Chapter 114 Space Medicine
The New Frontier
For online-only figures, please go to www.expertconsult.com
If space is, as one Doctor McCoy might suggest, the “final frontier,” then space medicine largely remains the “undiscovered country.” Relatively little is known about how the human body adapts to and recovers from long-term exposure to microgravity (notated as “µG” or, for many practical purposes, “0G”), let alone the best ways to treat illness or injury that occurs in space. Even less is known about how the less screened and minimally trained tourist population will be affected by short- or long-duration spaceflight with the anticipated advent of commercial space operations.41,59
Historical Perspective—X-15 To The Iss And Beyond
There have been very few suborbital* flights in the history of human space flight (Table 114-1, A). These early flights in the “Space Race” demonstrated human survivability in the space environment and tested early technology. Once these objectives were accomplished, attention shifted to orbital and lunar flights.
During the Mercury program, life sciences questions tended to focus purely on survivability in the space environment and ignored many of the normal physiologic functions deemed not (yet) relevant. For example, Alan Shepard’s spacesuit for the first Mercury flight Freedom 7 (Figure 114-1 and Table 114-1, B, online) didn’t even provide him with a way to relieve himself (a perhaps forgivable oversight, because the flight was only scheduled to last 15 minutes; unfortunately there was a lengthy delay on the launch pad).61 It was not until the NASA Mercury Project MA-6 mission of John H. Glenn that blood pressure readings were taken.
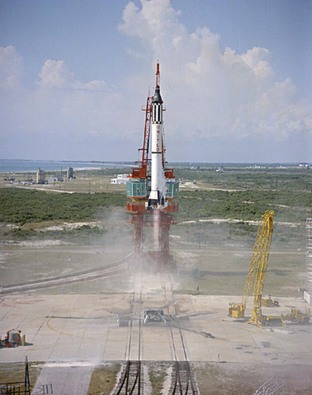
(From NASA Image Exchange. http://nix.larc.nasa.gov/info;jsessionid=1snd99vh206gk?id=GPN-2000-000859&orgid=12.)
TABLE 114-1, B Space Exploration Vehicles and Crew Compliment
Vehicle | Crewmembers Per Mission | Mission |
---|---|---|
Mercury | 1 | Orbital |
Gemini | 2 | Orbital with rendezvous and docking tests |
Vostok | 1 | Orbital with rendezvous tests |
Voskhod | 2-3 | Orbital |
Apollo | 3 | Orbital, Moon landing |
Soyuz | 3 | Orbital with docking to Salyut/Mir/ISS |
Apollo Soyuz Test Program | 5 total | Orbital with docking |
Skylab | 3 | Orbital Station |
Salyut | 2-3 | Orbital Station |
Shuttle | 2-8 | Orbital |
Mir | 3 | Orbital Station |
ISS | 2-6 | Orbital Station |
Shuttle/ISS | 6-13 | Docking |
Shenzhou | 1-2 | Orbital |
Note: All ISS missions have had visiting vehicles, increasing the crewmembers to a maximum of 13 in March 2009 with the simultaneous docking of STS 119 and Soyuz TMA 14.
All six NASA Mercury crewmembers returned to Earth in healthy condition. They were able to conduct complex visual-motor coordination tasks proficiently in the weightless state, and no evidence of physiologic dysfunction occurred during the flights. The principle findings were weight loss due to dehydration with mild cardiovascular impairment. There was some indication of post-flight orthostatic intolerance and hemoconcentration; however, frank signs of orthostatic hypotension were not noted in these suborbital flights.53,96
The main physiologic effects experienced and observed in the NASA Gemini missions were:71
The Apollo program findings were similar to those of Gemini, with the addition of:51
Medical Challenges of Spaceflight
Time Course Of Changes And Adaptation To Microgravity
The physiology of the space traveler is most labile during the transition to or from microgravity. Within a few days, the body adapts to its new environment (as described later), but during the first 72 hours following a change to or from microgravity, most of the physiologic processes are in a state of flux. With space tourists on suborbital flights, there may be a rise in medical events because of subclinical conditions that may not be discovered (or admitted to) preflight.41,59
Long-Term Effects
Several physiologic systems exhibit microgravity-related effects over a longer time frame (weeks to months) (Figure 114-2). For short-duration missions, these changes may be minor or even undetectable, but on longer flights, the effects can become pronounced. In some systems, it is unclear whether a “space normal” equilibrium is ever achieved, or whether changes continue so long as the crewmember remains in microgravity. Examples include alterations in red cell mass and bone demineralization.
Effects On Human Physiology
Effects on the Cardiovascular and Pulmonary Systems
The cardiovascular system undergoes several predictable adaptations in response to microgravity. Recalibration of baroreceptor homeostasis occurs after several days in space, likely due to cephalad fluid shifts induced by the microgravity environment27 (Figure 114-3). As a result of these shifts, the carotid baroreceptors sense a (relative) hypervolemia and cause a diuresis. Relative hypovolemia (approximately 10% decrease in total body fluid and a fall in central venous pressure (CVP) from a terrestrial average of 7 to 10 mm Hg to 0 to 2 mm Hg) then exists compared with preflight terrestrial fluid status.15 The fluid shift may also be associated with development of space motion sickness (SMS), with resultant nausea and vomiting that lead to further loss of fluids.
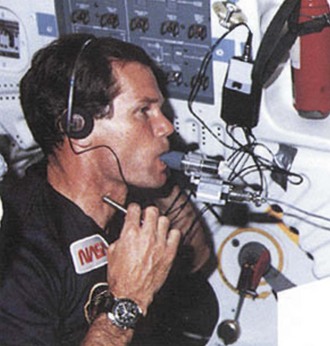
FIGURE 114-3 The cephalad fluid shift is measured by determining venous pressure in the jugular vein.
(From NP-119 Science in Orbit: The Shuttle & Spacelab Experience, 1981-1986. http://history.nasa.gov/NP-119/ch2.htm.)
Although there is still debate on the effects of microgravity on hemodynamic parameters, the most recent data suggest that heart rate and diastolic blood pressure decrease and cardiac output increases. This implies reduction in peripheral vascular resistance and a similar decrease in sympathetic tone. Pulmonary physiology testing has revealed a decrease in tidal volume and an increase in respiratory rate.102 There is also a decrease in dead space and improved CO2 diffusion capacity, possibly because of increased intrathoracic perfusion.83
Although these changes have not been demonstrated to affect human health or cardiovascular stamina during spaceflight, orthostatic intolerance following return to Earth’s gravity remains a concern. Compounding this effect may be a loss of adrenergic responsiveness; in a number of returning astronauts, lower norepinephrine levels have been found to correlate with deficient responses to orthostatic stress.15,88
The severity of orthostasis is generally proportional to the duration of time spent in space and poses a problem if an emergency situation requires crew mobility immediately on landing, especially following a long-duration flight.11 It is also a significant obstacle to planning a mission to Mars, where the crew will be required to function independently immediately on entering a -G gravity environment after several months’ travel in microgravity. Engineering solutions to these problems may necessitate the use of fully automated landing systems.
Several strategies are currently used as countermeasures against postflight cardiovascular dysfunction in the fit astronaut population. Five-bladder anti-G suits and liquid cooling garments (LCGs) have become standard equipment during landing, after experience from short-duration Shuttle flights demonstrated that they reduced orthostatic hypotension on landing. The former presumably is effective by minimizing venous pooling in the extremities, whereas the latter reduces heat stress, sweating, and dehydration during the high cabin temperatures of reentry.22 Another standard protocol is oral fluid loading with isotonic solutions (such as bouillon or a sports drink–like concoction called Astro-Ade) performed 2 hours prior to landing. This is a short-acting measure to mitigate the relative hypovolemia of spaceflight.
The Russians also employ another in-flight countermeasure to improve postflight orthostatic intolerance: a lower body negative pressure (LBNP) device (or Russian “Chibis” suit) (Figure 114-4, online). This device draws blood away from the central circulation and into the lower extremities through a negative pressure gradient, simulating a gravitational stress on the cardiovascular system.25 The Russians have also employed “brazlets,” or thigh cuffs, to encourage greater blood distribution in the lower extremities. Fortunately, even severe postflight orthostatic intolerance is a temporary event and within a few days, virtually all long-duration flight crews are able to mount an appropriate response to orthostatic stress.
