CHAPTER 6 Smooth muscle and the cardiovascular and lymphatic systems
The cardiovascular system carries blood from the heart to all parts of the body through a series of tubes, all but the smallest of which are muscular. The muscle in these tubes is of two types: smooth muscle is characteristic of the walls of blood vessels and cardiac muscle provides the walls of the heart chambers with their powerful contractile pumping action. The general characteristics and classification of muscle tissues are given on page 103. Smooth muscle also forms an important contractile element in the walls of many other organ systems of the body, e.g. the gastrointestinal tract.
SMOOTH MUSCLE
Smooth muscle cells (fibres) are smaller than those of striated muscle. Their length can range from 15 μm in small blood vessels to 200 μm, and even to 500 μm or more in the uterus during pregnancy. The cells are spindle-shaped, tapering towards the ends from a central diameter of 3–8 μm (Fig. 6.1). The nucleus is single, located at the midpoint, and often twisted into a corkscrew shape by the contraction of the cell. Smooth muscle cells aggregate with their long axes parallel and staggered longitudinally, so that the wide central portion of one cell lies next to the tapered end of another. Such an arrangement achieves both close packing and a more efficient transfer of force from cell to cell. In transverse section, smooth muscle is seen as an array of circular or slightly polygonal profiles of very varied size, and nuclei are present only in the centres of the largest profiles (Fig. 6.2). This appearance contrasts markedly with that of skeletal muscle cells, which show a consistent diameter in cross-section and peripherally placed nuclei throughout their length.
Smooth muscle has no attachment structures equivalent to the fasciae, tendons and aponeuroses associated with skeletal muscle. There is a special arrangement for transmitting force from cell to cell and, where necessary, to other soft tissue structures. Cells are separated by a gap of 40–80 nm. Each cell is covered almost entirely by a prominent basal lamina which merges with a reticular layer consisting of a network of fine elastin, reticular fibres (collagen type III) and type I collagen fibres (Fig. 6.3). These elements bridge the gaps between adjacent cells and provide mechanical continuity throughout the fascicle. The cell attaches to components of this extracellular matrix at dense plaques (see Fig. 6.4A) where the basal lamina is thickened; cell–cell attachment occurs at intermediate junctions or desmosomes, formed of two adjacent dense plaques. At the boundaries of fascicles, the connective tissue fibres become interwoven with those of interfascicular septa, so that the contraction of different fascicles is communicated throughout the tissue and to neighbouring structures. The components of the reticular network, the ground substance and collagen and elastic fibres, are synthesized by the smooth muscle cells themselves, not by fibroblasts or other connective tissue cells, which are rarely found within fasciculi.
Discontinuities occur in the basal lamina between adjacent cells, and here the cell membranes approach to 2–4 nm of one another to form a gap junction (Fig. 6.2). These junctions are believed to be structurally similar to their counterparts in cardiac muscle. They provide a low-resistance pathway through which electrical excitation can pass between cells, producing a coordinated wave of contraction. The incidence of gap junctions varies with the anatomical site of the tissue: they appear to be more abundant in the type of smooth muscle which generates rhythmic (phasic) activity.
MICROSTRUCTURE OF SMOOTH MUSCLE AND THE CONTRACTILE MECHANISM
Although electron microscopy revealed the presence of filaments in smooth muscle some years ago, this observation alone provided little insight into their mode of function because of the lack of any obvious organization of the filaments. More recent work, using high-resolution immunocytochemistry, has revealed further details of the internal architecture of the cell and suggests a structural basis for contractile function. The model, which is illustrated in Fig. 6.3, depends on the mutual interaction of two systems of filaments, one forming the cytoskeleton and the other the contractile apparatus.
