18 Stress, Strain, Speckle, and Tissue Doppler Imaging
Practical Applications
Tissue Doppler Imaging
One of the major limitations of any Doppler technique is the dependency of velocity measurements on the angle of imaging relative to the object’s direction of motion. The velocity will be underestimated by approximately 6% if the angle of interrogation is 20 degrees, 13% at 30 degrees, and 29% at 40 degrees.1 The second major limitation to TDI is its inability to distinguish between myocardial movement that is due to active contraction and myocardial movement that is due to passive tethering. These limitations have led to the development of alternate imaging techniques such as Doppler-based strain and strain rate imaging and speckle tracking.
Two major TDI techniques have been used in the assessment of ventricular function. Pulsed wave TDI (similar to routine pulsed Doppler) involves placing a defined sample volume over the area of interest. This technique has the advantage of displaying myocardial velocities in the defined small area of interest (usually <1 cm) with high temporal resolution. Alternatively, a color-coded template can be superimposed over a two-dimensional 2D or M-mode image (color TDI). This has the advantage of analyzing multiple segments and larger sample areas simultaneously. When tissue moves toward the transducer, it is color-coded red and when it moves away from the transducer it is color-coded blue (Fig. 18-2).
TDI has been found to be useful in many different clinical applications, including the following:
Evaluation of global and regional left ventricular (LV) systolic function
Assessment of LV diastolic dysfunction
Estimation of LV filling pressures
Management of heart failure and cardiac resynchronization therapy
Differentiation of constrictive pericarditis from a restrictive cardiomyopathy
Differentiation of the normal athletic heart from pathologic myocardial conditions such as hypertrophic cardiomyopathy
TDI can be used to evaluate both global and regional LV function. Mitral annular velocity has been used to estimate global LV systolic function. In a patient with normal LV function, the systolic velocity (S′ or Sa) of the mitral valve annulus is generally >6 cm/sec.2 A prior study that investigated the average mitral annular descent velocity (color-coded TDI M-mode) found that a velocity of >5.4 cm/sec predicted a LV ejection fraction of 50% or greater with a sensitivity and specificity of 88% and 97%, respectively.3 To evaluate regional systolic function, the TDI sample volume can be placed in the area of interest. Currently, color TDI is used most often to look for areas with low TDI velocities. Tissue velocities are decreased in areas where there is myocardial dysfunction or ischemia.
Tissue Doppler imaging has become a standard component of the assessment of diastolic function and the estimation of left ventricular filling pressures. The most frequently assessed regions are the lateral and medial mitral valve annulus. The lateral E′ is usually higher than the medial E′. In a normal individual, the lateral E′ is >15 cm/sec and the medial E′ is >10 cm/sec.2 As an individual ages or develops diastolic dysfunction, the E′ velocity decreases. It has been demonstrated that the ratio of E/E′ (E = mitral inflow early diastolic velocity/E′ = TDI E′) correlates well with increased pulmonary capillary wedge pressures (PCWP). An E/E′ >10 (lateral annulus) and a E/E′ >15 (medial annulus) have been shown to correlate with a PCWP greater than 20 mm Hg.4,5
Tissue Doppler imaging is a useful tool for the accurate timing of various myocardial events. This usefulness has led to its application in patients with congestive heart failure who are being evaluated for and treated with cardiac resynchronization therapy. The most commonly measured interval is the time from the onset of the QRS to the peak systolic velocity in the ejection phase (Ts). This measurement can be calculated in multiple walls and segments to assess for dyssynchrony. This topic is further discussed in Chapter 17.
TDI has also been found to be useful in the differentiation of constrictive pericarditis from a restrictive cardiomyopathy. A restrictive cardiomyopathy causes impaired myocardial function. This pathologic process leads to a decrease in the E′ velocity. In the setting of pericardial constriction, the E′ velocity is preserved as myocardial function is preserved. In a study looking at these patients, a E′ >8 cm/sec had a high sensitivity and specificity for the diagnosis of constrictive pericarditis (95% sensitivity, 96% specificity).6
Finally, TDI has been shown in several studies to be helpful in separating the athletic heart from other conditions such as hypertrophic cardiomyopathy. In patients with hypertrophic cardiomyopathy, the S′ and E′ velocities are reduced compared to normal individuals.7 Patients with the athletic heart have preserved myocardial tissue Doppler imaging velocities.
