tracheal cartilage.7 Above this level, the trachea is outside the thorax and not influenced by intrathoracic pressure.
At the carina, the right mainstem bronchus angles only 20 to 30 degrees away from the midline, forming a more direct continuation of the trachea than the left mainstem bronchus. The left mainstem bronchus breaks away more sharply, forming a 45- to 55-degree angle with the vertical tracheal midline (see Figure 1-9). The left bronchus is smaller in diameter than the right but twice as long.
The cartilage of the mainstem bronchi resembles the cartilage of the trachea initially, except that cartilage completely surrounds the bronchi at their entry point into the lung tissue, and the posterior membranous portion disappears. As the bronchi continue to branch, the cartilage becomes more irregular and discontinuous, no longer encircling the airway in complete sections (see Figure 1-9).
Conducting Airway Anatomy
All airways down to the level just before alveoli first appear are called conducting airways (Figure 1-11). No gas exchange between air and blood occurs across airway walls; they serve merely to conduct air to the alveoli—the gas-exchange surface of the lung. Beginning with the trachea, each of these conducting airways undergoes dichotomous branching until 23 to 27 subdivisions are formed.
The mainstem bronchi divide to form lobar bronchi, which undergo several divisions to form segmental and subsegmental bronchi. Segmental bronchial anatomy (Figure 1-12) is the basis for the application of chest physical therapy, in which a person is positioned for gravitational drainage of secretions from the various lung segments. Chest physical therapy is a respiratory therapy modality often used in treating lung diseases that produce large quantities of airway secretions.
The conducting airway subdivisions produce approximately 1 million terminal tubes at the level where alveoli (the gas-exchange units) first appear (see Figure 1-11). This enormously expansive branching gives rise to a massive increase in the cross-sectional area of the airways from the trachea (3 to 4 cm2) to the alveolar surface (approximately 50 to 100 m2—half the area of a tennis court—or about 40 times the surface area of the body).
Bronchioles are airways less than 1 mm in diameter that contain no cartilage in their walls. Their patency depends on the tethering retractile forces of the lung’s elastic parenchymal tissue. Bronchial and bronchiolar smooth muscle is oriented in a circular, spiral fashion, facilitating airway narrowing when it contracts (Figure 1-13). Strong smooth muscle contractions or spasms may nearly collapse the bronchioles, especially if disease weakens the lung’s opposing elastic tethering forces. In contrast, tracheal smooth muscle is transversely oriented between the two ends of its horseshoe-shaped cartilages (see Figures 1-6, B, and 1-9).
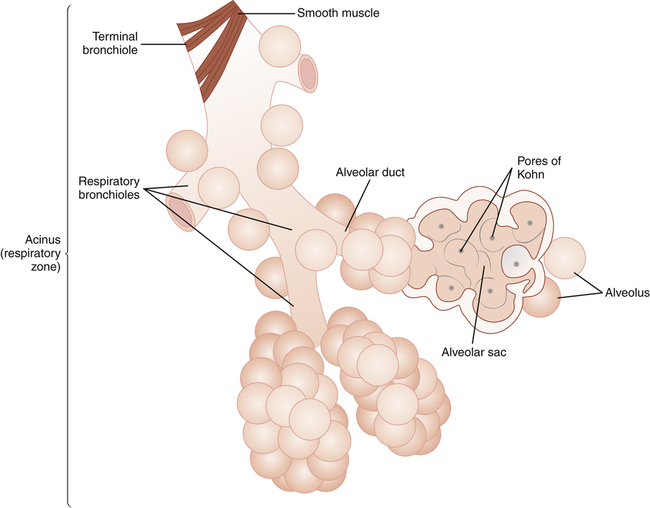
At the nineteenth or twentieth generation, the terminal bronchioles divide to form several generations of respiratory bronchioles, marking the beginning of the respiratory, or gas-exchange, zone (see Figures 1-11 and 1-13). The respiratory bronchioles are tubes containing thin, saclike pouches called alveoli in their walls. Alveoli are the gas-exchange membranes that separate air from pulmonary capillary blood. Alveolar ducts open into
blind terminal units called alveolar sacs and alveoli. The airways beyond the terminal bronchiole are collectively called the acinus, which is the functional respiratory unit of the lung (i.e., all alveoli are contained in the acinus) (see Figure 1-13). In other words, each terminal bronchiole gives rise to an acinus.
