Chapter 12 Special Senses
The special senses of olfaction, vision, taste, hearing and balance are conveyed to the brain in cranial nerves. In each case, highly specialized peripheral receptors respond to stimuli in the external environment or our relationship to it. The olfactory system has an ancient lineage, reflected by the fact that afferent olfactory pathways proceed directly to the cerebral cortex and bypass the thalamus. Its terminal fields are, likewise, primitive cortical areas in a phylogenetic sense and are considered to be parts of the limbic system. All other special senses have a thalamic representation that projects to specialized regions of the neocortex. The integrative functions related to the various special senses (e.g. control of ocular gaze) are also included here. Of particular importance is a detailed discussion of retinal functional anatomy.
Olfaction
Olfactory pathways subserving the sense of smell are described in this section. Details of the relationship between the olfactory pathways and the limbic system are shown in Figure 16.7.
There is a clear laminar structure in the olfactory bulb (Fig. 12.1). From the surface inward, the laminae are the olfactory nerve layer, glomerular layer, external plexiform layer, mitral cell layer, internal plexiform layer and granule cell layer.
The olfactory tract leaves the posterior pole of the olfactory bulb to run along the olfactory sulcus on the orbital surface of the frontal lobe (see Fig. 16.7). The granule cell layer of the bulb is extended into the olfactory tract as scattered medium-sized multipolar neurones that constitute the anterior olfactory nucleus. They continue into the olfactory striae and trigone to the grey matter of the prepiriform cortex, anterior perforated substance and precommissural septal areas. Many centripetal axons from mitral and tufted cells relay in, or give collaterals to, the anterior olfactory nucleus; the axons from the nucleus continue with the remaining direct fibres from the bulb into the olfactory striae.
As the olfactory tract approaches the anterior perforated substance, it flattens and splays as the olfactory trigone. Fibres of the tract continue from the caudal angles of the trigone as diverging medial and lateral olfactory striae, which border the anterior perforated substance. An intermediate stria sometimes passes from the centre of the trigone to end in a small olfactory tubercle. The lateral olfactory stria follows the anterolateral margin of the anterior perforated substance to the limen insulae, where it bends posteromedially to merge with an elevated region, the gyrus semilunaris, at the rostral margin of the uncus in the temporal lobe (see Fig. 16.7). The lateral olfactory gyrus forms a tenuous grey layer covering the lateral olfactory stria; it merges laterally with the gyrus ambiens, part of the limen insulae. The lateral olfactory gyrus and gyrus ambiens form the prepiriform region of the cortex, passing caudally into the entorhinal area of the parahippocampal gyrus. The prepiriform and periamygdaloid regions and the entorhinal area (area 28) together make up the piriform cortex. The medial olfactory stria, covered thinly by the grey matter of the medial olfactory gyrus, passes medially along the rostral boundary of the anterior perforated substance toward the medial continuation of the diagonal band of Broca. Together, they curve up on the medial aspect of the hemisphere, anterior to the attachment of the lamina terminalis. The diagonal band enters the paraterminal gyrus. The medial stria becomes indistinct as it approaches the boundary zone, which includes the paraterminal gyrus, parolfactory gyrus and, between them, prehippocampal rudiment (see Fig. 16.7).
Vision
Eye
The eyeball, the peripheral organ of vision, is situated in the orbit, a skeletal cavity whose walls help protect the eye from injury (Fig. 12.2). The orbit also has a more fundamental role in the visual process itself: it provides rigid support and direction to the eye and forms the sites of attachment for its external muscles. This setting permits the accurate positioning of the visual axis under neuromuscular control and determines the spatial relationship between the two eyes—essential for binocular vision and conjugate eye movements.
Visual Pathway
The visual pathway is illustrated in Figure 12.3. The first-order neurone of the visual system is a bipolar cell that is contained entirely within the retina. The second-order neurone is a ganglion cell whose axon enters the optic nerve.