Effects on the Neurovestibular and Sensory Systems
One of the earliest effects of these changes is SMS. This form of motion sickness, associated with exposure to microgravity, often manifests as headache, nausea, vomiting, anorexia, poor concentration, or general malaise. It is usually a minor and self-limited condition (generally lasting for the first 1 to 2 days in orbit), yet it is one of the most common reasons for pharmacologic intervention in space (most commonly treated with parenteral or rectal promethazine, 25 to 50 mg, administered at bedtime on the first in-flight day).83 On occasion, however, SMS has persisted for the duration of a Shuttle flight, significantly impairing the affected crewmember’s performance.
SMS is believed to be related to fluid redistribution patterns and/or alterations in bowel motility. It is only weakly correlated with the motion sickness associated with ship or air travel. The motion sickness experienced during parabolic flights on NASA’s DC-8 Reduced Gravity Research Program (Figure 114-5), during which passengers experience intervals of microgravity, has been anecdotally reported to correlate poorly with in-flight SMS.34,94
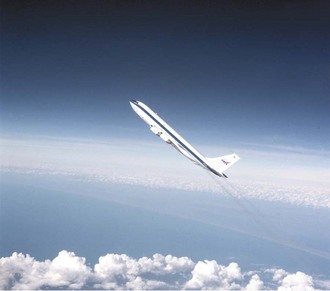
(From NASA Image Exchange. http://nix.ksc.nasa.gov/info;jsessionid=31kakwlvw0uun?id=MSFC-0001123&orgid=11.)
SMS affects more than 70% of space travelers. Reliable prediction of its occurrence (particularly in first-time flyers) is difficult.22 The incidence is somewhat lower among repeat flyers, suggesting some training effect.5
Even though SMS can be expected to have resolved several days into a mission, other neurovestibular and neurosensory changes persist, including alterations in eye–head coordination, target tracking, and optokinetic reflex function.5,27 These adaptations can significantly affect delicate technical operations, such as manipulation of the robotic arm or manually controlled docking maneuvers. In fact, neurovestibular dysfunction was implicated as one cause in the collision of the Russian space station Mir with a Progress resupply vessel in 1997, as well as the “bumping” of a satellite during a capture attempt by the Shuttle’s robotic arm.
About 80% of space travelers experience perceptual illusions during or after flight. Several different types have been reported: illusory self-motion (both linear and rotational), a sensation of the floor dropping when doing a squat to stand, the sensation of things floating in space, visual streaming (blurring), visual scene oscillation (oscillopsia), object position distortion, visual axis distortion (tilting or inversion), and platform stability illusion. Some crewmembers also experience a sense of being upside-down early in spaceflight.5 The term “EVA acrophobia” has been coined by spacewalking astronauts to describe a feeling that they might “fall off” their vehicle or “fall to Earth” (Figure 114-6).
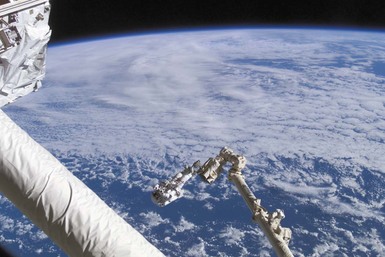
(From NASA Human Space Flight Gallery. http://spaceflight.nasa.gov/gallery/images/shuttle/sts-111/html/s111e5132.html.)
Restoring sensory references is one goal of neurovestibular countermeasures. Research is currently underway using preflight virtual reality training to simulate conflicts between the proprioceptive and visual systems and to help flyers become accustomed to the ISS geometry prior to arrival. ISS modules also have fluorescent directional guides and standardized coloration of “floor” and “ceiling” surfaces.28,99
On return to Earth, the nervous system readapts to a 1G field remarkably well, often returning to preflight states within 48 hours. However, most crewmembers experience some postflight neurovestibular symptoms, including nausea, illusory movements, clumsiness, and vertigo.7 These symptoms are usually mild, but in the event of an emergency egress or bailout over the water, they could prove dangerous.7 Crewmembers in such an emergency not only will have to overcome any musculoskeletal and aerobic deconditioning, but are also likely to have to cope with unsteadiness, poor coordination, vertigo, and motion sickness. Pharmacologic prophylaxis (anti–motion sickness medications) were used for landings during the Skylab program, but were ultimately felt to be counterproductive because of side-effect profiles that included sedation. With short-duration suborbital flights, even mild SMS might significantly detract from the enjoyment for commercial space travelers.
Effects on the Musculoskeletal System
With exposure to microgravity, mechanical load on the musculoskeletal system lessens dramatically, leading to muscle atrophy (particularly in the postural/antigravity muscles) and bone demineralization (disuse osteoporosis). The latter is one of the high priorities of space physiology research, because osteoporosis remains a limiting factor for astronaut health during and after long-duration flight.75 Changes are seen within the first few days of space flight and continue throughout exposure to microgravity, although they do not immediately become clinically significant.
To that end, a robust countermeasures exercise program has been initiated on the ISS, making use of both strength and aerobic training. Because enthusiasm for exercise (and compliance with the scheduled program) varies from person to person, it is important for the aerospace physician to educate space travelers about the need for regular exercise, as well as to monitor fitness and strength levels during the course of a mission (see http://www.asc-csa.gc.ca/eng/astronauts/living_exercising.asp).
Mechanical countermeasures, such as low-intensity vibrations (to stimulate bone maintenance) or the Russian “penguin suit” (which makes use of elastic bands to force use of extensor muscles) (Figure 114-7, online), have been studied to determine efficacy. Unfortunately, none has as yet proved sufficiently useful to warrant routine use.11 Pharmacologic and nutritional interventions, such as bisphosphonates and other antiosteoclastic agents or high-calcium diets, are currently under investigation as countermeasures, but none is (yet) routinely used. One concern is that use of drugs to alter calcium balance may have unintentional effects on renal physiology and enhance creation of kidney stones. Artificial gravity infrastructures could some day offer a solution to this problem, but current designs remain impractical for space travel in the near future.72
Effects on the Gastrointestinal and Genitourinary Systems
Weight loss, dehydration, and anorexia are common gastrointestinal symptoms during spaceflight.22 Reduced gastric emptying and altered intestinal transport time have been documented.27,32 Weight loss, likely augmented by fluid losses and muscle atrophy, may also be related to negative nitrogen balance, possibly because of persistent protein loss. Anorexia may be related to altered taste sensation, perhaps because of cephalad fluid shifts. Increased stressors and time pressures associated with mission objectives may influence food choices, with snack or “handheld” foods being preferred as full meals.
Regarding genitourinary changes, there is a well-documented increase in the incidence of renal stone formation. Microgravity-induced increases in bone metabolism contribute to increased calcium excretion in the urine, with a stone incidence of up to 5%.11 To prevent renal colic, the current hydration recommendation for crewmembers is to exceed 2.5 L per day.46 Persistent bone loss during extended missions (and the increased risk of renal stones) remains a significant obstacle to planning a Mars mission.
The missions to date have yielded minimal data on the male and female reproductive systems. Reversible reductions in male testosterone levels have been reported, whereas the phenomenon of retrograde menstrual flow requires further elucidation. Radiation exposure remains a concern as well. The implications of these findings for fertility following a mission have led some experts to endorse preflight preservation of gamete cells. Furthermore, though admittedly sparse, research to date suggests that successful reproduction away from Earth may be significantly difficult, if not impossible, which has major implications for any viable colonization strategy.3,12,98
Effects on the Immune System
Although studies to date have produced conflicting results, anecdotal data persistently indicate that immune function is compromised in microgravity. In vitro studies show that lymphocytes in-flight are largely unaffected by stimulating agents, suggesting that the white blood cells circulating in the blood, although more numerous than terrestrial levels, may be unable to mount an effective immune response.21,153,154 Changes in leukocyte morphology have also been reported, further suggesting that immune function may be impaired (Figure 114-8, online).
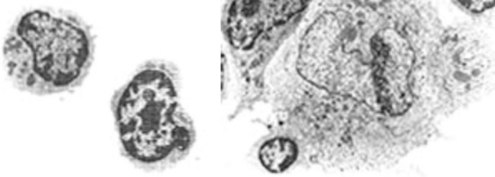
(From NP-119 Science in Orbit: The Shuttle & Spacelab Experience, 1981-1986. http://history.nasa.gov/NP-119/ch2.htm.)
Subjects in analog environments, such as crews spending the winter in Antarctica, often display latent viral reactivation,54 so it is not unreasonable to imagine that such an effect could be present in long-duration space flight.
