Chapter 10
Specialized Test Regimens
1. Describe the indications for respiratory muscle strength testing.
2. Identify the normal range for exhaled nitric oxide values.
3. List two indications for preoperative pulmonary function testing.
4. Understand the difference between open- and closed-circuit calorimetry.
1. Judge the reliability of metabolic measurements.
2. Select appropriate tests to evaluate disability in either chronic obstructive pulmonary disease (COPD) or pulmonary fibrosis.
3. Understand the equation of motion and the impact of impedance and elastance on flow.
4. Evaluate the clinical implications of an elevated level of exhaled nitric oxide (eNO).
Respiratory muscle strength testing
Description
Technique
The patient is connected to a valve, shutter apparatus, or the PF testing system with a mouthpiece (a flanged mouthpiece may facilitate testing in subjects with excessive muscle weakness) and noseclip in place. The mouthpiece can be placed in the mouth or the lips pressed against the mouthpiece opening, as would be done with a bugle, thus the early term for the procedure “bugles” (Figure 10-1). Regardless of the mouth-device interfaced used, there should be a tight fit so that the patient can exert maximal pressure. The airway is occluded by blocking a port in the valve or by closing a shutter. In either system, a small, fixed leak is introduced between the occlusion and the patient’s mouth. The leak may be a small opening such as a large-bore needle or created by the PFT system in their hardware. The leak eliminates pressures generated by the cheek muscles during the MEP maneuver by allowing a small amount of gas to escape the oral cavity. Likewise, the leak prevents glottic closure during the MIP maneuver. The incorporation of this small leak does not significantly change lung volume or the pressure measurement.
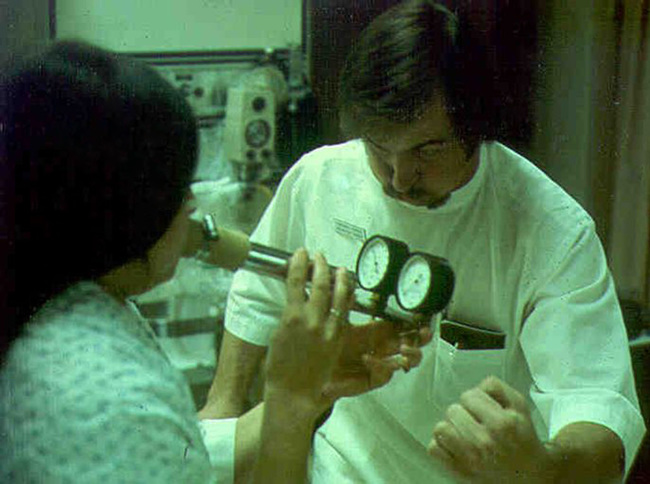
A simple and historical method of measuring maximal inspiratory and expiratory pressures involves the use of a small metal tube with a fixed leak and pressure gauges. During the expiratory maneuver, the subject would blow into the device with his or her lips within the mouthpiece, earning it the name, “bugle testing.”
Pressure may be measured using a manometer, an aneroid-type gauge, or a pressure transducer. The pressure-monitoring device should be linear over its range. It should be able to record pressures from −200 to approximately +200 cm H2O. Pressure transducers are typically used in PF testing systems with the output incorporated into a data acquisition screen and algorithms used to calculate the sustained pressure. If a manometer or aneroid gauge is used, the technologist directly observes the pressure and records it. This type of monitoring is common in the hospital where measuring vital capacity and respiratory pressures are used to assess ventilatory adequacy (Figure 10-2). The technologist should record the plateau pressure that the patient can maintain for 1–2 seconds.
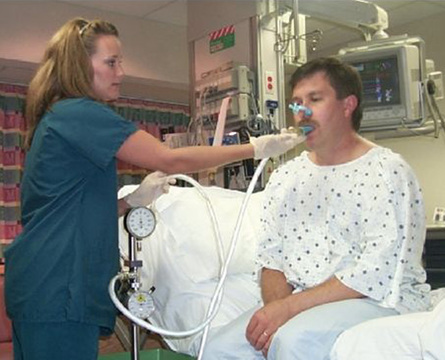
Measurement of vital capacity and respiratory muscle strength at the bedside.
For the MIP test, the patient is instructed to expire maximally. Monitoring expiratory flow or having the patient signal helps determine when maximal expiration has been achieved. Then the airway is occluded as described. The patient inspires maximally and maintains the inspiration for 1–2 seconds. The first portion of each maneuver is disregarded because it may include transient pressure changes that occur initially (Figure 10-3). The most negative value from at least three efforts that vary less than 20% is recorded.