Excluding the perinuclear region, the cytoplasm of a smooth muscle cell effectively consists of two structural domains. The cytoskeleton forms a structural framework that maintains the spindle-like form of the cell and provides an internal scaffold with which other elements can interact. Its major structural component is the intermediate filament desmin, with the addition of vimentin (which may also be present alone) in vascular smooth muscle. The intermediate filaments are arranged mainly in longitudinal bundles, but some filaments interconnect the bundles with each other and with the sarcolemma to form a three-dimensional network. The bundles of intermediate filaments insert into focal, electron-dense bodies, approximately 0.1 μm in diameter, which are distributed uniformly throughout the cytoplasm and also attach to dense plaques underlying the plasma membrane (Fig. 6.3A). The cytoplasmic dense bodies and submembraneous dense plaques are equivalent to the Z-discs of striated muscle cells. They contain the actin-binding protein α-actinin and thus also anchor the actin filaments of the contractile apparatus. These form a lattice of obliquely arranged bundles throughout the cytoplasm, which transmit force to the plasma membrane and thus the basal lamina and extracellular matrix via dense plaques. These are associated with a highly structured arrangement of ancillary proteins, including vinculin and talin, which in turn attach to integrins that cross the membrane and provide attachment to components of the extracellular matrix. An analogous arrangement underlies cell–cell attachment at desmosomes, but here the attachment between dense plaques is provided by transmembrane cadherin glycoproteins and intracellular catenins instead of integrins and talin. Mechanical deformation of the cell may be linked to cell signalling mechanisms via focal adhesion kinase (FAK) and its substrate paxillin; phosphorylation of talin and paxillin may modulate the deformability of the smooth muscle cell. Other regulatory proteins also associate specifically with actin, such as caldesmon and calponin. The cytoskeleton is therefore not a passive structure, and can adapt dynamically to load, presumably therefore contributing to the low energy requirements of smooth muscle contraction. The ratio of actin to myosin is about eight times greater in smooth compared to striated muscle, reflecting the greater length of actin filaments in smooth muscle.
Smooth muscle myosin filaments are 1.5–2 μm long, somewhat longer than those of striated muscle. Although smooth muscle cells contain less myosin, the longer filaments are capable of generating considerable force. The myosin filaments of smooth muscle are also assembled differently, such that their head regions lie symmetrically on either side of a ribbon-like filament, rather than imposing a bipolar organization on the filament. Actin filaments, to which they bind, can thus slide along the whole length of the myosin filament during contraction. This difference underpins the ability of smooth muscle to undergo much greater changes in length than striated muscle. Actin–myosin filament sliding generates tension which transmits to focal regions of the plasma membrane, changing the cell to a shorter, more rounded shape (Fig. 6.3B) and often deforming the nucleus to a corkscrew-like profile.
Caveolae, cup-like invaginations of the plasma membrane with a resemblance to endocytotic vesicles, are a characteristic feature of smooth muscle cells, and may form up to 30% of the membrane (Fig. 6.4). They are associated with many receptors, ion channels and kinases, and the peripheral sarcoplasmic reticulum and may thus act as sites for highly localized signalling pathways. They may also act as specialized pinocytotic structures involved in fluid and electrolyte transport into the cell. Other organelles (mitochondria, ribosomes etc) are largely confined to the filament-free perinuclear cytoplasm, although in some smooth muscle types, including vascular smooth muscle, peripheral mitochondria, sarcoplasmic reticulum and sarcolemma seem to form signalling microdomains. Recent studies using mitochondria-specific staining of such smooth muscle cells often show mitochondria forming a reticular network within the cytosol, which would be consistent with a cell signalling function, especially that concerned with intracellular calcium homeostasis.
INNERVATION
Smooth muscles are innervated by unmyelinated axons whose cell bodies are located in autonomic ganglia, either in the sympathetic chain or, in the case of parasympathetic fibres, closer to the point of innervation (Fig. 6.5). They ramify extensively, spreading over a large area of the muscle and sending branches into the muscle fasciculi. The terminal portion of each axonal branch is beaded, and consists of expanded portions, varicosities, packed with vesicles and mitochondria, separated by thin, intervaricose portions. Each varicosity is regarded as a transmitter release site, and, in the functional sense, is therefore a nerve ending. In this way the axonal arborization of a single autonomic neurone bears a very large number of nerve endings (up to tens of thousands), as opposed to a maximum of a few hundred in somatic motor neurones. The neuromuscular terminals of autonomic efferents are considered in more detail on page 62.
The neuromuscular junctions in smooth muscles do not show the consistent appearance seen in skeletal muscles. The neurotransmitter diffuses across a gap that can vary from 10 to 100 nm: even separations up to 1 μm may still allow neuromuscular transmission to take place, although more slowly. The nerve ending is packed with vesicles, but the adjacent area of the muscle cell is not structurally differentiated from that of non-junctional regions – there is no distinct synapse.