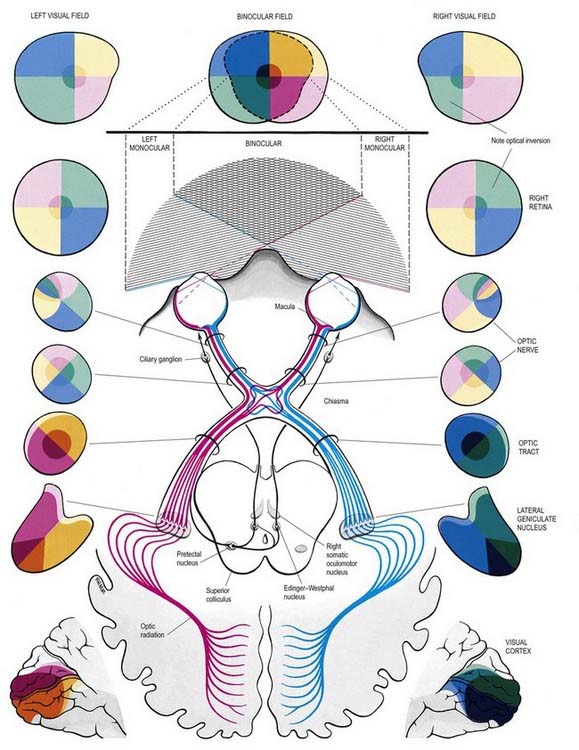
Fig. 12.3 The visual pathway, showing the spatial arrangement of neurones and their fibres in relation to the quadrants of the retinae and visual fields. The proportions at various levels are not exactly to scale; in particular, the macula is exaggerated in size in the visual fields and retinae. In each quadrant of the visual field and the parts of the visual pathway subserving it, two shades of the respective colour are used—paler for the peripheral fields, and darker for the macular part of the quadrant. From the optic tract onward, these two shades are both more saturated to denote the intermixture of neurones from both retinae, with the palest shade reserved for parts of the visual pathway concerned with monocular vision. The pathway subserving the pupillary light reflex is also indicated.
Axons from third-order visual neurones in the lateral geniculate nucleus run in the retrolenticular part of the internal capsule and form the optic radiation, which curves dorsomedially to the occipital cortex. Fibres representing the lower half of the visual field sweep superiorly to reach the visual cortex above the calcarine sulcus. Those representing the upper half of the visual field curve inferiorly into the temporal lobe (Meyer’s loop) before reaching the visual cortex below the calcarine sulcus.
Retina
The retina is the sensory neural layer of the eyeball (Figs 12.4–12.6). It is a very complex structure and should be considered a special area of the brain, from which it is derived by outgrowth from the diencephalon (Ch. 15). It is dedicated to the detection and early analysis of visual information and is an integrated part of the much larger apparatus of visual analysis present in the thalamus, cortex and other areas of the central nervous system.
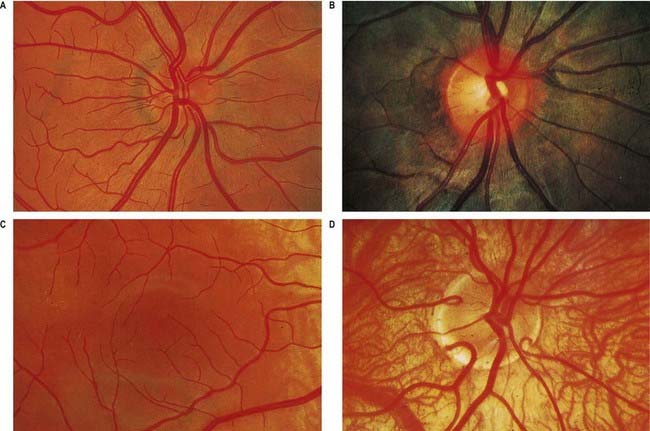
Fig. 12.4 Ophthalmoscopic photographs of the right human retina. A, Note the dichotomous branching of vessels. Arteries are brighter red and show a more pronounced ‘reflex’ to light, as a pale stria along their length. The veins are also larger in calibre; more of them cross arteries superficially than is usual. The optic disc, around the entry of the vessels, is a light pink, with a surrounding zone of heavier pigmentation. Compare with Figure 12.5A from the same Caucasian adult. B, Appearances in a heavily pigmented individual (an adult of African origin), with a paler optic disc than in A. Note accentuation of the edge of the disc by retinal and choroidal pigmentation. The arteries cross the veins superficially in this retina. C, Normal macula of a young Caucasian subject. The vessels radiate from the centrally placed fovea. The macular branches of the central retinal artery are approaching from the right. The macula is largely free of vessels of macroscopic size, but the capillaries here form a particularly close network, except at the fovea. D, The region of the optic disc in an eye with poorly developed pigmentation. Three cilioretinal arteries are curving around the edge of the disc (two on the left, one on the right). Between the two cilioretinal arteries, a single macular artery is apparent. Due to the depressed pigmentation, choroidal vessels are also visible, especially veins; on the left of the photograph, two large vorticose venous tributaries can be seen.