Effects on the Blood, Fluid, and Electrolyte Balance
There is in-flight loss of total body fluids, including extracellular fluid volume, plasma volume, and circulating blood volume.34 The majority of this volume loss is due to changes in plasma volume and circulating red cell mass.
Blood sodium levels decrease in spaceflight, although the relative ratio between sodium and potassium remains unchanged.34 Most of the other electrolytes are unaffected by exposure to space, with the one major exception being calcium. Both urine and plasma levels of calcium are increased in conjunction with bone demineralization, whereas negative nitrogen balance and muscle atrophy lead to elevated urinary levels of nitrogen and muscle breakdown products. Phosphorus levels mimic those of calcium and are also felt to be due to changes in bone metabolism.
Stressful Environment: Psychological and Behavioral Issues
NASA has identified a number of behavioral issues that are thought to have a direct bearing on productivity and habitability during routine operations onboard a permanently manned space station:68,102
Studies show that isolated and confined populations frequently demonstrate mild psychiatric symptoms, including depression, anxiety, increased defensiveness, and belligerence.18 It has also been suggested that prolonged isolation and confinement harms group dynamics, with social irritability reported among polar expeditioners, submariners, and space-simulator subjects.18
Interpersonal conflict is a real and concerning threat to crew health and mission performance.39 Cramped and confined spaces, cultural differences (often including language differences), and busy schedules replete with complex physical and mental tasks can often exacerbate stressed relationships. Ground support currently plays a large role in monitoring and providing intervention for crewmember strife, but this strategy will need modification for a mission to Mars, where transmission time delays can be as long as 40 minutes round-trip. This delay will not only diminish reliance on ground support for problem solving but may increase tensions between the crew and mission control. History is replete with strife between those on the “front” and the decision makers in the rear. During World War II, submariners on 2- to 3-month patrols complained about the “unrealistic” orders sent from rear echelon officers thousands of miles away. There have already been reports of animosity toward mission control from astronauts on long-duration missions, most famously during the first Skylab Mission. Indeed, ISS initially presented similar issues with unrealistic task timelines for crewmembers; much more emphasis is now placed on “free time” for crewmembers.
The main psychological effects on space travelers can be summarized as:43
Lack of Privacy
Privacy (or the lack of it) is a feature not only of space exploration but also of other stressful environments. Shuttle crews are arguably at more of a disadvantage than are ISS crews, despite their shorter mission duration. The larger crew size and smaller habitable volume force Shuttle crews to live in extremely close quarters (Figure 114-9). By contrast, the larger ISS, even with its six-person crew, affords significantly more privacy and personal space, with crewmembers having individual “sleeping” quarters in the various modules of the ISS. That said, the tiny crew complement ensures that there are few secrets on board.
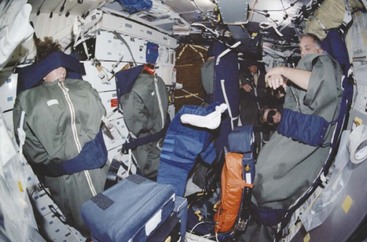
FIGURE 114-9 Sleeping astronauts on the crowded Shuttle middeck demonstrate the lack of privacy on board.
(From NASA Human Space Flight Gallery. http://spaceflight.nasa.gov/gallery/images/shuttle/sts-112/lores/sts112-345-028.jpg.)
Privacy may well become an even greater issue in interplanetary travel, with long transit times and larger crews. Unfortunately, the vehicle may not have the size to allow each crewmember the privacy he or she would like; space will be allocated to supplies (particularly food and water) as a matter of priority. Worsening the issue is the fishbowl-like nature of life in space. Not only do members of the ground support team follow the crew’s every action with close attention but so do members of the world media. This will be particularly true of any exploration mission crew bound for the Moon or Mars (Table 114-2).
Circadian Patterns and Sleep Disturbances
Sleep and circadian rhythm disturbances may also contribute to in-flight disorientation. Sleep hygiene is encouraged through limited exposure to light, pharmacologic aids (45% of all medicines used by Space Shuttle crews are sleep aids84), and guidelines that limit sleep-shifting schedule changes.
Analog Environments
Valuable conclusions can be inferred from looking at health data from these analog environments. These populations have prolonged absences from proximity to health care, and have limited resources. The Australian National Antarctic Research Expeditions (ANARE) Register noted 5103 illnesses and 3910 injuries over the 9 years from 1988 to 1997.54 Although most of these individual medical events posed little risk to the crew and mission, there were several of significant severity that needed emergency intervention.
Space Environment
Micrometeoroids And Space Debris
Spacecraft routinely collide with “space dust” of size 1 µm and larger.67 Meteors (also known as meteoroids or micrometeoroids, depending on their size) are small bodies of solid matter that move through space, having originated in the interplanetary region, presumably from crumbling asteroids or comets.99
Most meteors (61%) are made of stone, with a sizable number (35%) composed of iron.67 The vast majority of the micrometeoroid material is <0.1 mm in diameter.69 Although their size is tiny, micrometeorites travel at such high velocities that they have significant kinetic energy. When they enter Earth’s atmosphere, they are heated by the friction of the thickening air around them and may become visible as “shooting stars.” About 10 million kilograms of this interplanetary dust reaches Earth’s surface every day as meteorites or micrometeorites.38
Orbital debris is any human-made object in orbit that no longer serves a useful purpose; a great deal of orbital debris is material that comes from other spacecraft. Some estimates suggest that millions of kilograms of debris are suspended in orbit, of which the most numerous component is “fragmentation debris” consisting of old satellite fragments (e.g., dead batteries, unused fuel cells) and deterioration products such as paint chips and aluminum oxide particles left over from rocket fuel exhaust.68 The remainder is composed of rocket bodies, nonfunctional spacecraft, and mission-related debris.68 Orbital debris can remain in orbit indefinitely, depending on its orbital altitude. Objects at altitudes less than 200 km (124 miles) are likely to fall to Earth within a few days, whereas those above 36,000 km (22,370 miles) will stay in orbit forever.80 Debris at altitudes between 200 and 600 km (124 and 373 miles) will likely stay in orbit several years; debris at altitudes between 600 and 800 km (373 and 497 miles) will stay in orbit several decades; and between 800 and 36,000 km (497 and 22,370), will stay in orbit for centuries. Humanity’s short history of spaceflight to date has already produced a remarkable amount of debris (Figure 114-10).
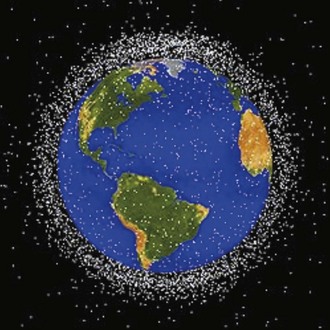
FIGURE 114-10 An artist’s image of Earth showing the currently tracked orbital debris in low Earth orbit.
(From NASA Orbital Debris Program Office. http://www.orbitaldebris.jsc.nasa.gov/photogallery/photogallery.html.)
Collisions with either form of matter (micrometeoroids or orbital debris [MMOD]) take place at very rapid speeds (approximately 10 km/s on average or 16 km/sec for head-on collisions), which releases a great deal of kinetic energy, even for tiny objects such as paint flakes.67 As a result, this material poses a danger to space travelers. For Shuttle missions, the risk of a lost mission increases from 1/107 to 1/76 when MMOD is considered.40 MMOD was termed the “number one loss of crew vehicle risk driver” in a 2003 probabilistic risk assessment (PRA), although the risk and consequence(s) of the MMOD strike vary greatly depending on where on the orbiter the impact occurs.100 In a September 2002 briefing, a senior ISS engineer reported that MMOD was predicted to cause one ISS evacuation every 214 years.40 This was considered the second most likely cause for an ISS evacuation, with a medical emergency (including the sequelae of a radiation event) being the most likely cause (estimated at one evacuation every 5 years). In the same presentation, data were presented assuming a 40% chance of an MMOD-caused hole in the ISS (over a 15-year lifespan of the station), with a 12.8% chance (1 in 8) of the resultant hole necessitating a crew evacuation.40
Approaches to Minimize Micrometeoroids and Space Debris
Very large objects (>10 cm [4 inches] in diameter) will usually be detected in time for the spacecraft to maneuver out of their way, whereas very small ones (<0.1 cm diameter) can be handled by current spacecraft shielding.64 Accordingly, there remains only a small number that are too big to be controlled by shielding, but too tiny to detect before impact. According to the NASA-Johnson Space Center (JSC) Office of Orbital Debris, “debris objects smaller than 0.1 cm generally do not penetrate spacecraft [but] 0.1 to 10 cm debris penetrate and damage spacecraft [whereas] 1 cm debris objects and larger will cause catastrophic failure (for example, loss of functionality of satellite due to impact).”67 The North American Aerospace Defense (NORAD) Command and United States Space Surveillance Network tracks orbital debris >10 cm in diameter so that spacecraft can avoid them; currently approximately 11,000 of these objects are being monitored. Another approximately 100,000 objects are estimated to fall in the 0.1 to 10 cm range and can neither be accurately tracked nor shielded against. The vast majority of orbital debris (numbering in the millions) are <1 cm and are therefore less likely to cause damage to a spacecraft.50
Objects 1 cm in diameter can penetrate typical spacecraft shielding, potentially causing depressurization of a habitation module.67 The speed of such a depressurization depends on the module volume and the size of the hole caused by the debris.