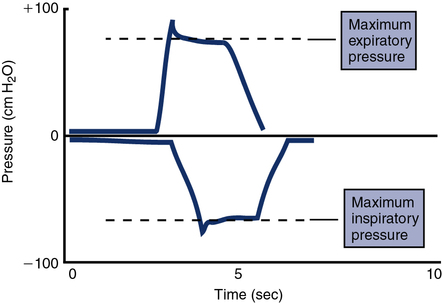
These tracings plot the respective pressures recorded in cm H2O against time on a single graph. Maximal inspiratory pressure shows a downward or negative deflection. Maximal expiratory pressure shows a positive or upward deflection. Each maneuver is conducted with the airway occluded (see text). The occlusion is maintained for a short interval (1 to 3 seconds), and any initial transient tracings are discarded.
MEP is recorded similarly. The patient inhales as much as possible, then exhales maximally against the occluded airway for 1–2 seconds. Longer efforts should be avoided as cardiac output can be reduced by the high thoracic pressures (e.g., Valsalva maneuvers) that are sometimes developed. MEP is usually larger than MIP in healthy patients. The pressure-monitoring device should be able to withstand the higher pressure without damage. The most positive value from at least three efforts that vary less than 20% is recorded. As for MIP, initial pressure transients during the MEP are disregarded. Both MIP and MEP require patient cooperation and effort. Low values may reflect a lack of understanding or insufficient effort. (Box 10-1)
Significance and Pathophysiology
Sniff nasal inspiratory pressure (SNIP) has been proposed as an alternative or complementary test to MIP. The measurement is performed by occluding a nostril during a maximal sniff maneuver performed through the contralateral nostril from functional residual capacity or residual volume. In the contralateral nostril, a nasal olive with a central catheter is connected to a pressure transducer. Instrumentation can be sophisticated with computer incentive screens to simple hand-held devices (Figure 10-4). Measurement limitations include nasal obstruction or collapse during the maneuver. Several papers have compared the test method to sniff pressures measured using an esophageal catheter with good correlation in subjects with neuromuscular disease. A sniff nasal-inspiratory force less than 40 cm H2O has been shown to predict mortality in patients with amyotrophic lateral sclerosis (sensitivity 97%, specificity 79%).
Exhaled nitric oxide
Description
Measurement of exhaled nitric oxide (eNO) provides a simple and noninvasive method for assessing airway inflammation. It is particularly useful in diagnosing and monitoring lung diseases characterized by eosinophilic inflammation, such as asthma. The fraction of eNO in an exhaled breath (FeNO) can be measured with a sensitive chemiluminescent or electrochemical analyzer (see Chapter 13). FeNO is usually reported in parts per billion (ppb). Because the measurement is dependent on flow during exhalation, the flow (in liters per second) may be subscripted to the term (e.g., FeNO0.05 represents the FeNO measured at a flow of 0.05 L/sec). NO is also sometimes measured from the nasal cavities, including the sinuses, as a marker of nasal inflammation.
Techniques
On-Line FeNO in Adults
Without breath holding, the patient then exhales slowly and evenly while exhaled gas is sampled continuously. To prevent contamination of the exhalate with nasal NO, the patient exhales against an expiratory resistance while receiving feedback to maintain a positive pressure at the mouth. This positive pressure (usually about +5 cm H2O) causes the velum in the posterior pharynx to close, preventing nasal NO from entering the air stream. The fractional concentration of NO in the exhaled gas varies inversely with the flow. To standardize on-line measurements, an exhaled flow of 0.05 L/sec (i.e., 50 mL/sec) ±10% is recommended. This flow allows dead space gas to be exhaled and a plateau in NO to be observed in about 10 seconds in adults (Figure 10-5). The correct flow can be maintained by using a pressure-sensitive flow controller and providing visual feedback to the subject. Current commercially available instrumentation (Chapter 11 uses visual incentives to assure standardized flow/pressure and will mark a maneuver as not meeting acceptability criteria if flow/pressure is not maintained).