EXCITATION–CONTRACTION COUPLING IN SMOOTH MUSCLE
The regulation of contraction of smooth muscle is however largely calcium-dependent. In the cytoplasm, calcium binds to calmodulin. The complex so formed regulates the activity of myosin light chain kinase, which phosphorylates myosin regulatory light chains and initiates the myosin-actin ATPase cycle (p. 111). The enzymatic activation process is therefore inherently slow. Unphosphorylated myosin II of smooth muscle cannot initiate actin binding, although it can maintain contraction, with little energy expenditure. Myosin phosphatase dephosphorylates myosin light chains, and thus promotes relaxation. Inhibition of the phosphatase, for example by Rho kinase, increases phosphorylation for any level of calcium (i.e. increases calcium sensitivity). This is now believed to be a very important component of the response to many constrictor agonists.
DEVELOPMENT
It was thought that all smooth muscle cells developed in situ exclusively from the splanchnopleuric mesenchyme in the walls of the anlagen of the viscera and around the endothelium of blood vessels. However, recent experimental studies have traced the progeny of cells proliferating from the epithelial plate of the somite and have identified endothelial and tunica media smooth muscle cells arising from individual somites (Scaal & Christ 2004). The origin of the smooth muscle of the iris is still unclear. This region of the eye develops from the optic cup, and so the smooth muscle which arises there is derived either from the neurectoderm of the original optic cup or from the neural crest mesenchyme which later invades the iris.
THE CARDIOVASCULAR AND LYMPHATIC SYSTEMS
GENERAL ORGANIZATION
From the centre to the periphery, the vascular tree shows three main modifications. The arteries increase in number by repeated bifurcation and by sending out side branches, in both the systemic and the pulmonary circulation. For example, the aorta, which carries blood from the heart to the systemic circulation, gives rise to about 4 × 106 arterioles and four times as many capillaries. The arteries also decrease in diameter, although not to the same extent as their increase in number, so that a hypothetical cross-section of all the vessels at a given distance will increase in total area with increasing distance from the heart. At its emergence from the heart, the aorta of an adult man has an outer diameter of approximately 30 mm (cross-sectional area of nearly 7 cm2). The diameter decreases along the arterial tree until it is as little as 10 μm in arterioles (each with a cross-sectional area of about 80 μm2). However, given the enormous number of arterioles, the total crosssectional area at this level is approximately 150 cm2, more than 200 times that of the aorta. As a result, blood flow is faster near the heart than at the periphery.
The development of blood vessels is described on pages, 206–208.
General features of vessel walls
Blood vessels, irrespective of size, and with the exception of capillaries and venules, have walls consisting of three concentric layers (tunicae) (see Fig. 6.7). The intima (tunica intima), is the innermost layer. Its main component, the endothelium, lines the entire vascular tree, including the heart, and the lymphatic vessels. The media (tunica media) is made of muscle tissue, elastic fibres and collagen. While it is by far the thickest layer in arteries, the media is absent in capillaries and is comparatively thin in veins. The adventitia (tunica adventitia) is the outer coat of the vessel, and consists of connective tissue, nerves and vessel capillaries (vasa vasorum). It links the vessels to the surrounding tissues. Vessels differ in the relative thicknesses and detailed compositions of their layers and, in the smallest vessels, the number of layers represented.
Large elastic arteries
The intima is made of an endothelium, resting on a basal lamina, and a subendothelial connective tissue layer. The endothelial cells are flat, elongated and polygonal in outline, with their long axes parallel to the direction of blood flow (see Fig. 6.17). The subendothelial layer is well developed, contains elastic fibres and type I collagen fibrils, fibroblasts and small, smooth muscle-like myointimal cells. The latter accumulate lipid with age and in an extreme form, this feature contributes to atherosclerotic changes in the intima. Thickening of the intima progresses with age and is more marked in the distal than in the proximal segment of the aorta.
A prominent internal elastic lamina, sometimes split, lies between intima and media. This lamina is smooth, measures about 1 μm in thickness, and, with the elastic lamellae of the media, is stretched under the effect of systolic pressure, recoiling elastically in diastole. Elastic arteries thus have the effect of sustaining blood flow despite the pulsatile cardiac output. They also smooth out the cyclical pressure wave. The media has a markedly layered structure, in which fenestrated layers of elastin (elastic lamellae) alternate with interlamellar smooth muscle cells (Fig. 6.6), collagen and fine elastic fibres. The arrangement is very regular, such that each elastic lamella and adjacent interlamellar zone is regarded as a ‘lamellar unit’ of the media. In the human aorta there are approximately 52 lamellar units, measuring about 11 μm in thickness. Number and thickness of lamellar units increases during postnatal development, from 40 at birth.