Protection Against Micrometeoroids and Space Debris During EVA
Although spacecraft walls are thick enough to protect astronauts from impacts with most of the tiny material, the risk to crewmembers increases when they leave the shielded spacecraft, as during spacewalk. Spacesuits are designed with a layer of shielding to protect the astronauts to the greatest extent possible, but even with this shielding, particles of 1 mm in diameter may still be able to penetrate the outer protective layer of the spacesuit, increasing risk by an order of magnitude.14,77 Intravehicular astronauts generally only worry about objects larger than 1 cm, but EVA astronauts are at risk from objects larger than 1 mm.
Impact Emergency Procedures
An example of an MMOD impact occurred on STS-94, when an impact crater in one of Columbia’s windows was found after landing.67 The crater was approximately 1 mm diameter, and the MMOD object was estimated to be approximately 100 µm (0.01 cm) in size. Tests indicated that it was aluminum oxide residue from a solid rocket motor. Figure 114-11 shows an MMOD impact from STS-7.
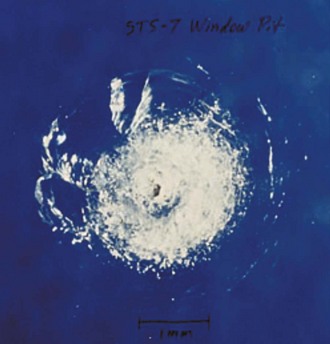
FIGURE 114-11 An MMOD impact on the orbiter window during mission STS-7.
(From NASA Orbital Debris Program Office. http://www.orbitaldebris.jsc.nasa.gov/photogallery/photogallery.html.)
Another example occurred in 1996, when a briefcase-sized piece of an Ariane 1 rocket body collided with a Cerise communication satellite at 14 km/sec (31,500 mph), severing the satellite’s 6-m stabilization boom.73,83
Following the Mir/Progress collision in 1997, when a Spektr module was punctured and lost its pressure, the crew was able to evacuate safely in a controlled fashion because the leak was relatively slow. The Spektr module was sealed off, and although it eventually dropped its internal pressure to vacuum, the rest of the station never dropped below 675 mm Hg (normal is 750 mm Hg).52
Radiation
In 1958, Dr. James Van Allen first described a “belt” of particles, predominantly protons and electrons, trapped in Earth’s magnetic field (Figure 114-12). The proton belts extend to an altitude of approximately 20,000 km (12,428 miles) with peak intensities at 5000 km (3107 miles), and the electron belts extend to 30,000 km (18,642 miles) with peaks at 3000 km (1864 miles) and 15,000 km (9321 miles). Although the magnetic field traps potential sources of radiation exposure, it also acts as a filter for the much more energetic heavy ion radiation of deep space. Galactic cosmic rays are extremely energetic ions ranging from protons to iron nuclei and have the most potential for radiation exposure on deep space missions, such as landing on Mars.
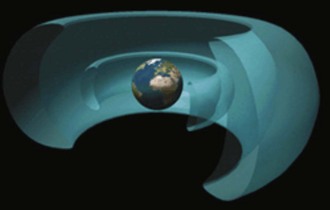
FIGURE 114-12 Artist’s rendition of the Van Allen Belts.
(From What Is Space Radiation? http://srag-nt.jsc.nasa.gov/AboutSRAG/What/What.htm.)
Radiation exposure in LEO comes primarily from the Van Allen belts and from GCRs. Crewmember exposure is measured in Sieverts (Sv), which is a product of radiation energy absorbed (Gray [Gy]) and a dose-equivalent quality factor that takes into account different types of radiation (alpha, beta, gamma rays). The amount of exposure varies with time, altitude, orbital inclination, and radiation shielding. A 1-week Shuttle mission has had exposures ranging from 0.005 to 0.05 Sv, and a 1-year Mir mission resulted in 0.584 Sv dose-equivalent exposure. NASA guidelines have established that the radiation dose limit for blood-forming organs (the most sensitive system to radiation effects) is 0.5 Sv/year. This is estimated to confer a 3% excess cancer mortality over a 10-year career.74
Countermeasures for prolonged radiation exposures are mostly unproven. Genetic screening has been proposed as a strategy for identifying crewmembers with vulnerability for oncogenic mutations.76 Other proposals include prophylactically dosing the crew with radioprotective chemotherapy. Side-effect profiles have limited this as a viable option but may hold promise for the future. Less toxic pharmaceutical interventions may incorporate antioxidants (vitamins A, C, and E; trace amounts of iron, zinc), or tumor growth disruptors, such as dimethylsulfoxide (DMSO) or protease inhibitors, as a way to minimize cell damage.20 Certainly for exploration-class missions, preflight cryogenic gamete preservation would be recommended for all crewmembers of childbearing age, and along the same lines, bone marrow preservation is recommended in case an autologous transplant should be necessary on return.
Shielding is an obvious solution to ambient radiation, but it requires inventive engineering solutions—such as water tanks built into the outer shell or the use of high-density polyethylene. Although the idea of a “storm shelter” (e.g., a location on the vehicle where crew could weather the relatively short-lasting SPEs) has been proposed, GCR exposure is constant, and the entire vessel would need to be shielded. Radiation exposure has an exponentially inverse relationship with protective shielding, meaning multiple layers of shielding confer small decreases in radiation exposure. Several meters of shielding would be required to eliminate GCR exposure—an impractical engineering demand given current technology. One creative solution for a Mars-bound vessel suggests using superconductors to create a synthetic magnetic field29; obviously, the physiologic effects of such a field would require extensive study before implementation. Until a combination of solutions emerge to mitigate the effects of GCR, manned spaceflight will likely be confined to LEO and the relatively short trip to the Moon.
The Moon has no atmosphere and is also exposed to GCR, so lunar soil has long been studied as an abundant source of radiation shielding for prolonged stays (Figure 114-13). Although Mars has an atmosphere that consists mainly of CO2, it is less than 1% as thick as Earth’s, and Mars has no global magnetic field. The Mars Radiation Environment Experiment (MARIE), part of the Mars Odyssey Orbiter, entered Martian orbit in 2001 for the purpose of determining GCR effects on Mars.56 Previous estimates of dose-equivalent GCR exposure on the Martian surface are 0.12 Sv/year (to blood-forming organs).23,91
Operational Concerns
Starting Out With “The Right Stuff:” Planning The Expedition
Crew Selection—Medical Criteria
The initial medical qualification standards for astronauts are significantly higher than the recurrent qualification criteria, as the latter reflect the substantial training investment made in the astronauts over the intervening time frame.62 A 48-year-old active astronaut with medical problems may thus be retained, whereas the same person as an astronaut applicant would be rejected.
It is important to understand the value placed on training and experience when reviewing the medical standards for space flight, as it reflects the stress placed on mission success. If the value added to the mission by a particular crewmember outweighs the medical risk (to both crewmember and mission) associated with his or her health, then he or she is likely to fly (Figure 114-14). In commercial space travel, where participants are passengers and not crewmembers, and mission success may be defined as a safe journey and satisfied customers, the need for training and screening drops dramatically.
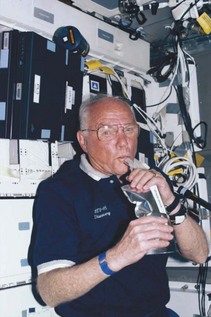
FIGURE 114-14 Astronaut John Glenn on his 1998 mission at age 77.