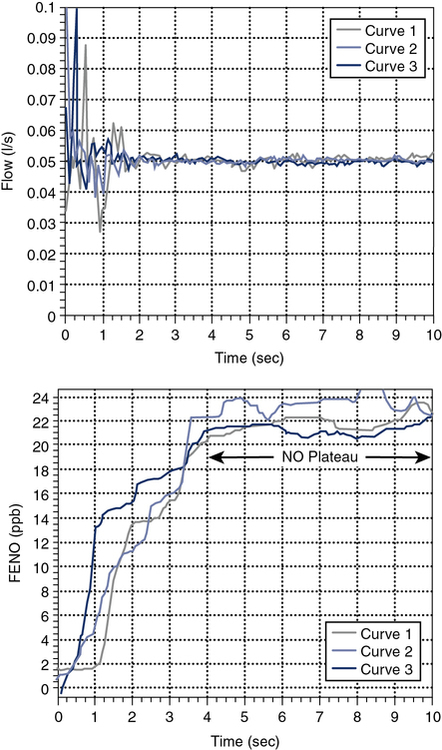
The upper graph shows three efforts in which flows are measured at the mouth. The subject exhales against a slight resistance to close the velum in the oropharynx. Visual feedback in the form of a computer display helps the patient maintain a constant pressure and thereby a constant flow at the recommended 0.05 L/sec. The lower graph displays the FeNO values for the three maneuvers over 10 seconds of exhalation. Exhaled NO rises to a plateau and the FeNO concentration is measured over a 3-second (i.e., 0.15 L) window from the plateau (see text).
FeNO is measured from the plateau of the single-breath exhalation profile (see Figure 10-5). The plateau phase may slope up or down slightly. The exhalation should last long enough to establish this plateau (>6 seconds for adults and children older than 12 years, >4 seconds for children ages 12 years and younger). Two points should be chosen on the plateau that represent a 3-second interval (about 0.15 L) in which the FeNO varies less than 10%. The FeNO is the mean concentration over this 3-second interval. For FeNO values of 10 ppb or less, variability of 1 ppb NO may be used in place of the 10% criteria. A minimum of 30 seconds should elapse between repeated measurements with the subject breathing air (off the NO sampling circuit).
Off-Line FeNO in Adults
The patient inhales through an NO scrubber or from a reservoir with NO-free gas. After inhaling to TLC, the patient exhales his or her VC into an appropriate sampling device without breath holding. Expiratory resistance (+5 cm H2O) is added just as is done for on-line measurements to minimize contamination by nasal NO. Flow is usually controlled by monitoring the back-pressure in the system; flows of 0.35 L/sec ±10% are recommended to allow the VC to be collected in a reasonable interval. The sample is collected in a balloon made of polyester (Mylar) or a similar material that is impermeable to NO and large enough to accommodate the adult’s VC. Off-line samples should be analyzed within 12 hours. Exhaled nitric oxide can be performed in children able to perform the single breath maneuver and is described in Chapter 8.
Nasal Nitric Oxide
Although various methods of sampling nasal NO have been described, the recommended method uses transnasal airflow in series. In this technique, air is aspirated into one naris and out the opposite side, where NO is sampled. The subject exhales against a resistance of approximately 10 cm H2O, while air is aspirated at a constant flow rate. As for measurement of FeNO from the lower airways, exhalation against resistance closes the velum in the posterior pharynx to prevent contamination of the sample. Airflow of 0.25–3.0 L/min is used for nasal NO measurements. Flows in this range allow a plateau in the NO signal within 20–30 seconds in most adults. The flow used for NO analysis, along with the transnasal flow, should be recorded (Figure 10-6).
Significance and Pathophysiology
Nasal NO levels are typically much higher than eNO. Values from 100 ppb up to more than 1000 ppb have been reported. Most of the nasal NO appears to be produced by the epithelial cells in the paranasal sinuses. Patients with allergic rhinitis show elevated levels of NO that appear to be responsive to nasal corticosteroids. One disease in which nasal NO measurements may be particularly useful is primary ciliary dyskinesia (PCD). Patients who have PCD have much lower levels of nasal NO (Interpretive Strategies Box 10-1).
Forced oscillation technique
Impedance of the respiratory system (Zrs) represents the net force that must be overcome to move gas in and out of the respiratory system (upper airway, lungs, and chest wall). Applying oscillatory flow is appropriate because that is how we breathe, in a regular in-and-out fashion. Imagine the lungs modeled as a stiff pipe (the airways) with a balloon on the end (the alveoli). The pressure required to push gas down the pipe and into the balloon must overcome three basic forces: the resistance (R) of the pipe, the elastance (E), or stiffness, of the balloon, and the inertia (I) of the gas itself (Figure 10-7). Stated mathematically, the pressure necessary to move gas down the pipe and into the balloon is as follows:
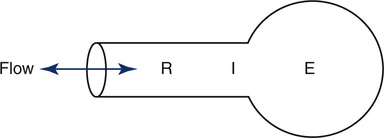
Measuring the pressure, flow, and volume generated at the mouth during breathing, one can solve for the parameters resistance (R), elastance (E), and inertia (I) that define the mechanical characteristics of the model.