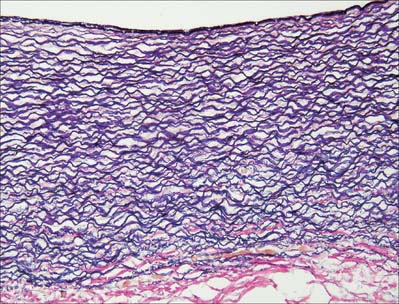
Fig. 6.6 Elastic artery (human aorta), stained for elastic fibres. The dense staining of the internal elastic lamina is seen close to the luminal surface (top); elastic lamellae fill the tunica media and merge with the external elastic lamina at its junction with the collagenous adventitia (red fibres, below). Compare with Fig. 6.20. Elastic van Gieson technique.
Muscular arteries
Muscular arteries are characterized by the predominance of smooth muscle in the media (Fig. 6.8). The intima consists of an endothelium, similar to that of elastic arteries, which rests on a basal lamina and subendothelial connective tissue. The internal elastic lamina (Fig. 6.7, Fig. 6.8) is a distinct, thin layer, sometimes duplicated and occasionally absent. It is thrown into wavy folds as a result of contraction of smooth muscle in the media. Some 75% of the mass of the media consists of smooth muscle cells which run spirally or circumferentially around the vessel wall. The relative amount of extracellular matrix is therefore less than in large arteries, however, fine elastic fibres which run mainly parallel to the muscle cells are present. An external elastic lamina, composed of sheets of elastic fibres, forms a less compact layer than the internal lamina, and separates the media from the adventitia in the larger muscular arteries. The adventitia is made of fibroelastic connective tissue, and can be as thick as the media in the smaller arteries. The inner part of the adventitia contains more elastic than collagen fibres.
Arterioles
In arterioles (Fig. 6.9, Fig. 6.10) the endothelial cells are smaller than in large arteries, but their nuclear region is thicker and often projects markedly into the lumen. The nuclei are elongated and orientated parallel to the vessel length, as is the long axis of the cell. The basal surface of the endothelium contacts a basal lamina, but an internal elastic lamina is either absent or is highly fenestrated and traversed by the cytoplasmic processes of muscle cells or endothelial cells.
Capillaries
The capillary wall (Fig. 6.11) is formed by an endothelium and its basal lamina, plus a few isolated pericytes. Capillaries are the vessels closest to the tissue they supply and their wall is a minimal barrier between blood and the surrounding tissues. Capillary structure varies in different locations. Capillaries measure 4–8 μm in diameter (much more in the case of sinusoids) and are hundreds of microns long. Their lumen is just large enough to admit the passage of single blood cells, usually with considerable deformation. However, the true bottleneck of the circulatory system occurs at the level of the arterioles, where muscle contraction can obliterate the lumen.
Typically a single endothelial cell forms the wall of a capillary, so that the junctional complex occurs between extensions of the same cell. The endothelial cells of some capillaries have fenestrations, or pores, through their cytoplasm. Fenestrations are approximately circular, 50–100 nm in diameter, and at their edge the luminal and abluminal membranes of the endothelial cell come into contact with each other. The fenestration itself is usually occupied by a thin electron-dense diaphragm of unknown molecular composition. Fenestrated capillaries occur in renal glomeruli, where they lack a diaphragm, in intestinal mucosae, and in endocrine and exocrine glands. Fenestrations are almost invariably present in capillaries which lie close to an epithelium, including the skin.
Sinusoids
Sinusoids are expanded capillaries (Fig. 6.12), and are large and irregular in shape. They have true discontinuities in their walls, allowing intimate contact between blood and the parenchyma. The discontinuities are formed by gaps between endothelial cells, which are also fenestrated, such that the sinusoidal lining, and sometimes also the basal lamina, is incomplete. Sinusoids occur in large numbers in the liver (where a basal lamina is completely absent), spleen, bone marrow, adenohypophysis (Fig. 6.12) and suprarenal medulla.
Venules
When two or more capillaries converge, the resulting vessel is larger (10–30 μm) and is known as a venule (postcapillary venule). Venules (Fig. 6.9) are essentially tubes of flat, oval or polygonal endothelial cells surrounded by basal lamina and, in the larger vessels, by a delicate adventitia of a few fibroblasts and collagen fibres mainly running longitudinally. Pericytes (see Fig. 6.21) support the walls of these venules.