(From NASA Human Space Flight Gallery. http://spaceflight.nasa.gov/gallery/images/shuttle/sts-95/html/s95e5055.html.)
Review and revision of medical standards are supposed to occur regularly, usually on a 5-year cycle for major changes (lesser changes every 2 to 3 years), but operational demands have been known to delay this review.73,87 Since the days of the Apollo program, standards have been significantly relaxed, and more modern technology, such as endoscopy, ultrasonography, magnetic resonance imaging (MRI), and other imaging modalities, are used extensively in evaluating crew health.
Psychological Factors in Crew Selection
Several authors have suggested that future long-duration missions are more likely to be compromised by psychological reasons than by physical ones.48,78 It is therefore very important to ensure crewmembers are not only physically healthy, but also mentally and emotionally so. In addition, the blend of crewmembers should facilitate interpersonal relationships.
A survey of NASA, CSA (Canadian Space Agency), ESA, and JAXA personnel identified 14 key multicultural factors:43,79
Longer missions will mean increasing leisure time and more unstructured activity, which may affect the cohesiveness of a crew, resulting in the formation of subgroups with “scapegoating.” Lieutenant General Beragovoi, who headed cosmonaut training in the 1980s, reported that at about 30 days into a mission, hostility became evident within a space station crew, although the feelings were displaced to the earthbound ground crew.19 However, prolonged Soviet Salyut/Mir missions of the 1980s and more recent ISS experience have shown that maintenance of good communications and psychosocial support are important in maintaining high work capacity and a happy environment among crews.
Preflight/Mission Planning
Communication and team-building exercises are also staples of preflight training, with crewmembers trained in conflict resolution and stress management. Classroom activities are interspersed with mission simulation in the mock-ups, in the NBL, and during C9B parabolic flights. Land and water survival training courses are also part of the training regimen (Figure 114-15, online). Medical training for all crewmembers consists of the general principles of space medicine and physiology, as well as first aid and cardiopulmonary resuscitation (CPR) courses.
Medical System Design
Over the history of spaceflight there have been many incidents of injury, and 22 mission-associated fatalities (equivalent to roughly 5% of the total flown crew population) (Table 114-3). To date, the fatalities all occurred during the most dangerous phases of flight—launch and landing. Although spaceflight is an endeavor not without risk, with planning and foresight, physicians in the space medicine community can help to minimize the likelihood of illness, injury, and loss of life, and when illness and injury occur, prevent patient deterioration and promote recovery.
TABLE 114-3 Spaceflight Contingencies, Morbidity and Mortality, 1961-2009
Date | Mission | Description |
---|---|---|
3/23/1961 | Soyuz ground test | Cosmonaut Valentin Bondarenko died in a fire in a spacecraft simulator with 100% oxygen environment. |
7/21/1961 | Mercury 4-Liberty Bell 7 | Gus Grissom suborbital flight—nearly drowned when the hatch unexpectedly blew off his capsule after splashdown. |
5/16/1963 | Mercury 9 | Elevated CO2 levels and loss of power to control system, required manual reentry. |
3/18-19/1965 | Voskhod 2 | Manual deorbit, and service module failed to separate during reentry, landed 1200 miles off target. Crew rescued next day. |
3/16/1966 | Gemini 8 | Docked vehicles rotated out of control near structural limits. Crew landed early—waited overnight before ocean recovery. |
6/5/1966 | Gemini 9 | Astronaut’s helmet faceplate continually fogged over during extravehicular activity. |
1/27/1967 | Apollo 1 | Fire in crew module during ground test, with 100% oxygen environment. Crewmembers, Chaffee, Grissom, and White perished. |
4/24/1967 | Soyuz 1 | Parachute system did not deploy after reentry capsule destroyed on impact, resulting in fatality of cosmonaut Komarov. |
11/15/1967 | X-15 | X-15 Flight 191 was a test flight of the North American X-15 experimental aircraft piloted by Michael J. Adams. The aircraft broke apart minutes after launch because of technical difficulties, killing the pilot and destroying the plane. |
1/18/1969 | Soyuz 5 | Spacecraft tumbled during entry, landing 2000 km off target, with hard impact. Cosmonaut suffered non-lethal injuries. |
4/11-17/1970 | Apollo 13 | Mission to Moon aborted after oxygen tank ruptured. Crew returned safely. One crewmember developed urosepsis, in part because of degraded vehicle systems. |
4/23-25/1971 | Soyuz 10 | Failed docking with Salyut 1. During landing, Soyuz air supply became contaminated and cosmonaut became unconscious. |
6/29/1971 | Soyuz 11 | Cabin pressure failure during reentry. Three crewmembers—Dobrovolsky, Volkov, and Patsayev—perished. |
12/1972 | Apollo 17 | Back strain from drilling core sample during walk on lunar surface. |
4/5/1975 | Soyuz 18-A |
Launch vehicle malfunction; second stage abort subjecting crew to nearly 20Gx. Crew landed in Eastern Russia, rescued the next day. Crewmember suffered internal injuries. |
7/24/1975 | Apollo-Soyuz | Apollo crewmembers developed airway reactivity/pneumonitis from toxic contaminants in cabin air during reentry, requiring hospitalization |
8/24/1976 | Soyuz 21 / Salyut 5 | Mission curtailed because of crewmember illness—related to Environmental Control Systems problem |
10/16/1976 | Soyuz 23 | After failure to dock with Salyut 6, capsule landed in blizzard conditions at night onto ice-covered Lake Tengiz; rescue team unable to recover capsule until next morning. |
11/11/1982 | Salyut 7 | Acute abdominal pain—probable kidney stone, resolved on-orbit. |
9/26/1983 | Soyuz T-10A | Launch abort due to pad fire, crew landed safely via capsule escape system. |
6/1985-9/1985 | Soyuz T-13 | Hypothermia and CO2 toxicity during reactivation of Salyut 7. |
11/21/1985 | Salyut 7 | Crewmember became ill with prostatitis and urosepsis. Return to Earth required after 56 days into a 216-day mission. |
1/28/1986 | STS-51L | Solid rocket booster seal failure resulted in Shuttle destruction 73 seconds into flight. All seven crewmembers perished: Gregory B. Jarvis, Christa McCauliffe, Ronald E. McNair, Ellison S. Onizuka, Judith A. Resnik, Francis R. (Dick) Scobee, and Michael J. Smith. |
1987 | Mir 2 | Crewmember developed tachy-dysrhythmia during extravehicular activity—safely returned on next mission of opportunity. |
6/1991 | STS-40 | Freezer motor malfunction caused formaldehyde toxicity and headaches, exacerbated by cabin noise |
1995 | Mir 18 | Crewmember experienced episode of asymptomatic, sustained ventricular tachycardia. No mission impact. |
1996 | Mir 22 | One week preflight, crewmember developed electrocardiogram changes and was disqualified from the mission. |
1995 | Mir 18 | Traumatic eye injury resolved with onboard treatment. |
2/23/1997 | Mir 23 | Fire due to oxygen generator led to smoke and potentially toxic fumes in station. Mild second-degree burns and reactive airway changes among crew. Onboard treatment given. |
1997 | Mir 23 | Three crewmembers experienced upper-airway irritation and dermal reaction following accidental exposure to ethylene glycol. |
6/25/1997 | Mir 23 | Progress resupply vehicle collided with Spektr module during manual docking, resulting in module depressurization. |
2/1998 | Mir 24 | Three crewmembers exposed to elevated carbon monoxide, with headache symptoms. |
2/1/2003 | STS-107 | Space Shuttle Columbia broke apart on reentry, and all crew were lost: Michael Anderson, David Brown, Kalpana Chawla, Laurel Clark, Rick Husband, William McCool, and Ilan Ramon |
Adapted from Space Clinical Medicine, “Medical Evacuation.”