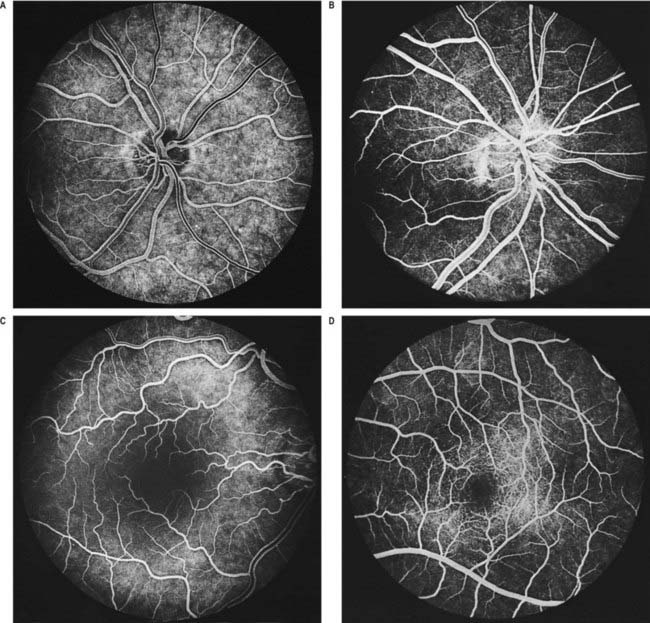
Fig. 12.5 Fluorescence angiograms of the retina. These are produced by photography with a fundus camera at known periods of time following the introduction of fluorescein into the circulation. A, Angiogram of the same retina shown in Figure 12.4A, taken in ‘mid-venous’ phase. The arteries display an even fluorescence, but the veins appear striped, owing to laminar flow. This appearance is the reverse of the arterial ‘reflex’ shown in Figure 12.4A and should not be compared with it. The background mottling is due to fluorescence from the choroidal vessels. B, Angiogram of the left optic disc, showing the major arteries and veins and also their smaller branches. Note particularly the radial pattern in the retinal capillaries. The laminar flow in the veins is less obvious than in Figure 12.4A. C, Angiogram showing the macular region of a right eye. The main macular vessels are approaching from the right. The subject is an elderly person with considerable macular pigmentation, which masks fluorescence from the choroidal circulation. D, Angiogram of the macula of a young subject (left eye) showing the macular capillaries in detail. Note the central avascular fovea. Compare with C.
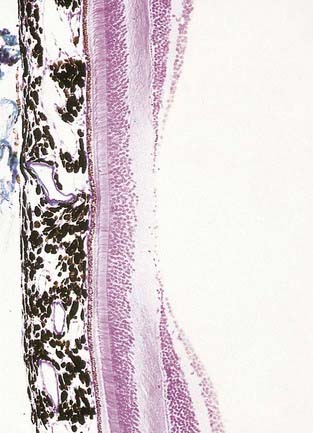
Fig. 12.6 Section through the fovea centralis.
(By permission from Young, B., Heath, J.W., 2000. Wheater’s Functional Histology, Churchill Livingstone, Edinburgh.)
Near the centre of the retina, there is a region 5 to 6 mm in diameter that contains the macula lutea (see Fig. 12.5C, D), an elliptical yellowish area measuring approximately 2 mm horizontally and 1 mm vertically. Its colour is due to the presence of xanthophyll derivatives. The macula lutea contains a central depression, the fovea centralis or foveola, with a diameter of approximately 0.4 mm, where visual resolution is highest (see Fig. 12.6.) Here, all elements except pigment epithelium cone photoreceptors are displaced laterally. The minute size of the foveola is the reason why the visual axes must be directed with great accuracy to achieve the most discriminative vision.
About 3 mm medial (nasal) and 1 mm superior to the foveola, the optic nerve becomes continuous with the retina at the optic disc (‘blind spot’). It is approximately 1.5 mm in diameter. The name ‘optic papilla,’ which is often applied to the disc, is a misnomer because almost all of a normal disc is level with the retina. Centrally, it contains a shallow depression, where it is pierced by the central retinal vessels (see Figs 12.2, 12.4, 12.5A, B). The disc is devoid of photoreceptors and is therefore insensitive to light. By ophthalmoscopy, the disc is normally pink, but it is much paler than the retina and may be grey or almost white. In optic atrophy the capillary vessels disappear, and the disc is then white.
Layers of the Retina
The retina is organized into layers or zones (Fig. 12.7), where distinctive components of its cells are clustered together or in register to form continuous strata. These layers extend uninterrupted throughout the photoreceptive retina except at the exit point of the optic nerve fibres at the optic disc, although certain layers are much reduced at the foveola where the photoreceptive elements predominate. The names given to the different layers reflect, in part, the components present within them, and also their position in the thickness of the retina. Conventionally, those structures farthest from the vitreous (i.e. toward the choroid) are designated as outer or external, and those toward the vitreous are inner or internal.
Customarily, 10 retinal layers are distinguished (Fig. 12.8), beginning at the choroidal edge and passing toward the vitreous. These are the retinal pigment epithelium, layer of rods and cones (outer and inner segments), external limiting membrane, outer nuclear layer, outer plexiform layer, inner nuclear layer, inner plexiform layer, ganglion cell layer, nerve fibre layer, and internal limiting membrane. Some of these are subdivided into substrata, and an innermost plexiform layer between layers 8 and 9 has also been demonstrated.