< ?xml:namespace prefix = "mml" />

To measure Z, one could apply flow at various single frequencies and measure the resulting pressures generated at each frequency. Alternatively, one could apply a flow signal consisting of many different frequencies at once and then use a mathematical function known as the fast Fourier transform (FFT) to break down the output into unique sine waves, each of its own frequency. Despite involving complex mathematics with real and imaginary numbers, computers have made this method quick and accurate, and it has become the technique most commonly used (Figure 10-8, A).
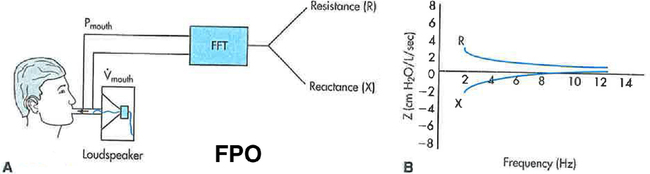
A, A typical apparatus for measuring input Zrs involves a loudspeaker generating a flow signal consisting of many, simultaneous frequencies. These pulsations are delivered to the mouth while mouth pressure (P) and flow (VY) are measured. These measurements are then processed using the fast Fourier transform (FFT). B, The real (R) and imaginary (X) parts of Zrs are shown in relation to frequency. R is frequency dependent, falling to lower values with higher frequency.
Traditional devices use loudspeakers pulsating at different, predetermined frequencies to generate the broadband flow signals (Figure 10-9, A). One commercially available device (Figure 10-9, B) generates the broadband flow signal by an electronically controlled deflection of an internal speaker to create an impulse of flow containing many frequencies, although the frequencies analyzed range from 5–35 Hz. For all devices, the flow signal can be applied during quiet spontaneous breathing and thus requires little subject effort or cooperation. Once performed, the output is recorded in two parts: the part of Z that is in phase with the flow signal, which represents the real part of the Z and is due to flow resistive properties of the respiratory system, R, and the part that is out of phase with the flow signal, called the reactance (X), which represents the imaginary part of Z, and encompasses E and I. These real and imaginary parts are plotted against frequency, as shown in Figure 10-8, B.
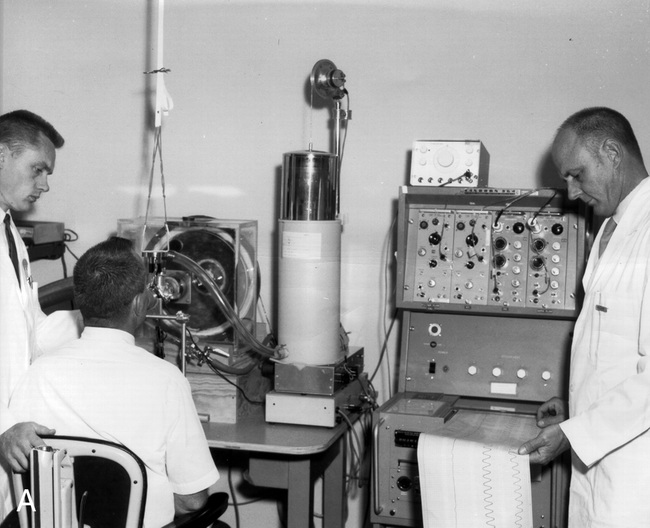
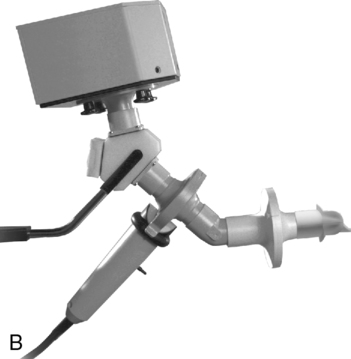
Mayo Clinic pulmonary physiology lab in the 1960s testing FOT using a loudspeaker and recorder. B, A built-in loud speaker generates an impulse of flow containing a wide range of frequencies (5–35 Hz). (Courtesy Carefusion, Cardiopulmonary Diagnostics, Yorba Linda, Calif.)