Collateral air channels called pores of Kohn connect adjacent alveoli with one another (see Figure 1-13). The canals of Lambert connect terminal bronchioles and nearby alveoli. These collateral air passages make it possible for the acinus supplied by a mucus-plugged bronchiole to receive ventilation from neighboring airways and alveoli.
Sites of Airway Resistance
Dichotomous branching of the airways through many generations creates an enormous increase in the total airway cross-sectional area. Therefore, the velocity of airflow is sharply reduced as inspired gas approaches the alveoli. Flow velocity is so low in small, distal airways that molecular diffusion is the dominant mechanism of ventilation beyond the terminal bronchioles. Airways less than 2 mm in diameter account for only about 10% of total resistance to airflow because of their huge cross-sectional area (Figure 1-14). (Table 1-1 shows the relative size of airway cross sections at different levels.) Although the resistance of a single terminal bronchiole is greater than the resistance of a single lobar bronchus, the cross-sectional area of all terminal bronchioles combined greatly exceeds the cross-sectional area of all the lobar bronchi combined. For this reason, upper airway resistance is normally much greater than lower airway resistance.
TABLE 1-1
Subdivisions of the Respiratory Tree
Generation | Name | Diameter (cm) | Length (cm) | Number per Generation | Histological Notes |
0 | Trachea | 1.8 | 12.0 | 1 | Wealth of goblet cells |
1 | Primary bronchi | 1.2 | 4.8 | 2 | Right larger than left |
2 | Lobar bronchi | 0.8 | 0.9 | 5 | 3 right, 2 left |
3 | Segmental bronchi | 0.6 | 0.8 | 19 | 10 right, 8 left |
4 | Subsegmental bronchi | 0.5 | 1.3 | 20 | — |
5 | Small bronchi | 0.4 | 1.1 | 40 | Cartilage still a component; pseudostratified ciliated columnar respiratory epithelium |
↓ | |||||
10 | 0.1 | 0.5 | 1020 | ||
11 | Bronchioles (primary and secondary) | 0.1 | 0.4 | 2050 | No cartilage; presence of smooth muscle, cilia, and goblet cells |
↓ | |||||
13 | 0.1 | 0.3 | 8190 | ||
14 | Terminal bronchioles | 0.1 | 0.2 | 16,380 | No goblet cells; presence of smooth muscle, cilia, and cuboidal cells |
15 | Respiratory bronchioles | 0.1 | 0.2 | 32,770 | |
16 | 0.1 | 0.2 | 65,540 | No smooth muscle; cuboidal cell epithelium; cilia are sparse | |
↓ | |||||
18 | 0.1 | 0.1 | 262,140 | ||
19 | Alveolar ducts | 0.05 | 0.1 | 524,290 | No cilia; cuboidal cells become flatter |
↓ | |||||
23 | 0.04 | 0.05 | 8,390,000 | ||
24 | Alveoli∗ | 244 | 238 | 300,000,000 | Squamous cells |
∗Alveolar dimensions are given in micrometers.
Modified from Weibel ER: Morphometry of the human lung. In Martin DE, Youtsey JW, editors: Respiratory anatomy and physiology, St Louis, 1988, Mosby.
Conducting Airway Histology
A mucus-secreting epithelium (mucosa) lines the lumen of the conducting airways (Figure 1-15). A basement membrane beneath the epithelium separates it from the lamina propria below, which contains smooth muscle, elastic fibers, blood vessels, and nerves. The epithelium and lamina propria constitute the respiratory mucosa. Below the mucosa is the submucosa, which contains numerous mucous glands (submucosal glands) that have ducts leading to the epithelial luminal surface. A connective tissue sheath, the adventitia, surrounds cartilaginous airways and blood vessels. This sheath ends at the bronchioles; their airway walls are in direct contact with the lung parenchyma.