The fundamental mission priorities are (in descending order of importance):
For the ISS medical systems, initial planning decisions favored a “scoop and run” concept, in which a crew return vehicle (CRV) with medical resources would be permanently available and casualties could be evacuated speedily to a DMCF on Earth (Figure 114-16). This allowed minimal on-orbit resources and required only limited medical training for crewmembers. This trade of in situ capabilities for rapid evacuation seemed appropriate, saving as it did up/down mass, stowage volume, and training time. Unfortunately, a change in the mission profile subsequently occurred, caused by changes in program funding, cancellation of the CRV program, extension of mission duration, and temporary decrease in crew size. As a result, neither in situ medical capabilities nor prompt emergency medical evacuation were available to ISS crews, creating a mismatch between required and actual system capabilities.6
Predicting Likely Illnesses And Injuries
An organized approach to risk management follows the principles of primary, secondary and tertiary prevention, with the aim of preventing (or at least minimizing the risk of) a medical situation or, should such an event occur, supporting full recovery of the affected crewmember as quickly as possible.31
Deciding What To Bring (Benefit Vs. Burden)
What do we know of illnesses during spaceflight and what can we expect as we increase the number of human-hours in space? NASA data on the first 89 Shuttle flights from 1981 to 1998 demonstrated that 98% of astronauts reported a medical event or symptom other than SMS during their mission.11
Designing The Vehicle
The equipment packaging itself is more driven by engineering requirements than by usability. The ISS medical checklist is a telephone book–sized document that was initially compiled in alphabetical order to suit engineering protocols, although studies showed that this type of organization was the least successful in guiding medical intervention and successful resuscitation.57
Currently, there is a professional, highly trained, and extremely motivated astronaut corps. However, the current astronauts are, in many cases, less fit than the first Mercury Project test pilots, and it is likely that as more people venture into space, be they tourists or maintenance workers on a moon base (Figure 114-17, online), fitness levels will decrease further. Accordingly, the medical system must be prepared to handle a wider variety of preexisting conditions. This is similar to trends seen terrestrially, as a wider range of people embark on “adventure tourism” and as more contractor personnel are used in military operations overseas.
Training The Crew
During a LEO mission, there is often no physician available, and the astronauts must rely on a fellow crewmember to be their primary caregiver. This system of “buddy care” training is formalized in the role of the crew medical officer (CMO). ISS CMOs receive approximately 40 hours of training on topics such as diagnostics and therapeutics, phlebotomy, suturing, splinting, and endotracheal intubation (Figure 114-18, online). Part of their role is to provide information to the ground-based Flight Surgeon in Mission Control, who has the ultimate responsibility for the health of the crew. Studies using the NEEMO underwater research facility and crews simulating medical intervention for emergencies have shown the limitations of the CMO system.35,36 Medical instructions and protocols using diagrammatic checklists and decision support technologies have also been proposed and/or developed for the ISS, using similar approaches to those used in Earth-based prehospital care.
Treating The Casualties
Issues for Therapeutic Intervention
Pharmacokinetics involves the absorption, distribution, utilization, and metabolism of drugs. Pharmacodynamics involves drug interactions in living systems. Although there is evidence that both vary in the space flight environment (e.g., one study on in-flight use of intramuscular promethazine documented less than 5% experience of sedation, whereas ground-based controls had a sedation rate of more than 70%;10 bioavailability of certain drugs, including scopolamine and acetaminophen, has been shown to be different in-flight), relatively little is known about microgravity-associated changes to these parameters.97 In part, this is due to small sample size and limited opportunities for in-flight observations. Unfortunately, analog environments are largely unsuitable for pharmacologic studies, although some work has been done using bedrest and head-down tilt models. As a result, few data can be gathered from anything other than on-orbit research. Unfortunately, this research is often beset by confounding factors, such as relative hypovolemia, changes in diet and sleeping patterns, SMS, muscle atrophy (which could have effects on drug binding by circulating proteins), and stress levels. Other parameters, some as yet poorly understood, can also play a role; for example, hepatic blood flow may be altered in microgravity, and this would be expected to have a significant impact on first-pass effects and drug excretion.
Crew Medical Officer Training and Pharmaceuticals
Preliminary data suggest that the shelf life for certain pharmaceuticals is reduced on orbit, presumably because of the higher radiation environment.84,97 Supplementary testing of all drugs intended for flight will likely be necessary, particularly for those intended for exploration-class missions. Without this research, it would be impossible for the aerospace physician to know if a patient’s failure to respond to a prescribed drug was due to an erroneous diagnosis, worsening condition, or inactive pharmaceutical. This work may also result in the need for new spacecraft designs, with additional radiation shielding for medication kit(s). Another alternative might be to store drugs in powder form and reconstitute them as needed, because powdered forms tend to have longer shelf lives in the terrestrial environment. However, mixing drugs during space flight poses numerous challenges, from the current inability to create injectable-grade water in-flight, to the increased training requirements for CMOs, to the challenges of creating a homogeneous mixture in microgravity. Research is currently underway in this area, but the technical difficulties are considerable.
In-Flight Support on Long-Duration Missions
Keeping Them Healthy: Creating A Home
The ISS ventilator (AutoVent 2000) also uses 100% oxygen exclusively.42 As a result, medically compromised patients aboard the ISS can receive either 100% oxygen or room air, but there is currently no means of providing supplemental oxygen between 21% and 100%. This may create difficulties in seriously ill or injured crewmembers who require mechanical ventilation or supplemental oxygen for prolonged periods, as studies have shown that deleterious effects from breathing 100% oxygen at sea-level pressures occur in as little as a day. In addition, there are limited oxygen supplies and therefore thought needs to be given as to how best to use a ventilator in space and how to wean a crewmember off the ventilator, assuming of course the CMO is competent to intubate in the first place. The issue here is that it is easier to start care than to sustain it in environments where there are limited supplies and limited options for definitive care. For example, what would happen if a crewmember on the way to Mars required ventilation? At some stage, the supply would expire and a return to definitive care may not be an option.
Air supplies for spacecraft repressurization and crew respiration are usually stored in high-pressure tanks as liquids in cryogenic storage or in other forms.14,16 Nitrogen, for example, can be stored as hydrazine (N2H2), which is also a spacecraft propellant. Four high-pressure gas tanks (two each for nitrogen and oxygen) are located on the exterior of the joint airlock on the ISS, and can be either refilled or replaced by the Shuttle (Figure 114-19).
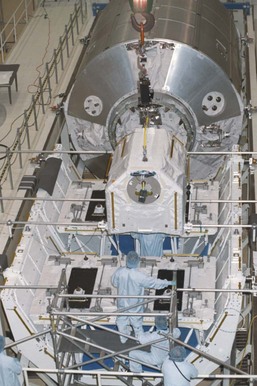
(From NASA Image Exchange. http://nix.larc.nasa.gov/info;jsessionid=1n21fjejgtner?id=KSC-01PP-0952&orgid=5.)
Spacecraft life support systems must monitor several atmospheric parameters, including ambient pressure, partial pressure of each gas (including carbon dioxide), ventilation, temperature, and humidity.82 Whereas the oxygen monitoring system maintains the partial pressure of oxygen relatively close to that experienced on Earth, spacecraft carbon dioxide monitoring systems deal with levels significantly higher than what is seen terrestrially; 7.6 mm Hg of carbon dioxide is the upper limit of normal for both ISS and Shuttle life support (33 times Earth-normal). Current technology does not offer a method to reduce these levels further, and to date, no evidence exists that these elevated levels have long- or short-term effects on space traveler health.
Contaminants
During emergencies (such as a fire), additional hazards from cyanide gas to molten metal particles can be introduced into the atmosphere. Removal of many of these substances can be achieved through use of filters (Figure 114-20). However, there are some contaminants (e.g., toxic by-products of the Halon used as a fire suppressant on the Shuttle) that current filters cannot remove.
Temperature
The life support system of a spacecraft must protect its occupants not only from the intense cold outside but also from buildup of heat within. The multiple sources of heat within the spacecraft that can affect thermal control are: electronics, lighting, solar heating, and metabolic heat (as produced by exercise). Equipment produces high thermal loads. In most cases, hyperthermia is more of a danger to space travelers than is hypothermia. The literature clearly documents decrements in task performance as crew comfort declines. Long-duration Soviet/Russian spaceflights suggest that ideal temperatures for a working environment are 22° to 24° C (72° to 75° F). When temperatures drop below 19° C (66° F), with a relative humidity around 70%, the crews complain of cold. An unusual example of contending with cold occurred on the damaged Apollo 13 spacecraft, which required strict conservation of energy on the return to Earth. The uncomfortable crew was very glad to get home (Figure 114-21).
Humidity
Circulation of cabin air is the mainstay of temperature and humidity control. As air is recirculated, moisture can be removed from it either by a desiccant (as is usually the case in spacesuits) or through condensation of the vapor and removal of water from the gaseous air. This latter method is used more often in spacecraft, because it allows reuse of the water thus produced. In general terms, condensation occurs when cabin air is cooled on heat exchangers, and the resultant water is then removed by separators (either via capillary action or hydrophobic–hydrophilic surfaces). A description of the life support system for the ISS is shown in Figure 114-22.