Rod and cone cells reach radially inward from the rod and cone lamina through the outer nuclear layer, where they have their nuclei, to the outer plexiform layer, where they synapse with bipolar and horizontal cells. Bipolar cells possess dendrites in the outer plexiform layer, cell bodies and nuclei in the inner nuclear layer, and axons in the inner plexiform layer, where they synapse with ganglion cell and amacrine cell dendrites. Horizontal cells have their dendrites and axons in the outer plexiform layer and their nuclei in the inner nuclear layer; ganglion cells have their dendrites in the inner plexiform layer, their cell bodies in the ganglion cell layer and their axons in the layer of nerve fibres (and within the optic nerve). Amacrine cell dendrites are mainly in the inner plexiform layer, although some (interplexiform cells) extend into the outer plexiform layer; amacrine cell dendrites are situated in either the inner nuclear layer or the outer part of the ganglionic layer (displaced amacrines). Pigment cells lie behind the retina, and several types of retinal glial cell are distributed in distinctive locations among its different layers.
The composition of the retinal layers is as follows:
Layer 7: Inner Plexiform Layer
This is divisible into three layers, depending on the types of contact that occur. The outer or ‘off’ layer contains synapses between ‘off’ bipolar cells, ganglion cells and some amacrines; a middle or ‘on’ layer contains synapses between the axons of ‘on’ bipolars and the dendrites of ganglion cells and displaced amacrines; and an inner ‘rod’ layer contains synapses between rod bipolars and displaced amacrines. (Refer to Wässle and Boycott 1991 for an explanation of the ‘off’ and ‘on’ cell designations.)
Cells of the Retina
Retinal Pigment Epithelial Cells
The retinal pigment epithelial cells are low cuboidal cells that form a single continuous layer, extending from the periphery of the optic disc to the ora serrata and continue from there into the ciliary epithelium. They are flat in radial section and hexagonal or pentagonal in surface view. There are about 4 million to 6 million in the human retina. Their cytoplasm contains numerous melanin granules (Fig. 12.9). Apically (toward the rods and cones), the cells bear long (5 to 7 mm) microvilli that contact or project between the outer ends of rod and cone processes. The tips of rod outer segments are deeply inserted into invaginations in the apical membrane. The attachments are unsupported by junctional complexes and are broken in the clinical condition of retinal detachment arising from trauma or disease processes.
Cone and Rod Cells
The cone and rod cells are the retinal photoreceptor cells (Fig. 12.10). They are long, radially oriented structures with a cylindrical photoreceptive portion at the end nearest to the pigment epithelium and synaptic contacts at the other end, within the outer plexiform layer. Both types of cells have a similar organization, although their details differ. From the external (choroidal) end inward, the cells consist of outer and inner segments, a cell body containing the nucleus and either a cone pedicle or a rod spherule (depending on cell type); this is an area of synaptic contact with adjacent bipolar and horizontal cells and with other cone or rod cells. The outer and inner segments together form a cone process or a rod process (it should be noted that the terms cone and rod are often loosely applied to the whole cell); the cone process is wider but tapers (hence the name), whereas the rod processes are cylindrical. The outer and inner segments are connected by a short cilium.
Cone cells are chiefly responsible for high spatial resolution and colour vision in good lighting conditions (photopic vision). Rod cells provide high monochromatic sensitivity to a much wider range of illumination down to much lower intensities (scotopic vision), although with relatively low spatial discrimination because of their different neural connections. Cone cells are of three types—red, green and blue—according to their maximal spectral sensitivities. They are highly concentrated in the centre of the retina (the fovea), where visual acuity is greatest, but they populate the whole retina, intermingled with rods, as far as its neural edge. Rods are excluded from the fovea. The total number of rods in the human retina has been estimated at 110 million to 125 million, and cones at 63 million to 68 million (Osterberg 1935).
Bipolar Cells
Bipolar cells are radially oriented neurones, each with one or more dendrites that synapse with cones or rods and horizontal cells and interplexiform cells in the outer plexiform layer. Their somata are located in the inner nuclear layer, and axonal branches given off in the inner plexiform layer synapse with dendrites of ganglion cells or amacrine cells (see Fig. 12.8). Cone bipolars are of three major types—midget, blue cone or diffuse—according to their connectivity and size. As their name implies, midget cone bipolars are small cells, and each one is part of a single one-to-one channel from cone to ganglion cell; they are thought to mediate high spatial resolution. Blue cones have similar connectivity and selectively form part of a short wavelength-mediating channel. The larger diffuse cone bipolars are connected to up to 10 cones and are thought to signal luminosity rather than colour. Rod bipolars receive direct photoreceptive inputs from many rods and relate to ganglion cells indirectly via a synapse with amacrine cells.