The mucosal epithelium of the trachea and bronchi consists of tall, columnar, ciliated, pseudostratified epithelial cells interspersed with numerous mucus-secreting goblet cells (see Figure 1-15). The goblet cells and submucosal mucous glands secrete mucus onto the ciliated epithelial surface of the airways, forming a mucous blanket that is continually propelled upward in the direction of the pharynx. The submucosal glands contribute the greater volume of mucus; their secretion increases under the influence of parasympathetic nervous stimulation. All epithelial cells are attached to the basement membrane, but not all of them reach the airway lumen, and they appear to be stratified (see Figure 1-15)—hence the term “pseudostratified.” Epithelial cells gradually flatten and lose their cilia as they proceed from bronchi to alveoli; cartilage disappears, and goblet cells gradually decrease in number and disappear (see Figure 1-15).
Other Epithelial Cells
Other bronchial epithelial cells include basal cells, serous cells, Kulchitsky cells, brush cells, Clara cells, and intermediate (or undifferentiated) cells. Serous cells may transform to goblet cells if chronically exposed to air pollutants, including cigarette smoke. Cigarette smoke causes all mucous cells to proliferate and spread into the small bronchioles, where they are usually absent. Cigarette smoke also ultimately reduces ciliary activity. Kulchitsky cells are endocrine cells more prominent in newborns than adults and are apparently precursors of carcinoids and small cell bronchogenic carcinomas.9 Clara cells, found in the terminal and respiratory bronchioles, are nonciliated secretory cells bulging upward into the airway lumen. These cells are normally the sole source of secretions at this level because mucous cells are absent. Their secretions apparently also form part of the alveolar liquid lining. Injury to the epithelium at this level may cause the Clara cells to differentiate into ciliated or mucous cells.9
Mucociliary Clearance Mechanism
Each ciliated epithelial airway cell contains about 250 cilia beating about 1300 times per minute, moving the sheet of mucus toward the pharynx at a rate of approximately 2 cm per minute. The cilia have a rapid, forward, propulsive stroke, reaching up high into the viscous gel layer of mucus with their tips and pulling the mucous blanket up the airway. The recovery stroke is slower, and the flexible cilia bend as they are pulled backward (tips down) through the lower, less viscous sol layer of mucus (see Figure 1-15). The gel layer traps microbes and inhaled particles on its sticky surface.
Normal ciliary function and mucous composition are crucial for the effective function of this important lung clearance mechanism, often called the mucociliary escalator. It is the lung’s main method for removing microbes and inhaled particles that have gained access to the bronchial tree. The combined actions of the mucociliary mechanism, a functional glottis that prevents aspiration, and an intact cough mechanism are remarkably effective in keeping the lower airways of healthy individuals sterile.10
Importance of Humidity
Normally, the upper airways—the nose, pharynx, and trachea—heat and humidify inspired air. However, when an artificial airway such as an endotracheal tube (see Figure 1-4) is in place, these functions are completely bypassed. The addition of supplemental heat and humidity becomes critically important. The temperature of normal room air is about 22° C and has a relative humidity of about 50%, equivalent to a water vapor content of about 10 mg per liter of air (see Figure 1-10). During normal quiet breathing, inspired air warms to body temperature (37° C) and achieves 100% relative humidity soon after it passes the bifurcation of the trachea. Under these conditions, each liter of air contains about 44 mg of water vapor. As previously mentioned, this point in the airway, in the region of the subsegmental bronchi,8 is the ISB. With an endotracheal tube in place, relatively dry gas at room temperature is introduced into the trachea just above the carina, placing an unusual demand on the airway mucosa below this point, which must warm and humidify the inspired air. Consequently, the ISB moves deeper into lower airway generations. If supplemental humidity is not added to the inspired air when an endotracheal tube is in place, lower airway mucus thickens as water evaporates. The humidity deficit is the difference between the water content of room air (about 10 mg/L) and saturated body temperature gas (about 44 mg/L). Abnormally thick mucus in the lower airways hinders ciliary motion and the efficiency of the mucociliary clearance mechanism. The lung is less able to remove contaminants and becomes susceptible to infections as mucus becomes immobile and consequently builds up. If airways become completely plugged with mucus, their downstream alveoli receive no ventilation and cannot impart oxygen to the blood.