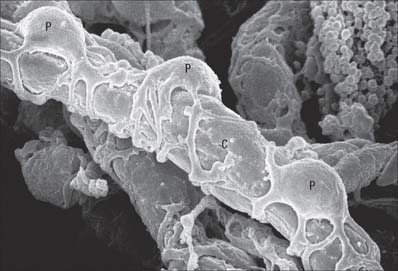
Fig. 6.21 Scanning electron micrograph of capillary (C) and pericytes (P) supporting the vessel wall.
(By courtesy of T. Fujiwara and Y. Uehara, Department of Anatomy, Ehime University School of Medicine, Japan.)
Postcapillary venules are sites of leukocyte migration. In venules of mucosa-associated lymphoid tissue (MALT), particularly of the gut and bronchi, and in the lymph nodes and thymus, endothelial cells are taller and have intercellular junctions through which lymphocytes and other blood components can readily pass. These are known as high endothelial venules (HEV) (see Figs 6.18, 6.19). Elsewhere, venules are believed to be a major site where migration of neutrophils, macrophages and other leukocytes into extravascular spaces occurs, and where neutrophils may temporarily attach, forming marginated pools.
Veins
Most veins have valves to prevent reflux of blood (Fig. 6.13, Fig. 6.14). A valve is formed by an inward projection of the intima, strengthened by collagen and elastic fibres, and covered by endothelium which differs in orientation on its two surfaces. Surfaces facing the vessel wall have transversely arranged endothelial cells, whereas on the luminal surface of the valve, over which the main stream of blood flows, cells are arranged longitudinally in the direction of flow. Most commonly two, or occasionally three, valves lie opposite one another, sometimes only one is present. They are found in small veins or where tributaries join larger veins. The valves are semilunar (cusps) and attached by their convex edges to the venous wall. Their concave margins are directed with the flow and lie against the wall as long as flow is towards the heart. When blood flow reverses, the valves close and blood fills an expanded region of the wall, a sinus, on the cardiac side of the closed valve. This may give a ‘knotted’ (varicose) appearance to the distended veins, if these have many valves. In the limbs, especially the legs where venous return is against gravity, valves are of great importance to venous flow. Blood is moved towards the heart by the intermittent pressure produced by contractions of the surrounding muscles. Valves are absent in veins of the thorax and abdomen.
VASCULAR SHUNTS AND ANASTOMOSES
Arteriovenous shunts and anastomoses
Communications between the arterial and venous systems are found in many regions of the body. In some parts of the microcirculation (e.g. mesentery), the capillary circulation can be bypassed by wider thoroughfare channels formed by metarterioles (Fig. 6.15A). These have similarities to both capillaries and the smallest arterioles, and have a discontinuous layer of smooth muscle in their walls. Metarterioles can deliver blood directly to venules or to a capillary bed, according to local demand and conditions. When functional demand is low, blood flow is largely limited to the bypass channel. Periodic opening and closing of different arterioles irrigates different parts of the capillary network. The number of capillaries in individual microvascular units and the size of their mesh determine the degree of vascularity of a tissue: the smallest meshes occur in the lungs and the choroid of the eye.
Arteriovenous anastomoses (Fig. 6.15B) are direct connections between smaller arteries and veins. Connecting vessels may be straight or coiled, and often possess a thick muscular tunic. Under sympathetic control, the vessel is able to close completely, diverting blood into the capillary bed. When patent, the vessel carries blood from artery to vein, partially or completely excluding the capillary bed from the circulation. Simple arteriovenous anastomoses are widespread and occur notably in the skin of the nose, lips and ears, nasal and alimentary mucosae, erectile tissue, tongue, thyroid gland and sympathetic ganglia. In the newborn child, there are few arteriovenous anastomoses, but they develop rapidly during the early years. In old age they atrophy, sclerose and diminish in number. These factors may contribute to the less efficient temperature regulation which occurs at the two extremes of age.
In the skin of the hands and feet, especially in digital pads and nail beds (see Fig. 7.18), anastomoses form a large number of small units termed glomera. Each glomus organ has one or more afferent arteries, stemming from branches of cutaneous arteries which approach the surface. The afferent artery gives off a number of fine periglomeral branches and then immediately enlarges, makes a sinuous curve, and narrows again into a short funnel-shaped vein which opens at right angles into a collecting vein (Fig. 6.16).