Horizontal Cells
Horizontal cells (see Fig. 12.8) are inhibitory interneurones whose dendrites and axons extend within the outer plexiform layer, making synaptic contacts with the bases of cones and rods and, via gap junctions at the tips of their dendrites, with one another. Their cell bodies lie in the outer part of the inner nuclear layer. Because of their interactions with photoreceptor cells and bipolar cells, horizontal cells create inhibitory surrounds. When illumination of a photoreceptor cluster with a point of light causes depolarization of synaptically connected ‘on’ bipolars at its bright centre, horizontal cell dendrites cause inhibition at the edge of the illuminated area, thus sharpening contrast and maximizing spatial resolution.
Amacrine Cells
Amacrine cells (see Fig. 12.8) lack typical axons, but their dendrites function as axons and dendrites and make both incoming and outgoing synapses. Each neurone has a cell body in the inner nuclear layer; near its boundary with the inner plexiform layer; or on the outer aspect of the ganglion cell layer, where they are known as displaced amacrine cells. The processes of amacrine neurones make scattered chemical synaptic contacts with the axons of bipolar cells, dendrites (and possibly axons) of ganglion cells and the processes of other amacrine cells. They also receive numerous synapses from bipolar cells. Some amacrine cells form electrical synapses with bipolar cells.
Ganglion Cells
Ganglion cells (see Fig. 12.8) are the final common pathway neurones of the retina. Their dendrites are synaptically connected with processes of bipolar and amacrine neurones in the inner plexiform layer, and their axons are likewise connected with neurones in the central nervous system. Their axons form the layer of nerve fibres on the inner surface of the retina. They turn tangentially to the optic disc, through which they leave the eye as fibres of the optic nerve. The axons are subsequently distributed to various parts of the brain, including the lateral geniculate nucleus, pretectal area and superior colliculus of the midbrain; the thalamic pulvinar; and the accessory optic system.
Retinal Glial Cells
Retinal glial cells are of three types: radial (Müller) cells, astrocytes and microglia. Radial glial cells are the predominant glial element in most of the retina. Retinal astrocytes are largely confined to the ganglion cell and nerve fibre layers. Microglial cells are scattered throughout the neural part of the retina in small numbers (see Fig. 12.8).
Radial glial cells span the entire thickness of the neural retina. They ensheathe and separate the various photoreceptive and neural cells, except at synaptic sites. They form the outer boundary of the retinal tissue at the level of the inner rod and cone segments and the inner boundary at the internal limiting membrane. Their nuclei lie within the inner nuclear layer, and from this region, a single thick fibre ascends radially, giving off complex lateral lamellae that branch among the processes of the outer plexiform layer. Apically, the central process terminates in a surface from which microvilli project into the space between the rod and cone processes. Just beneath this area, the radial glial cells form a line of dense zonulae adherentes with one another and with receptor inner segments, thus forming the external limiting membrane. On the inner aspect of the retina, the main radial glial cell process expands in a terminal footplate that contacts those of neighbouring radial glial cells and astrocytes and attaches to the internal limiting membrane. Like other neuroglia, radial glia also contact blood vessels, especially capillaries, and their basal laminae fuse with those of the vascular smooth muscle in the media of larger vessels or of the endothelia lining capillaries. These extensive neuroglial cells form much of the total retinal volume and almost totally fill the extracellular space between neural elements. Their functions appear to be similar to those of astrocytes—that is, maintenance of the stability of the retinal extracellular environment by ionic transport, uptake of neurotransmitter, removal of debris, storage of glycogen, electrical insulation of receptors and neurones and mechanical support of the neural retina.
Optic Disc and Retinal Blood Vessels
Optic Disc
The optic disc is the region where retinal tissues meet the neural and glial elements of the optic nerve and the connective tissues of the sclera and meninges (see Figs 12.2, 12.4, 12.5). It is the exit point for the optic nerve fibres and a point of entry and exit for the retinal circulation. It is the only site where anastomoses occur with other arteries (the posterior ciliary arteries). It is visible, by ophthalmoscopy, and is a region of much clinical importance; it is here that the central vessels can be inspected directly—the only vessels so accessible in the whole body. Oedema of the disc (papilloedema) may be the first sign of raised intracranial pressure, which is transmitted into the subarachnoid space around the optic nerve and compresses the central retinal vein where it crosses the space.