Nonepithelial Cells in the Airway
Mast cells release their inflammatory agents when activated by a process called immune sensitization, which is common in people who have certain allergies. Inhaled irritants or antigens, such as ragweed pollen, cause the plasma B cells to synthesize immunoglobulin E (IgE), which is an abnormal response to the antigen (Figure 1-16). IgE first binds to specific receptor sites on the mast cell surface, sensitizing the mast cell. The antigen combines with IgE molecules attached to the surface of the mast cell, which inactivates the antigen. However, in this process, the antigen cross-links two IgE molecules, which causes the mast cell membrane to rupture and release inflammatory agents into the airway tissues (see Figure 1-16). Histamine causes the normally tight, impermeable cell wall junctions of the airway epithelium to open, allowing it to penetrate deeply, breaking down more mast cells and creating more vascular leakage, mucosal swelling, and bronchospasm. Mast cell breakdown, airway inflammation, and subsequent bronchospasm are features of asthma, a condition characterized by chronic airway inflammation and hypersensitivity. Clinical Focus 1-7 discusses asthma and the basis for its pharmacological management.
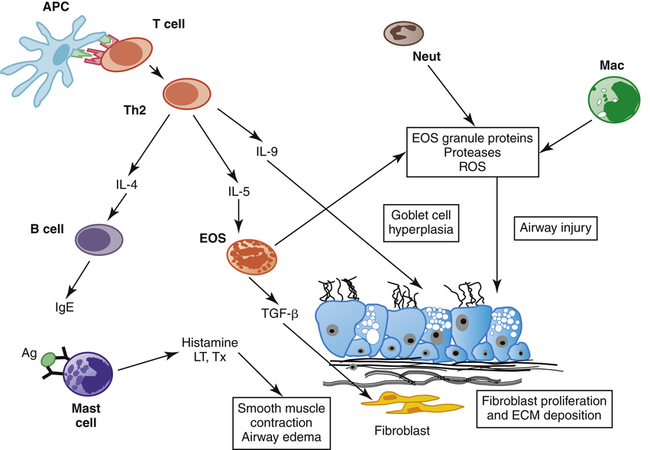
Epithelial Chloride Channel Regulation and Secretion Viscosity
Water movement into the airway lumen is an osmotic process influenced by epithelial cell membrane secretion of chloride ions.10 Chloride ions are secreted into the airway through specialized epithelial channels. Positively charged sodium ions follow the negatively charged chloride ions into the airway. Transmembrane secretion of ions provides the osmotic force for water flow into the airway lumen and plays a major role in hydrating the mucus and facilitating normal ciliary function. This mechanism is defective in cystic fibrosis (CF), a disease characterized by thick, immobile airway secretions (see Clinical Focus 1-8).
Various neurohumoral and pharmacological agents regulate epithelial chloride channels. Among these agents are those that increase intracellular concentrations of cyclic adenosine monophosphate (cAMP).10 Beta-adrenergic agonists increase intracellular cAMP levels, causing epithelial chloride channels to open and chloride secretion to increase. Increased levels of cAMP also cause smooth airway muscle relaxation and bronchodilation. Beta-adrenergic drugs, commonly administered for their bronchodilating properties, stimulate chloride secretion in normal airway cells.10 This action may account for the enhanced mucociliary clearance observed clinically when beta-adrenergic bronchodilators are administered. (Chapter 2 discusses adrenergic receptors in more detail.)
Epithelium-Derived Relaxing Factor
a substance that causes smooth muscle relaxation, called epithelium-derived relaxing factor (EpDRF). EpDRF apparently modifies the responsiveness of airway smooth muscle to various stimuli. In theory, patients with damaged or dysfunctional airway epithelium do not produce EpDRF, and the response of the smooth muscle to various stimuli goes unchecked. Therefore, the airways are hyperreactive and prone to bronchospasm. EpDRF may help regulate smooth muscle tone by modifying autonomic neural impulses.