In most clinical applications, the FOT is used to measure R. Because the technique is noninvasive, fast, and easy, it can be applied in many situations where other methods of measuring R are difficult or cumbersome. These situations include use in pediatric patients or others who cannot perform the panting maneuvers to measure Raw by the body box technique. However, as with Raw measured in the body box, the maneuver does require some degree of subject cooperation, including cheek holding to reduce the effect of facial noise in the signal (Figure 10-10). The R derived from the FOT has been shown to be sensitive to bronchoconstriction and bronchodilatation and thus can be used to assess airway hyperresponsiveness. An important disadvantage of the technique is that, unless an esophageal balloon is placed to measure transpulmonary pressure, the R measured is not of the lungs alone but of the entire respiratory system, thus including the upper airway and chest wall. The upper airway, in particular, can markedly influence the measurement because of its high compliance and the propensity for glottic interference.
Preoperative pulmonary function testing
1. To estimate postoperative lung function in candidates for pneumonectomy or lobectomy
2. To plan perioperative care (preoperative preparation, type and duration of anesthetic during surgery, postoperative care) to minimize complications
3. To enhance the estimate of risk involved in the surgical procedure (i.e., morbidity and mortality) derived from history and physical examination
Preoperative pulmonary function testing may be indicated in patients who have the following:
2. Symptoms of pulmonary disease (e.g., cough, sputum production, shortness of breath)
3. Abnormal physical examination findings, particularly of the chest (e.g., abnormal breath sounds, ventilatory pattern, respiratory rate)
Pulmonary Artery Occlusion Pressure
Tests that predict the effects of resection on the remaining lung are normally done in series, with spirometry done first, followed by split-function lung scans (if spirometry results are acceptable), and then pulmonary artery occlusion pressure (if cor pulmonale is a concern). Table 10-1 summarizes general value ranges used for preoperative pulmonary function testing.
Table 10-1
Preoperative Pulmonary Function
Test | Increased Postoperative Risk | High Postoperative Risk | Candidate for Pneumonectomy* |
FVC | < 50% of predicted | < 1.5 L | |
FEV1 | < 2.0 L or 50% of predicted | < 1.0 L | > 2.0 L |
MVV | < 50 L/min or 50% of predicted | > 50 L/min or 50% of predicted | |
Paco2 | > 45 mm Hg | ||
![]() |
15–20 mL/min/kg | < 15 L/min/kg | |
Predicted postoperative FEV1 | > 0.8 L/min | ||
Pulmonary artery occlusion | > 35 mm Hg |
*Values in this column determine whether the patient is to be considered a candidate for lung resection (see text).
Pulmonary function testing for disability
Forced Vital Capacity and Forced Expiratory Volume
Spirometry is the most useful index for the assessment of impairment caused by airway obstruction. The test should not be performed unless the patient is stable. The reported FVC and FEV1 should be the largest values obtained from at least three acceptable maneuvers. The two largest FVC values and FEV1 values should be repeatable within 5% or 0.1 L, whichever is greater. Peak flow should be achieved early in the expiration, and the spirogram should show gradually decreasing flow throughout the breath. Spirometry should be repeated after inhaled bronchodilator if the patient’s FEV1 is less than 70% of the predicted value. Spirometric efforts, before and after bronchodilators, should meet these repeatability criteria. Standing height, without shoes, should be used for comparison of measured values with limits for disability (Table 10-2). In case of marked spinal deformity, arm-span measurement should be used (see Chapter 1).
Table 10-2
Forced Expiratory Volume and Forced Vital Capacity Values for Disability Determinations
Height Without Shoes (in.) | FEV1 Equal to or Less Than (L) | FVC Equal to or Less Than (L) |
60 or less | 1.05 | 1.25 |
61–63 | 1.15 | 1.35 |
64–65 | 1.25 | 1.45 |
66–67 | 1.35 | 1.55 |
68–69 | 1.45 | 1.65 |
70–71 | 1.55 | 1.75 |
72 to more | 1.65 | 1.85 |
Adapted from Disability Evaluation Under Social Security (Blue Book, September 2008) 3.00 Respiratory System—Adult.
Computation of the FEV1 should be done with back-extrapolated volumes (see Chapter 2). The spirogram is acceptable if the back-extrapolated volume is less than 5% of the FVC, or 0.1 L, whichever is greater. Each maneuver should be continued for 6 seconds or until there is no detectable change in volume for the last 2 seconds of the maneuver. It is unacceptable to report FEV1 when only an F-V curve is recorded. A volume-time tracing from which FEV1 can be measured is required. All lung volumes and flows must be reported at body temperature, pressure, and saturation (BTPS).