The optic disc is superomedial to the posterior pole of the eye, so it lies away from the visual axis. It is round or oval, usually approximately 1.6 mm in transverse diameter and 1.8 mm in vertical diameter, and its appearance is very variable (for details, see Jonas, Gusek and Naumann 1988). In light-skinned subjects, the general retinal hue is a bright terra-cotta red, with which the pale pink of the disc contrasts sharply; its central part is usually even paler and may be light grey. These differences are due in part to the degree of vascularization of the two regions, which is much less at the optic disc; it is also due to the total absence of choroidal or retinal pigment cells, because the retina is represented in the disc by little more than the internal limiting membrane. In subjects with strongly melanized skin, both the retina and disc are darker (see Fig. 12.4B). The optic disc does not project at all in many eyes, and rarely does it project sufficiently to justify the term papilla. It is usually a little elevated on its lateral side, where the papillomacular nerve fibres turn into the optic nerve. There is usually a slight depression where the retinal vessels traverse its centre.
Visual Field Defects
Plotting visual field loss frequently reveals the approximate location of the causative lesion in the visual pathway and sometimes its nature (Fig. 12.11). Because retinal lesions can be visualized using an ophthalmoscope, these aids might appear to be redundant, but visual field measurement is still helpful in assessing the extent of the damage and may be the key factor in confirming a diagnosis. Glaucoma serves as an example. Field defects in glaucoma, occurring as a consequence of damage to the nerve fibre bundles at the optic nerve head, may be detectable ophthalmoscopically, but confirmation of the diagnosis frequently depends on field assessment. An initial constriction of the visual field is of little clinical significance, but later defects, characteristic of the disease, consist of a scotoma between 10 and 20 degrees of the fixation area, extending upward or, less commonly, downward from the blind spot. This later elongates circumferentially along the arcuate nerve fibres and subsequently extends farther. The field defect forms a linear limit or step along the horizontal meridian nasally; the loss continues, ultimately resulting in blindness.
With regard to the location of lesions central to the retina, deficits in the vision of one eye are usually attributable to optic nerve lesions. Lesions of the optic chiasma, involving crossing nerve fibres, produce a bilateral field loss, as exemplified by pituitary adenoma. The tumour expands upward from the pituitary fossa, compressing the inferior midline of the chiasma, and eventually produces bitemporal hemianopia, starting with an early loss in the upper temporal quadrants. Field defects in the rare cases of optic tract lesions are distinctive. The tract contains contralateral nasal and ipsilateral temporal retinal projections, and damage causes a homonymous contralateral loss of field with substantial incongruity (dissimilar defects in the two fields). Incongruity probably results from a delay in achieving coincidence between retinal topographical projections of the two inputs of the visual pathway; as contiguous projections adjust their location, they gradually achieve coincidence. It also likely reflects the normal reorganization of fibres that occurs in the optic tracts, as some fibres leave the tract in the superior brachium and others progress to the lateral geniculate nucleus. The two defective fields may display incongruity as a result of lesions above the level of the chiasma. Incongruity is most marked in optic tract defects, less obvious in optic radiation defects and usually absent in cortically induced field defects, thus providing an additional clue to the location of the cause.
CASE 3 Optic Neuritis
A 26-year-old woman presents with a 2-day history of progressive loss of vision in her left eye, with accompanying left orbital pain, especially on eye movement. At the onset she noted blurring of vision. She performed a monocular cover test on herself and discovered that the blurring was confined to the left eye. She noted that when she looked at a computer screen with her left eye it became ‘black and white.’ Her visual loss stabilized after several days; she ultimately observed that she could see ‘all around’ a central visual loss in the left eye. It is noteworthy that 1 year before she had an episode of numbness and tingling sensations affecting her left arm and leg, lasting about 6 weeks. She did not seek medical attention at that time.
Discussion: Progressive loss of central and colour vision over several days in association with orbital pain and normal fundi points to a left retro-orbital optic neuropathy, with clinical features of a Marcus Gunn pupil. Acutely there may be disc swelling and, with time, disc pallor may occur. These findings associated with aterial attenuation suggest acute ischemic optic neuropathy (Fig. 12.12). The observation of a central scotoma with intact peripheral visual fields emphasizes that about 90% of the axons in the optic nerve subserve macular vision.
CASE 4 Superior Visual Field Disturbance
Discussion: The ‘pie in the sky’ or wedge-shaped visual field defect noted in this patient is not uncommon following temporal lobectomy for epilepsy. Rostral geniculocalcarine pathway lesions produce incongruous visual field defects—that is, defects that are not exactly the same in both eyes—because geniculocalcarine fibres from corresponding retinal areas are not anatomically in close proximity until reaching the occipital lobe. Occipital lobe lesions, in contrast, produce congruous (i.e. identical) field defects that can be quadrantal. Because of the anatomical inversion of the visual pathways, inferiorly placed lesions in the optic tract or calcarine cortex tend to produce superior quadrantal defects, whereas inferior defects are consistent with lesions in the parietal lobe involving the tract from above. This case also demonstrates that visual field disturbances, particularly in the superior field, may go unnoticed by the patient. (See Fig. 12.11, optic radiation, lower division.)