16Antiproteases in Lung Tissues and Airway Secretions
Airway secretions and lung tissues contain inhibitors of proteolytic enzymes, known as antiproteases. In people with chronic airway inflammation (patients with CF, chronic bronchitis, asthma, or emphysema and cigarette smokers), neutrophils invade the airways and release neutrophil elastase (NE), a powerful proteolytic enzyme.17 NE is designed to destroy bacteria and other microorganisms that might be present in the airway; however, when it is chronically present, NE degrades elastin and collagen, which are major structural components of the healthy lung. Healthy people have natural antiproteases in the blood, lung tissues, and secretions, the major one being alpha1 protease inhibitor (A1PI), also known as alpha1-antitrypsin. Secretory leukoprotease inhibitor is another antiprotease found in healthy airway secretions, which, along with A1PI, protects the lung from the NE released during episodes of airway inflammation.17 However, in chronic inflammatory lung conditions, NE overwhelms the antiproteases, and lung tissue damage occurs. Balance between the proteases and antiproteases is important for normal lung function.
The Alveoli
The appearance of alveoli marks the beginning of the respiratory, or gas-exchange, zone. Alveoli first appear in the respiratory bronchioles (see Figure 1-13). The distance from the beginning to the end of the acinus is only a few millimeters, but most of the lung’s volume is contained in acinar structures (about 3000 mL at rest). As mentioned, the conducting airways contain only about 150 mL of gas. The lungs of an adult contain about 300 million alveoli, representing a gas-exchange surface area of 50 to 100 m2. Alveolar diameters range from 100 to 300 µ. Capillaries that are in contact with the alveolar membrane are only 10 to 14 µm in diameter, which is just large enough to allow the passage of red blood cells. These tiny capillaries are wrapped around the alveoli in an extremely dense network; each alveolus may be associated with up to 1000 capillary segments (Figure 1-17).
Alveolar Capillary Membrane
Alveolar epithelium has type I and type II cells (Figure 1-18). Type I cells constitute most of the alveolar surface and are extremely flat. Type II cells are compact, polygonal-shaped cells protruding into the alveolar airspace. The adjoining basement membranes of the alveolar epithelium and capillary endothelium form an extremely thin blood-air barrier, less than 0.5 µm thick in the flattest regions of the type I cell. The space between these membranes is the interstitium. The alveolar epithelium is highly permeable to respiratory gases, but the tight junctions between epithelial cells form an impermeable barrier to liquid solutions. Endothelial capillary cell junctions are loose and more permeable to water than alveolar epithelium (see Figure 1-18). Inhaled or circulating toxic agents may injure the alveolar capillary membrane, increasing its permeability, a major feature of acute respiratory distress syndrome (ARDS).
Type II Cells and Surfactant Secretion
Type II cells have short, blunt projections (microvilli) on their alveolar surfaces and contain many internal organelles, including organelles known as lamellar bodies (see Figure 1-18). The lamellar bodies are the source of alveolar surfactant phospholipid, an agent that reduces surface tension and is essential for keeping the alveoli open. (See Chapter 3 for the properties of pulmonary surfactant.)
Alveolar Macrophages and Alveolar Clearance Mechanisms
Alveolar macrophages are large migratory phagocytes wandering freely throughout the alveolar airspaces and interstitium (see Figure 1-18). Their main function is to engulf and digest microorganisms and foreign material. The alveolar macrophage is the major lung clearance mechanism distal to the terminal bronchiole.
Cigarette smoke increases phagocytosis and the release of powerful proteases by macrophages and neutrophils (proteases are enzymes that can eventually degrade and destroy surrounding cellular protein and elastic tissue).17 Although the lung normally contains protective antiprotease enzymes, these enzymes may be overwhelmed by the continual activation of alveolar macrophages and neutrophils caused by chronic smoking. A state of chronic inflammation and alveolar destruction develops, which is the primary feature of emphysema, an irreversible airway obstructive disease.