Waste Management
Generally speaking, waste collection is designed to be as simple as possible for the crew. In some cases, crewmembers merely place the refuse in a container and stow it. In other cases, the waste may be automatically collected and stored, as is the case for the toilet appliance. In all cases, however, limited stowage space remains an issue that drives waste disposal policy. For waste material that the crew should not handle for reasons of health or safety, the appropriate systems usually collect and package the waste automatically. Virtually all forms of waste disposal currently make use of Earth, either by returning trash to the ground for final disposal or by utilizing atmospheric reentry to destroy trash in the Progress rockets. Unfortunately, these options are not ideal for long-duration exploration missions, when Earth will not be available. In these situations, waste will need to be reused or destroyed in more efficient ways, so as not to take up valuable storage space.60,93
Biologic solid wastes, such as those from food, generally contain 40% to 90% moisture and soluble organic compounds.44 As a result, these wastes cannot be stored for extended periods, because they decompose (leading to growth of undesirable anaerobic microorganisms), produce noxious gases (including N2O, NH3, H2S), and create foul odors.
Even missions to orbit risk exposure to outside contaminants, as was seen during STS-98, when an EVA crewmember became covered in ammonia crystals during connection of the U.S. Lab module to the rest of the ISS30 (Figure 114-23).
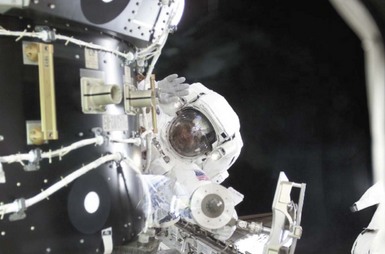
FIGURE 114-23 During an extravehicular activity on STS-98, astronaut Bob Curbeam was exposed to ammonia.
(From http://spaceflight.nasa.gov/gallery/images/shuttle/sts-98/lores/s98e5190.jpg.)
Medical Waste
There is also the question of what to do with medical samples. The current ISS blood analysis device, the i-STAT Portable Clinical Blood Analyzer,42 only uses a few drops of blood, which are wicked into a self-contained cartridge. However, if longer-duration missions have expanded laboratory facilities, additional attention to the question of discarded samples will be needed.
Crews also use urine collection devices during EVAs. Male astronauts wear a pouch-like device similar to a condom catheter, and women wear “disposable absorption and containment trunks,” a pair of multilayered shorts that contain an absorptive powder.1,51,61 Each device has a storage capacity of roughly a liter of fluid. Crew also wear urine collection garments (like adult diapers) underneath their launch and entry suits, because the Shuttle toilet is unavailable for use during those phases of flight (and even if it were available, the crew cannot easily doff the ACES suits).
To handle feces, the toilet has a cylindric container in which a plastic bag is placed before use. Crewmembers use foot restraints and bars positioned over the thighs to hold themselves on the toilet seat, ensuring a good seal with the contoured, soft seat. The good seal is necessary to ensure that the toilet’s airflow will be able to draw the waste away.33 The hole for solid wastes is only 10 cm (4 inches) in diameter, and crewmembers actually practice positioning themselves on a toilet trainer before flight. A camera placed within the toilet trainer, directly beneath the hole for solid waste, allows them to see if they are positioning themselves correctly. A video showing proper use of the Shuttle toilet can be found at http://edspace.nasa.gov/text/livespace/gottago.html.
Feces enter the commode through the seat opening and are drawn in by air flowing through holes under the seat. This downrushing airflow (850 L/min) substitutes for gravity in collecting and keeping the waste material inside the commode.33 The wastes are then broken up by rotating vanes and deposited along the walls in a thin layer. A hydrophobic liner inside the commode prevents free liquid and bacteria from leaving the collector. The plastic bag is then sealed, and a plunger attached to a lever forces it to the bottom of the cylinder. A new bag is then placed in the toilet for the next astronaut. When the cylinder is filled, it is replaced by a new cylinder. Figure 114-24 shows the design.
Another question (that has largely been avoided to date) is what to do in the event of a death on orbit. Leaving aside the questions of how death would be ascertained and declared, what effects such an event would have on the other crewmembers, the impact on the mission, and other such matters, there still exists the issue of what to do with the remains. Although returning the remains to Earth might be feasible, albeit unpleasant, on the Shuttle, the tight quarters of the Soyuz make it much more difficult to transport a body. And what about exploration missions? Even if a crewmember’s death were to force abandonment of the mission, the time required to return to Earth might be too long to permit transport of an unpreserved cadaver. Procedures to handle a death on an exploration-class mission remain under discussion.8,9
What To Wear?
In the early days of the space program, astronauts wore pressure suits derived from X-15 suits during virtually the entire (short) mission. The Mercury “silver foil” spacesuit was a custom-fitted, modified version of the Goodrich U.S. Navy Mark IV high-altitude jet aircraft pressure suit (Figure 114-25, online, shows Mercury program boots, helmet, and gloves). By contrast, current spacecraft have a “shirtsleeve” cabin environment; in other words, no special gear or equipment is needed to live comfortably in the environment.71 However, protective suits are worn during launch and reentry phases of flight. Although the cabin environment at these times remains unchanged from the “shirtsleeve” parameters, protective suits are worn because of the dangers inherent in these periods of flight. The suits differ somewhat between the Shuttle and Soyuz spacecraft, but both are designed to protect the crew in the event of a problem during take-off or landing.
One unique item of apparel used to counter physiologic adaptation to space is the Russian “penguin suit.”72 Designed as a countermeasure for muscle atrophy associated with prolonged exposure to microgravity, the penguin suit is a snug-fitting, full-length, long-sleeved jumpsuit that zips vertically to the crotch, with horizontal zip pockets on either side of the chest and just below the waist. The suit is made of a synthetic fabric with elastic inserts at the collar, waist, wrists, and ankles and along the vertical sides of the suit. The inside of the suit contains a system of elastic straps and buckles that are used to adjust fit and tension of the suit. When astronauts and cosmonauts tighten tension on these bungee-like bands, the suit can exert resistance, opposing the astronauts’ movements and simulating some of the effects of gravity (see Figure 114-7, online).
Another Russian countermeasure suit makes use of lower body negative pressure and resultant redistribution of blood volume to combat postflight orthostatic hypotension.25 This suit was employed on Soviet and Russian space stations, but is not in use on the ISS. The ISS is of course a shirtsleeve environment, and any long-duration exploration-class mission is likely to be the same.
While they are in the EMU, crewmembers wear a liquid cooling (and ventilation) garment (LCG). The LCG contains a network of vinyl tubing that circulates cool water and air in order to maintain core body temperature at normal levels.1,61 In conjunction with a thermal undergarment, it keeps an astronaut relatively comfortable while he or she is outside the vehicle and exposed to wildly varying temperatures (about −100° C to +120° C [about −150° F to +250° F]), depending on whether he or she is in sunlight or in shadow). The LCG that is worn under the launch and entry ACES suit only circulates water for cooling purposes. The LCG that is worn under the EMU also circulates air in order to scrub out the CO2 (Figure 114-26, online) (http://www.nasa.gov/audience/forkids/home/CS_Astronaut_Fashion_Show.html).
The current EMU is designed for use in orbit only. The spacesuits used by Apollo astronauts who walked on the Moon were different, in part because of the different technology in those days, but also because the suits were subject to different strains, from Moon dust to lunar gravity. Crews on planetary exploration missions in the future will need new spacesuits, some of which are currently being designed and tested (Figure 114-27).
Personal Hygiene
With water a potentially mission-limiting consumable, personal hygiene in space is different from that on Earth. Water pressure on the ISS, for example, is about half that in a U.S. household. However, for morale purposes as well as basic hygiene concerns, washing, shaving, and toothcleaning must be available for the crews, particularly on long-duration missions. Although both Mir and Skylab had showers on board (Figure 114-28), none of the current spacecraft has one; instead, sponge baths using wet washcloths are the norm. This reduces the quantity of water required from 50 L for the average terrestrial shower to 4 L for the average ISS “bath.”37 Handwashing in space uses only about 10% of the water used for handwashing on Earth.48,55
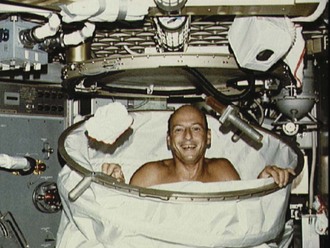
FIGURE 114-28 Astronaut Pete Conrad makes use of the Skylab shower.