Strain and Strain Rate Imaging
Following electrical stimulation, the myocardium deforms in a three-dimensional fashion due to sarcomeric shortening. There is longitudinal shortening, radial thickening, and circumferential shortening. Strain is a dimensionless measure of this deformation and is generally expressed as a percentage. Strain (ε) is defined as the deformation (change in length) of an object normalized to its original length. Lengthening is represented by positive values and shortening by negative values (Fig. 18-3).
Strain rate imaging is defined as the speed at which deformation (change in length) occurs and is expressed in seconds–1. Within a region of interest, the strain rate is calculated as the instantaneous difference in velocity between two points relative to the distance between the points (ν1 distal and ν2 proximal). A difference between two point velocities indicates that there is movement relative to one another, either compression or expansion (Fig. 18-4).
Strain Rate and Strain: A Step-by-Step Approach
Both strain and strain rate imaging have the ability to overcome the problem of separating translational motion/tethering from true myocardial contraction, because they look at myocardial deformation rather than just motion. However, this Doppler-based technique is still angle dependent, which limits its application to many myocardial segments. Strain techniques also are disadvantaged by their vulnerability to many technical difficulties such as artifacts, noise incorporation, aliasing, and dropouts. Because of these challenges, better-than-average image quality at high frame rates is required to do accurate analysis (Fig. 18-5).
There have been several practical and research applications of strain and strain rate imaging:
Detection of myocardial ischemia and evaluation of regional function
Assessment of myocardial viability
Assessment and evaluation of cardiomyopathies
Management of heart failure and cardiac resynchronization therapy
Strain and strain rate imaging have been used to detect and identify areas of myocardial ischemia. As anticipated, during ischemia the maximal strain and strain rate signals are decreased, and there is a delay in the onset of myocardial motion.8,9 In addition, there is a time delay from systole to diastolic relaxation in ischemic myocardium.10 Although strain and strain rate imaging seems to improve the sensitivity for detecting myocardial ischemia, their overall impact on clinical and diagnostic outcomes is less clear. Clearly there are technical challenges limiting their use in exercise stress echocardiography. There may be a role for their use in dobutamine stress echocardiography, but this is still being defined.
Strain and strain rate imaging have been evaluated for their ability to assess myocardial viability. A phenomenon called post-systolic shortening (late myocardial contraction occurring after aortic valve closure) has been found to be present in viable myocardium. Also, during low-dose dobutamine echocardiography, an increase in the peak systolic strain rate was found to be helpful in assessing the degree of myocardial viability.11
There is some evidence that strain imaging may be helpful in the evaluation of patients with a possible cardiomyopathy such as hypertrophic cardiomyopathy. Patients who have myocardial thickening due to an athletic heart have normal TDI and strain parameters. All strain parameters (longitudinal, radial, and circumferential) are abnormal in patients with hypertrophic cardiomyopathy. This appears to be true even in patients with apparently normal LV systolic function.12 There is early evidence that strain parameters may help to differentiate between the different types of cardiomyopathy.
Strain imaging has been found to have some utility in the management of patients with heart failure. Again this tool is being used for its ability to accurately time myocardial events. The time to peak negative strain/strain rate has been used to help predict a patient’s response to cardiac resynchronization therapy. This topic is further discussed in Chapter 17.