The angiosome concept and vascular territories
An angiosome is a three-dimensional block of tissue (known as an anatomical territory) supplied by a source artery and its accompanying veins. It can be a composite of skin, underlying fascia, muscle and bone. These blocks of tissue form a complex three-dimensional jigsaw puzzle: some pieces have a predominantly cutaneous component while others are predominantly muscular. Each angiosome is made up of arteriosomes and venosomes and they are linked to neighbouring angiosomes by either simple anastomoses composed of similar calibre vessels or reduced calibre vessels termed choke vessels. The anastomoses between adjacent angiosomes can occur within the skin or within muscle. Some muscles are supplied by a single artery and its accompanying veins and therefore lie within one angiosome, while other muscles are supplied by more than one vessel and therefore cross more than one angiosome.
FUNCTIONAL MICROSTRUCTURE OF VESSELS
Intima
Endothelium
The endothelium is a monolayer of flattened polygonal cells which extends continuously over the luminal surface of the entire vascular tree (Fig. 6.17, see Fig. 6.10). Its structure varies in different regions of the vascular bed.
The endothelium is a key component of the vessel wall, and subserves several major physiological roles. Endothelial cells are in contact with the bloodstream and thus influence blood flow. They regulate the diffusion of substances and migration of cells out of and into the circulating blood. In the brain, endothelial cells of small vessels actively transport substances, e.g. glucose, into the brain parenchyma. Endothelial cells participate in the formation of blood clots (by secreting clot-promoting factors – von Willebrand factor); in minimizing clot formation (by secreting prostacyclin and thrombomodulin); and in the process of clot dissolution or fibrinolysis (by secreting tissue plasminogen activator). They have selective phagocytic activity and are able to extract substances from the blood. For example, the endothelium of pulmonary vessels removes and inactivates several polypeptides, biogenic amines, bradykinin, prostaglandins and lipids from the circulation. Endothelial cells secrete both vasoconstrictive (thromboxane) and vasodilator (prostacyclin) prostaglandins, nitric oxide (NO, relaxing factor) and endothelin (a vasoconstrictor), which affect the tone of smooth muscle in vessel walls. They are sensitive to stretch (e.g. increased pressure) and the shear effect of blood flow, via stretch-sensitive ion channels in the cell membrane. Endothelial cells synthesize components of the basal lamina. They proliferate to provide new cells during the growth in size of a blood vessel, to replace damaged endothelial cells, and to provide solid cords of cells which develop into new blood vessels (angiogenesis). Angiogenesis, which may be stimulated by endothelial production of autocrine growth factors (see Ch. 1) in response to locally low oxygen tension, is important in wound healing, and in the growth of tumours. Endothelial cells are also active participants in, and regulators of, inflammatory processes (reviewed in Pober & Sessa, 2007).
Endothelial cells are thin but extend over a relatively large surface area. They are generally elongated in the direction of blood flow, especially in arteries (see Fig. 6.17). They usually adhere firmly to each other at their edges, so that the lining of the lumen presents no discontinuity, other than in sinusoids. The thickness of endothelial cells is maximal at the level of their nucleus, where it can reach 2–3 μm, and this part of the cell often bulges slightly into the lumen (see Fig. 6.10). Elsewhere, the endothelial cell is thinner and laminar: in capillaries, these portions of the cell often measure as little as 0.2 μm in thickness.
Transcytotic (pinocytotic) vesicles (see Fig. 6.11) are present in all endothelial cells, but are particularly numerous in exchange vessels; they include caveolae (see Fig. 6.4) typical of smooth muscle cells. They shuttle small amounts of extracellular fluid or blood plasma across the endothelial cytoplasm and thus facilitate the bulk exchange of dissolved gases, nutrients and metabolites between these compartments. In spite of the factors known to be released by endothelial cells, they do not have the morphological characteristics of secretory cells.
Endothelial–leukocyte interactions
HEV (Fig. 6.18) are located within the T cell domains, between and around lymphoid follicles in all secondary lymphoid organs and tissues except the spleen. They are specialized venules of 7–30 μm diameter, which possess a conspicuous cuboidal endothelial lining. The luminal aspect of HEVs shows a cobblestone appearance. The endothelial cells rest on a basal lamina and are supported by pericytes and a small amount of connective tissue (Fig. 6.19). They are linked by discontinuous adhesive junctions at their apical and basal aspects: the junctions are circumnavigated by migrating lymphocytes. Ultrastructurally, the endothelial cells have the characteristics of metabolically active secretory cells. They contain large, rounded euchromatic nuclei with one or two nucleoli, prominent Golgi complexes, many mitochondria, ribosomes and pinocytotic vesicles. Typically, they also possess Wiebel–Palade bodies (see above).