(From http://aerospacescholars.jsc.nasa.gov/HAS/cirr/em/11/11.cfm.)
Astronauts wash their hair with a rinseless shampoo originally designed for bedridden hospital patients. Crewmembers first wet their hair with water, then apply the rinseless shampoo (Figure 115-29, online). The hair is scrubbed carefully to avoid flinging water drops throughout the cabin, then dried with a towel. Blow drying is not an option on orbit—crewmembers must wait for their hair to air dry. Generally, the cabin humidity is held around 60%, which allows relatively rapid drying, although it does put additional strain on the humidity control system. A video of Pam Melroy washing her hair in space is available at http://spaceflight.nasa.gov/living/spacehygiene/hygiene_1.html.
“Marching On Their Stomachs”
Food is a key part of crew morale and performance. Napoleon recognized that “an Army travels on its stomach.” The U.S. Navy has a great deal of experience showing how lack of variety in a menu or poorly prepared food can negatively impact a mission. Indeed, in addition to decrements in job performance, increased interpersonal conflicts have been reported when palatable foods are in short supply.24,64,70,85
One major problem with consumption of processed foods on orbit is that they tend to be high in sodium. One study on the Shuttle documented dietary sodium intakes higher than 4000 mg per day.64,70 Terrestrial research has demonstrated that excessive sodium increases bone turnover and urinary calcium excretion, which are already abnormal in space because of the microgravity environment. There is thus concern that the processed foods may contribute to (or worsen) bone demineralization and increase renal stone risk during flight.
Systems for water storage in microgravity environments are significantly more complex than are terrestrial systems. Normally, gravity ensures that water stays in one place, separates from air, and moves down an incline. None of these things occurs in microgravity, necessitating enclosed containers, pressurized lines, and other methods for air–fluid separation. In microgravity, it is much easier to force water out of flexible-walled containers, because they can be squeezed or rolled, much like toothpaste tubes on Earth.13 Unfortunately, these containers tend to have shorter life spans than do their rigid counterparts. Some systems make use of both; in the Soyuz, for example, a rigid-walled tank holds a flexible bladder containing the water, and mouth suction is used to draw water out as required.
Water is routinely delivered to the ISS via the U.S. Shuttle (often in 90-lb, duffle bag–like water containers) and Russian “Progress” rockets (in large tanks), but doing so is expensive and creates dependency on ground supplies. With the addition of Node 3, and the new Water Recovery System, the ISS now has a degree of autonomy13 (Figure 114-30, online). It was in part this dependence on Shuttle-delivered water that forced a decrease in ISS crew size following the grounding of the Shuttle fleet in February 2003 after the Columbia disaster. In the absence of regular visits from the Shuttle to replenish water supplies, a three-person crew could not be sustained aboard the ISS, and the crew complement dropped temporarily to two during the 2003-2005 timeframe in ISS Expeditions 7 through 11.
In-Flight Exercise Countermeasures Programs
As early as the 1960s, Busby identified “periodic physical exercise” as a means for “maintaining an optimum level of physical ‘fitness’ during space missions.”17 In the early days of the space program, vehicle dimensions did not permit much physical exercise. Since the Skylab program, in-flight exercise has been used as a way not only to have fun (as numerous crew photos attest), but also to monitor physical condition and to prepare for various tasks, such as an EVA.
One of the systems most affected by prolonged exposure to microgravity is the musculoskeletal, because lack of a gravitational field causes muscle atrophy, bone demineralization, diminished exercise tolerance, loss of strength, increased risk of kidney stones, osteoporosis, and increased fracture risk. Even during a mission to LEO, musculoskeletal changes can prove dangerous, such as excessive fatigue leading to premature end of an EVA. Exploration-class missions could be seriously compromised if crewmembers become so weakened by their time in microgravity that they are unable to carry out physically challenging tasks on arrival. An expert panel voiced concern that “an abrupt return to gravity whether back on Earth or at the destination of an exploration mission imposes high workloads on weakened muscles and may cause post-space travel pathologies. Adaptation to the lower workload in microgravity may render muscle tissue more prone to structural failure when it is reloaded.”11
The value of strength training versus aerobic conditioning for astronaut health, performance, and postflight recuperation has been much debated, but there is little solid evidence to determine which (if either) is the best countermeasure. Similarly, there are few data to identify the preflight training regimen that will best prepare astronauts for long-duration space flight and their eventual return to Earth’s gravitational field. As a result, both strength and aerobic exercises are currently performed by long-duration crewmembers before and during their sojourn on the ISS. Usually 30 minutes of each 90-minute training session are devoted to aerobic conditioning, with the remaining 60 minutes devoted to resistive exercise.94 The RED allows numerous muscle groups to be exercised, including the back, quadriceps, and arms (Figure 114-31, online).
Cycle ergometry use has been associated with improvements in cardiovascular deconditioning, but it does little to affect skeletal muscle atrophy. Exercise on the treadmill (Figure 114-32), by contrast, may be able to improve cardiovascular parameters and prevent a common problem of foot-drop positioning with associated extensor range shortening. It can also minimize skeletal deconditioning through its associated axial load and heel strike. When used in conjunction with visual stimulation (e.g., watching a DVD while running on the treadmill), it may also enhance neurovestibular readaptation. However, additional controlled studies are required before any particular form of exercise can be clearly labeled “best.”
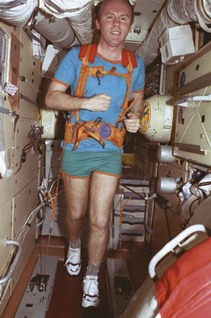
FIGURE 114-32 Astronaut Andy Thomas uses a treadmill aboard the Russian Mir Station.
(From http://spaceflight.nasa.gov/gallery/images/shuttle/sts-91/hires/91375011.jpg.)
Cycle ergometers (Figure 114-33, online) proved popular, not only because bicycling is a familiar terrestrial activity but also because with a simple substitution of hand grips for pedals, the same device can be used for upper-limb training as well as lower-limb. This is particularly useful for EVA crewmembers, as the majority of the work done during spacewalks is performed by the upper body.
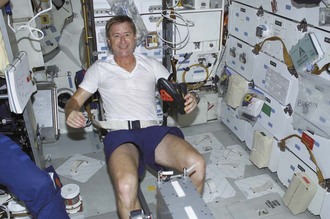
FIGURE 114-33 Astronaut Frank Culbertson on the Shuttle middeck works out on the bicycle ergometer.
(From http://spaceflight.nasa.gov/gallery/images/shuttle/sts-105/hires/s105e5004.jpg.)
Various types of treadmill devices have been flown over the years, from unpowered ones with a Teflon-like surface that allowed easy sliding (Figure 114-34), to powered ones such as the current ISS model. In all cases, a restraint harness is required to hold the astronaut down against the treadmill surface.
On the ISS, devices recently added for strength and aerobic exercise include the new Combined Operational Load Bearing External Resistance Treadmill (COLBERT) which is placed inside Tranquility Node 3 (see http://www.nasa.gov/images/content/177228main_Mobility6.jpg).
Psychological Support
On long-duration missions, psychological support becomes critical to mission success. This takes many forms, from support of the family that has been left behind to avoidance of overwork and fatigue (Figure 114-35).
As a result, the international space medical community has developed rules governing crew rest. NASA flight surgeons attempt to prevent fatigued crews from performing dangerous tasks. Although lack of normal circadian cues in space makes it more challenging to determine when crews are at their best (or worst), it is clear that crews require a few days to adjust to a new sleep schedule before they function at peak performance (Figure 114-36, online).
“Are We There Yet? I’m Bored!” Personal Recreation
For years, military leaders have been aware of the importance of providing adequate rest and recreation time to their subordinates, lest team morale and performance drastically decrease. In many wilderness expeditions, the surroundings and the activity involved in getting to the wilderness areas provide all the recreation one can desire. In space, however, although the views can be breathtaking (Figure 114-37), the sheer duration of the missions can lead to boredom.
Postflight Rehabilitation
The “continuum of preventive, therapeutic, and rehabilitative care on the ground, during space travel, and upon the return from space travel” described by the Institute of Medicine as required by astronauts,11 particularly those returning from long-duration missions, has worrying implications for exploration programs. If ISS astronauts, after flights of several months, require the close attention and rehabilitation currently provided to them, how will any Mars-bound astronauts be able to function on reaching their destination?