Volume calibration of the spirometer should agree to within 1% of a 3-L syringe. If spirometer accuracy is less than 99% but within 3% of the calibration syringe, a calibration correction factor should be used (see Chapter 12). If a flow-sensing spirometer is used, linearity should be documented by performing calibration at three different flows (3 L/6 sec, 3 L/3 sec, and 3 L/1 sec). The volume-time tracing should have the time sensitivity marked on the horizontal axis and the volume sensitivity marked on the vertical axis. The paper speed should be at least 20 mm/sec and the volume excursion at least 10 mm/L to allow manual calculation of the FEV1 and FVC. The manufacturer and model of the spirometer should be stated in the report (see Criteria for Acceptability Box 10-2).
Diffusing Capacity
The Dlco is useful in determining impairment because of chronic impairment of gas exchange in both obstructive and restrictive disorders. The single-breath method should be used. The standard criteria for acceptability and repeatability for the Dlco maneuver may be applied (see Chapter 3). However, the IVC should be at least 90% of the patient’s best VC and the breath-hold time between 9 and 11 seconds. The reported value should be uncorrected for hemoglobin (Hb), but abnormal Hb or carboxyhemoglobin (COHb) values should be reported. Correction for altitude should be made if the PIO2 is significantly different from 150 mm Hg. If the Dlco is greater than 40% of predicted but less than 60%, resting blood gas analysis is indicated.
Arterial Blood Gases
Although blood gas results are objective, they are largely nonspecific in determining impairment. Blood gases should be obtained while the subject is breathing air, either sitting or standing. The A-aO2 gradient may not be reliable because it can be affected by hyperventilation (Table 10-3). Blood gas analysis may be required in diffuse pulmonary fibrosis and should include Pao2 and Paco2. Blood gases (and A-a gradient) may also be assessed during exercise. The requirement for supplemental O2 may also be quantified by exercise blood gas analysis. Pulse oximetry or capillary blood gas analysis is not an acceptable substitute for arterial blood gas analysis. Blood gas analysis should be performed by a laboratory certified by a state or federal agency.
Table 10-3
Arterial Oxygen Tension for Disability Determinations
Less Than 3000 Feet Above Sea Level | 3000 to 6000 Feet Above Sea Level | More Than 6000 Feet Above Sea Level | |
PCO2 (mm Hg) | PO2 (mm Hg) | PO2 (mm Hg) | PO2 (mm Hg) |
30 or below | 65 | 60 | 55 |
31 | 64 | 59 | 54 |
32 | 63 | 58 | 53 |
33 | 62 | 57 | 52 |
34 | 61 | 56 | 51 |
35 | 60 | 55 | 50 |
36 | 59 | 54 | 49 |
37 | 58 | 53 | 48 |
38 | 57 | 52 | 47 |
39 | 56 | 51 | 46 |
40 or above | 55 | 50 | 45 |
Adapted from Disability Evaluation Under Social Security (Blue Book, September 2008) 3.00 Respiratory System—Adult.
Exercise Testing
Patients considered for exercise evaluation should first have resting blood gas evaluation, either sitting or standing. A steady-state exercise test (see Chapter 7) is then performed, preferably with a treadmill. The patient should exercise for 4–6 minutes at an O2 consumption rate (o2) of approximately 17.5 mL/min/kg (approximately 5 METs) breathing room air. An equivalent workload should be used for cycle ergometry (e.g., 75 W for a 175-lb patient). Blood gas samples should be drawn at this workload to determine whether significant hypoxemia is present (see Table 10-3). If the patient does not desaturate at this level, a higher workload can be used to determine exercise capacity. If the patient cannot achieve a workload of 5 METs, a lower workload can be selected to determine exercise capacity.
Limits for determining disability based on respiratory impairment have been set for the United States by the Social Security Administration. Criteria are set according to the disease category (see Interpretive Strategies 10-1). COPD is evaluated by comparing FEV1 with the values in Table 10-2. Restrictive ventilatory disorders are evaluated by comparing FVC with the values in Table 10-2. Impaired gas exchange is evaluated by comparing Pao2 with the values in Table 10-3. Disability caused by asthma is also evaluated with FEV1. Episodes of asthma (requiring emergency treatment or hospitalization) occurring at least every 2 months or at least six times per year may also be evidence of disability (Criteria for Acceptability 10-3 and Interpretive Strategies 10-2).