CASE 5 Homonymous Hemianopia
It is noteworthy that the patient’s visual field testing showed macular sparing. For many years, there has been debate whether such macular sparing has an underlying anatomical explanation, such as bilateral cortical representation of the macula or a dual blood supply, or whether this phenomenon is merely the result of slight horizontal movement of the eyes during field testing. The most commonly accepted explanation is the latter. (See Fig. 12.11, optic radiation, both divisions.)
Neural Control of Gaze
Neural control systems are required to coordinate the movements of the eyes so that the image of the object of interest is simultaneously held on both foveae, despite movement of the object or the observer. A number of separate neural systems are involved: first, to shift gaze to the object of interest using rapid movements, called saccades; and second, to stabilize the image on the fovea either during movement of the object of interest (smooth pursuit system) or during movement of the head or body (vestibulo-ocular and optokinetic systems). Although the detailed anatomical substrates for these systems differ, they share common circuitry that lies mainly in the pons and midbrain for horizontal and vertical gaze movements, respectively (Fig. 12.13).
The gaze motor command involves specialized areas of the reticular formation of the brain stem that receive a variety of supranuclear inputs. The main region for horizontal gaze is the paramedian pontine reticular formation (PPRF), which is located on each side of the midline in the central paramedian part of the tegmentum and extends from the pontomedullary junction to the pontopeduncular junction. Each PPRF contains excitatory neurones that discharge at high frequencies just before and during ipsilateral saccades. Pause neurones, which are located in a midline caudal pontine nucleus called the nucleus raphe interpositus, discharge tonically except just before and during saccades. They appear to exert an inhibitory influence on the burst neurones and thus prevent extraneous saccades occurring during fixation.
The final common pathway of vertical gaze movements is formed by the oculomotor and trochlear nuclei. The rostral interstitial nucleus of the MLF (riMLF) contains neurones that discharge in relation to up-and-down vertical saccadic movements. The riMLF projects through the posterior commissure to its equivalent on the other side of the mesencephalon, as well as directly to the oculomotor nucleus. Therefore, lesions within the posterior commissure give rise to disturbance in vertical gaze, especially up-gaze (see Ch. 10, Case 9). Lesions located more ventrally in the region of the riMLF give rise to vertical gaze disorders that may be mixed up and down or mainly down-gaze. Slightly caudal to the riMLF, and directly connected to it, lies the interstitial nucleus of Cajal. It contains neurones that appear to be involved in vertical gaze by holding the vertical pursuit.
The cerebral hemispheres are extremely important for the programming and coordination of both saccadic and pursuit conjugate eye movements. There appear to be four main cortical areas in the cerebral hemispheres involved in the generation of saccades (see Fig. 12.13): the frontal eye field, which lies laterally at the caudal end of the second frontal gyrus in the premotor cortex (Brodmann’s area 8); the supplementary eye field, which lies at the anterior region of the supplementary motor area in the first frontal gyrus (Brodmann’s area 6); the dorsolateral prefrontal cortex, which lies anterior to the frontal eye field in the second frontal gyrus (Brodmann’s area 46); and the posterior eye field, which lies in the parietal lobe, possibly in the superior part of the angular gyrus (Brodmann’s area 39), and in the adjacent lateral intraparietal sulcus. These areas are apparently interconnected and send projections to the superior colliculus and the brain stem areas controlling saccades.
Pupillary Light Reflex
The pupillary light reflex is a dynamic system for controlling the amount of light reaching the retina (Fig. 12.14). Illumination of the retina causes reflex constriction of the pupil (miosis). The direct component of the light reflex mediates constriction of the pupil of the ipsilateral eye, and the consensual component elicits simultaneous constriction of the contralateral pupil.
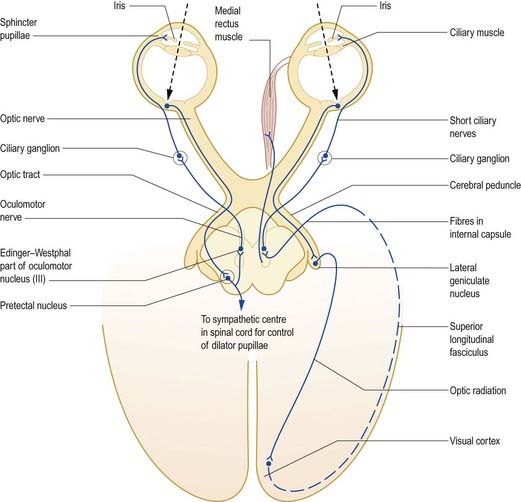
Fig. 12.14 Pupillary light reflex and accommodation reflex.
(From MacKinnon, P., Morris, J. [Eds.], 1990. Oxford Textbook of Functional Anatomy, vol 3, Head and Neck. Oxford University Press, Oxford. By permission of Oxford University Press.)