Speckle Tracking Echocardiography
The potential future clinical applications of speckle tracking imaging are yet to be defined and currently are an area of active research. It has been shown that the rate of early diastolic untwist correlates with the degree of diastolic dysfunction.13 This correlation has been demonstrated in medical conditions where diastolic dysfunction is expected. There is also ongoing research defining its utility in diagnosing and quantifying cardiac dyssynchrony. It may prove helpful in managing patients who are candidates for cardiac resynchronization therapy. Initial research has found the assessment of radial strain in determining the septal-to-posterior wall thickening delay to be a predictor of cardiac resynchronization therapy response.14 Speckle tracking combined with longitudinal TDI measurements predicts a better response to CRT than either parameter alone.15
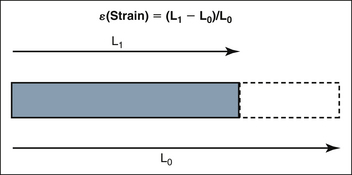
Figure 18-3 Strain: Deformation or change in length (L1 – L0) of an object normalized to its original length (L0)
1. Zagzebski J.A. Essentials of ultrasound physics. Doppler Implement. 1996;5:90-91.
2. Oh J., Seward J.B., Tajik A.J. The Echo Manual, 3rd ed. Philadelphia: Lippincott Williams & Wilkins; 2006.
3. Gulati V.K., Katz W.E., Follansbee W.P., Gorcsan J. Mitral annular descent velocity by tissue Doppler echocardiography as an index of global left ventricular function. Am J Cardiol. 1996;77:979-984.
4. Nagueh S.F., Middleton K.J., Kopelen H.A., et al. Doppler tissue imaging: a noninvasive technique for evaluation of left ventricular relaxation and estimation of filling pressures. J Am Coll Cardiol. 1997;30:1527-1533.
5. Ommen S.R., Nishimura R.A., Appleton C.P., et al. Clinical utility of Doppler echocardiography and tissue Doppler imaging in the estimation of left ventricular filling pressures: a comparative simultaneous Doppler-catheterization study. Circulation. 2000;102:1788-1794.
6. Ha J.W., Ommen S.R., Tajik A.J., et al. Differentiation of constrictive pericarditis from restrictive cardiomyopathy using mitral annular velocity by tissue Doppler echocardiography. Am J Cardiol. 2004;94:316-319.
7. Nagueh S.F., Bachinski L.L., Meyer D., et al. Tissue Doppler imaging consistently detects myocardial abnormalities in patients with hypertrophic cardiomyopathy and provides a novel means for an early diagnosis before and independently of hypertrophy. Circulation. 2001;104:128-130.
8. Armstrong G., Pasquet A., Fukamachi K., et al. Use of peak systolic strain as an index of regional left ventricular function: comparison with tissue Doppler velocity during dobutamine stress and myocardial ischemia. J Am Soc Echocardiogr. 2000;13:731-737.
9. Pislaru C., Belohalavek M., Bae R.Y., et al. Regional asynchrony during acute myocardial ischemia quantified by ultrasound strain rate imaging. J Am Coll Cardiol. 2001;37:1141-1148.
10. Abraham T.P., Belohlavek M., Thomson H.L., et al. Time to onset of regional relaxation: feasibility, variability, and utility of a novel index of regional myocardial function by strain rate imaging. J Am Coll Cardiol. 2002;39:1531-1537.
11. Hoffmann R., Altiok E., Nowak B., et al. Strain rate measurement by Doppler echocardiography allows improved assessment of myocardial viability in patients with depressed left ventricular function. J Am Coll Cardiol. 2002;39:443.
12. Serri K., Reant P., Lafitte M., et al. Global and regional myocardial function quantification by two-dimensional strain application in hypertrophic cardiomyopathy. J Am Coll Cardiol. 2006;47:1175-1181.
13. Perry R., De Pasquale C.G., Chew D.P., et al. Assessment of early diastolic left ventricular function by two-dimensional speckle tracking. Eur J Echocardiogr. 2008;9:791-795.
14. Cannesson M., Tanabe M., Suffoletto M.S., et al. Velocity vector imaging to quantify ventricular dyssynchrony and predict response to cardiac resynchronization therapy. Am J Cardiol. 2006;98:949-953.
15. Gorcsan J.III, Tanabe M., Bleeker G.B., et al. Combined longitudinal and radial dyssynchrony predicts ventricular response after resynchronization therapy. J Am Coll Cardiol. 2007;50:1476-1483.