Metabolic measurements: indirect calorimetry
Description
Techniques
Open-Circuit Calorimetry
Exchange of O2 and CO2 may be measured by recording the fractional differences of O2 and CO2 between inspired and expired gas. These measurements are accomplished with a mixing chamber, a dilution system, or a breath-by-breath system similar to those used for expired gas analysis during exercise (see Chapter 7). o2 and
co2 are measured as described for exercise testing with mixing chamber and breath-by-breath systems.
E, VT, and fb (respiratory rate) may be measured simultaneously. In systems that use the dilution principle, a constant flow of gas is mixed with expired air. The dilution of CO2 is then used to calculate ventilation. Connection to the patient may be made by a standard directional breathing valve with a mouthpiece and noseclips. A ventilated hood or canopy (Figure 10-11) may also be used. Almost all metabolic measurement systems provide for connection to a mechanical ventilator circuit.
Performing Metabolic Measurements
The primary purpose of indirect calorimetry is to estimate REE over an extended period, usually 24 hours. To extrapolate the values obtained during the sampling period, the patient’s condition during the measurement is critical (Criteria for Acceptability 10-4). The calorimeter or metabolic cart should be calibrated at least daily, preferably before each test. Gas analyzers should be calibrated using gas concentrations appropriate for the clinical situation. Sample lines and gas-conditioning devices (absorbers)
should be checked before each test. If calibration or testing produces questionable values, the device should be checked against a known standard. Burning ethanol or other material with a fixed RQ can be used. A large-volume syringe can be used to simulate a patient with o2 and
co2 values near zero. Alternately, biologic controls can be used to check the precision of the calorimeter.
Pre-test instruction should include fasting after midnight in the outpatient subject or for 2–4 hours before the test starts in a hospitalized patient. If the inpatient is receiving either enteral or parenteral feedings, the feedings should be continuous rather than in bolus form. Information about the type and amount of nutritional support in the previous 24 hours may be helpful in interpreting test results. Drugs or substances that alter metabolism should be avoided. Substances such as caffeine and nicotine are particularly common stimulants. Theophylline-based drugs may also increase metabolic rate. The subject should refrain from exercise. Typically these tests are performed in the morning hours to try to reduce the amount of activity performed before testing. The patient should be recumbent or supine for 20–30 minutes before beginning measurements and should stay quiet during the test. Ideally, the patient should be awake and alert during testing. The testing apparatus should not cause discomfort or exertion for the patient, which is the reason canopy testing is ideal for this procedure because breathing valves, mouthpieces, and noseclips may alter the patient’s breathing pattern. The patient should be in a neutral thermal environment. Special corrections may be required for patients who are febrile or hypothermic. The patient’s temperature at the time of the test should be recorded along with a temperature history of the previous 24 hours. Temperature changes of 1 °C can result in a 13% change in REE. Data collection should continue long enough to establish a stable baseline and verify steady-state conditions (Figure 10-12). Ten to 15 minutes of stable readings for o2 and
co2 may be required, but an adequate measurement can be obtained in as short an interval as 5 minutes. Common indicators of steady-state conditions are the parameters assessed as part of the metabolic study.
o2 and
co2 should not vary more than 5% from the mean value measured during the te st (at least 5 minutes). Respiratory quotient (RQ) values should be within the normal physiologic range (0.67–1.30). If the patient does not achieve steady-state conditions, a longer test interval may be required to average representative periods of metabolic activity. Patients on ventilators should be in a stable condition. Leaks in the ventilator circuit, around cuffed endotracheal tubes, or from chest tubes or bronchopleural fistulas may invalidate measurements of RQ and REE. No ventilator adjustments should be made 1–2 hours before the test period. Modifications in minute ventilation or FIO2 settings can cause gross changes in the patterns of gas exchange, particularly in patients with pulmonary disease. The ventilator must have a stable delivered O2 concentration; FIO2 settings greater than 0.60 may result in erroneous
o2 measurements. Appropriate valves may need to be used for ventilator modes that involve continuous gas flow.