There is also a connection to the spinal sympathetic centre controlling the dilator pupillae. The preganglionic fibres arise from neurones in the lateral column of the first and second thoracic segments and pass via the sympathetic trunk to the superior cervical ganglion, where they synapse on postganglionic neurones. Postganglionic fibres arising from these neurones are distributed to the cavernous plexus; from there, they travel mainly through the long ciliary nerves to the anterior part of the eye, where they supply the dilator pupillae.
Hearing
The primary afferents of the auditory pathway arise from cell bodies in the spiral ganglion of the cochlea (see Figs. 11.20, 11.23, 11.28, 11.31). The axons constitute the auditory component of the vestibulocochlear nerve, which enters the brain stem at the cerebellopontine angle. Afferent fibres bifurcate and terminate in the dorsal and ventral cochlear nuclei. Onward connections make up the ascending auditory pathway (Fig. 12.15). The dorsal cochlear nucleus projects via the dorsal acoustic stria to the contralateral inferior colliculus. The ventral cochlear nucleus projects via the trapezoid body or the intermediate acoustic stria to relay centres in the superior olivary complex, the nuclei of the lateral lemniscus or the inferior colliculus. The superior olivary complex is dominated by the medial superior olivary nucleus, which receives direct input from the ventral cochlear nucleus on both sides, and is involved in localization of sound by measuring the time difference between afferent impulses arriving from the two ears.
The inferior colliculus consists of a central nucleus and two cortical areas. The dorsal cortex lies dorsomedially, and the external cortex lies ventromedially. Secondary and tertiary fibres ascend in the lateral lemniscus. They converge in the central nucleus, which projects to the ventral division of the medial geniculate body of the thalamus. The external cortex receives both auditory and somatosensory input. It projects to the medial division of the medial geniculate body and, together with the central nucleus, also projects to olivocochlear cells in the superior olivary complex and to cells in the cochlear nuclei. The dorsal cortex receives an input from the auditory cortex and projects to the dorsal division of the medial geniculate body. Connections also run from the nucleus of the lateral lemniscus to the deep part of the superior colliculus, to coordinate auditory and visual responses.
Balance
Functionally, the vestibular apparatus is customarily divided into two components: the kinetic labyrinth, which provides information about acceleration and deceleration of the head, and the static labyrinth, which detects the orientation of the head in relation to the pull of gravity. In terms of structure, the kinetic labyrinth consists of the semicircular canals and their ampullary cristae, and the static labyrinth consists of the maculae of the utricle and saccule. However, the saccular macula also responds to head movements, and both maculae can be stimulated by low-frequency sound and may therefore have minor auditory functions (see Fig. 11.28).
Another major function of the vestibular system is the control of visual reflexes, which allows the fixation of gaze on an object in spite of movements of the head and requires coordinated movements of the eyes, neck and upper trunk. Constant adjustments of the visual axes are achieved chiefly through the MLF, which connects the vestibular nuclear complex with neurones in the oculomotor, trochlear and abducens nuclei and with upper spinal motor neurones (see Fig. 10.26), as well as by the vestibulospinal tracts.
Cagan R.H., editor. Neural Mechanisms in Taste. Boca Raton, FL: CRC Press, 1989.
Hubel, D.H., 1988. Eye brain and vision. Scientific American Library Series No. 22
Jonas, Gusek, Naumann, 1988Jonas J.B. Gusek G.C. Naumann G.O. Optic disc cup and neuroretinal rim size configuration and correlations in normal eyes. Invest. Ophthalmol. Vis. Sci.. 29:1988;1151-1158.
A clinical template of normal range.
Kandel E.R., Schwartz J.H., Jessel T.M., editors. Principles of Neural Science, fourth ed, New York: McGraw-Hill, 2000.
Oertel D., Fay R.R., Popper A.N., editors. Integrative Functions in the Mammalian Auditory Pathway. Springer Handbook of Auditory Research, vol. 15. New York: Springer, 2002.
Osterberg G.A. Topography of the layers of the rods and cones in the human retina. Acta. Ophthalmol.. (Suppl. 6):1935.
Savino P.J., Danesh-Meyer H.V. Color atlas and synopsis of clinical ophthalmology, Wills Eye Hospital. New York: McGraw-Hill; 2003.
Victor M., Dreyfus P.M., Mancall E.L. Deficiency amblyopia in the alcoholic patient. Arch. Ophthalmol.. 1960;64:1.
Wässle, Boycott, 1991Wässle H. Boycott B.B. Functional architecture of the mammalian retina. Physiol. Rev.. 71:1991;447-480.
Unitary cell responses of the various retinal neurones and their connectivity.
Zeki S. A Vision of the Brain. Oxford: Blackwell Scientific; 1993.