Metabolic Calculations
The Harris-Benedict equations are commonly used to estimate REE:


The Weir equation is used to calculate REE from respiratory gas exchange and urinary nitrogen:

o2 is expressed in mL/min (STPD)
co2 is expressed in mL/min (STPD)
UN = urinary nitrogen (g/24 hr)
If UN is unknown, REE may be calculated

UN= urinary nitrogen (g/24 hr)
1.44= factor to convert mL/min to L/24 hr
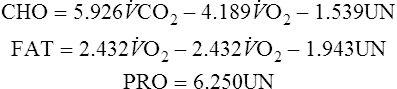
CHO= Carbohydrates oxidized in grams/24 hours
FAT= Fat oxidized in grams/24 hours
PRO= Protein oxidized in grams/24 hours
From the grams of each substrate used, the kcal derived from that source can be computed:
Carbohydrates (in kcal) = 4.18 carbohydrates (in grams)
Fat (in kcal) = 9.46 fat (in grams)
Protein (in kcal) = 4.32 protein (in grams)
Significance and Pathophysiology
See Interpretive Strategies 10-3. Indirect calorimetry assesses nutritional status in patients whose daily energy needs are altered by disease, injury, or therapeutic interventions. REE accounts for approximately two thirds of the daily energy requirements in healthy patients. The Harris-Benedict equations, or similar predictive equations, are commonly used to estimate REE. Various factors can be used to adjust estimated REE to account for additional caloric needs imposed by the patient’s clinical status. This approach works well in many patients. However, metabolic requirements of critically ill patients vary widely. Indirect calorimetry is indicated for patients who do not respond favorably to traditional methods of nutritional assessment and support. Indirect calorimetry can be used to detect undernourishment, overnourishment, or use of inappropriate substrates (Box 10-2).
Technical considerations involved in indirect calorimetry include the accuracy of gas analysis and measurement of expired volume during the test (open-circuit methods). The most common problem during metabolic measurements is attainment of a true steady state. Only if the measurements are made under steady-state conditions is the metabolic rate representative of caloric expenditure over 24 hours. Hyperventilation resulting from connection to a mask or mouthpiece, or from ventilator manipulation, occurs frequently. Head hoods or continuous-flow canopies can eliminate much of the stimulation associated with connection to the metabolic measurement system (see Figure 10-10, A) but cannot be used for patients on mechanical ventilators. Hoods or canopies may also cause hyperventilation in awake, alert patients. An RER greater than 1.0 should always be evaluated in relation to E and end-tidal CO2. Abnormally high
E and low end-tidal CO2 values may indicate hyperventilation. RER values in excess of 1 that cannot be explained as hyperventilation may be caused by storage of excess calories as fat (lipogenesis). RER values between 0.67 and 0.70 may occur in ketosis caused by extreme fasting or diabetic ketoacidosis. However, more commonly, low RER values (<0.67) signal improper calibration of the CO2 or O2 analyzers. Inaccurate calibration or improper performance of gas analyzers can result in RER values outside of the usual metabolic range of 0.70–1.

If a stable FIO2 cannot be achieved (as is often the case when it is 0.60), REE can be estimated from the
co2. If an RQ of 0.85 is assumed,
o2 can be estimated by dividing the
co2 by the RQ and then solving the Weir equation (see pg 342). This method will underestimate the REE when the RQ is greater than 0.85, with a maximal error of about 25% if the RQ is really 1.20. Similarly, the REE will be overestimated for RQ values less than 0.85, with a maximal error of approximately 19% if the RQ is 0.67.
Other considerations involved in metabolic measurements of ventilated patients include the effects of positive pressure on gas analysis and on volume determination. Analysis of O2 and CO2 in the ventilator circuit must take into account the effect of positive pressure breaths on the gas analyzers. Depending on the sampling method used, positive pressure swings during each breath may generate falsely high partial pressure readings. Closed-circuit calorimetry places a volumetric device in the breathing circuit between the ventilator and the patient (Figure 10-10, B). The volume delivered by the ventilator must be increased to accommodate the higher compressible gas volume in the circuit, approximately 1 mL/cm H2O for each liter of added volume.
Summary
• Respiratory muscle strength (RMS) can be a helpful tool in characterizing neuromuscular disease and other abnormalities that affect the diaphragm and abdominal muscles.
• Exhaled nitric oxide (FeNO) provides a noninvasive means of measuring eosinophilic inflammation of the airways. FeNO may be useful in the diagnosis and management of diseases characterized by inflammation such as asthma.
• Impulse oscillometry uses high frequency oscillations to measure the mechanical properties of the respiratory system, in particular, resistance and impedance. It is especially useful in children or subjects who cannot perform conventional pulmonary function tests such as spirometry.
• Preoperative testing and disability testing use spirometry, lung volumes, diffusing capacity, blood gases, and exercise testing. Each test examines a specific aspect of either preoperative risk or respiratory impairment that prevents work.
• Metabolic measurements, specifically indirect calorimetry, provide a means of assessing nutritional status and support. They may be particularly useful in the evaluation of patients who do not respond adequately to estimated nutritional needs.