The first step in this cascade is the loose binding or tethering of leukocytes, and is initiated via L-, P- or E-selectin (see below). This weak, reversible adhesion allows leukocytes to roll along the endothelial surface of a vessel lumen at low velocity, making and breaking contact, and sampling the endothelial cell surfaces. Recognition of chemokines (chemotactic signalling molecules) presented by the endothelium leads to ‘inside-out’ signalling and conversion of integrins at the leukocyte surface into actively adhesive configurations which bind strongly to their endothelial ligands, resulting in stable arrest. Finally, the leukocyte migrates through the vessel wall (diapedesis), passing between (paracellular migration) or across (transcellular migration) endothelial cells. It is now thought that transcellular migration is the preferred pathway and endothelial transcytotic vesicles (caveolae), intermediate filaments (vimentin) and F-actin are important in the creation of transient transcellular channels through which leukocytes pass. They then cross the basal lamina and migrate into the surrounding tissue by mechanisms which involve CD31 antigen and matrix metalloproteinases (see Ch. 2).
Subendothelial connective tissue
The subendothelial connective tissue, also termed the lamina propria, is a thin but variable layer. It is largely absent in the smallest vessels, where the endothelium is supported instead by pericytes (see Fig. 6.21). It contains a typical fibrocollagenous extracellular matrix, a few fibroblasts and occasional smooth muscle cells. Endothelial von Willebrand factor concentrates in this layer and participates in the clotting process when the overlying endothelium is damaged.
Media
Smooth muscle
Smooth muscle forms most of the media of arteries (see Fig. 6.8) and arterioles. A thinner layer of smooth muscle is also found in venules and veins, with the exception of small segments of the pulmonary veins, where striated cardiac muscle is present in the portions nearest to the heart. Contraction of the smooth muscle in arteries and arterioles reduces the calibre of the vessel lumen, which reduces blood flow through the vessel and raises the pressure on the proximal side. This role is particularly effective in small resistance vessels where the wall is thick, relative to the diameter of the vessel. Smooth muscle can also alter the rigidity of the wall, without causing constriction (isometric contraction), and this affects the distensibility of the wall and propagation of the pulse.
Collagen and elastin
Elastin is found in all arteries and veins and is especially abundant in elastic arteries (see Fig. 6.6). Individual elastic fibres (0.1–1.0 μm in diameter) anastomose with each other to form net-like structures, which extend predominantly in a circumferential direction. More extensive fusion produces lamellae of elastic material, which though usually perforated and thus incomplete, separate the layers of muscle cells. A conspicuous elastic lamella, the internal elastic lamina, is seen in arteries, between intima and media. This is a tube of elastic material which allows the vessel to recoil after distension. When the intraluminal pressure falls below physiological limits (postmortem), the inner elastic lamina is compressed and it coils up into a regular corrugated shape (Fig. 6.20, see Figs 6.8, 6.10): in these conditions the lumen is much reduced but is not obliterated, and the profile of the artery remains circular. Fenestrations in the elastic lamina, which may also be split in thickness, allow materials to diffuse between intima and media. An outer elastic lamina, similar in appearance to, but markedly less well developed and less compact than the internal elastic lamina, lies at the outer aspect of the media at its boundary with the adventitia (see Fig. 6.8). These laminae are less evident in elastic arteries, where elastic fibres occupy much of the media (see Fig. 6.6).
Collagen fibrils are found in all three vessel layers. Type III collagen (reticulin) occupies much of the interstitial space between the muscle cells of the media, and is also found in the intima. Collagen is abundant in the adventitia, where type I collagen fibres form large bundles which increase in size from the junction with the media to the outer limit of the vessel wall. In veins, collagen is the main component of the vessel wall, and accounts for more than half its mass.
Adventitia
Vasa vasorum
In smaller vessels, the nourishment of the tissues of the vessel wall is provided by diffusion from the blood circulating in the vessel itself. Large vessels have their own vascular supply within the adventitia (see Figs 6.7, 6.8), in the form of a network of small vessels, mainly of the microcirculation, which are called the vasa vasorum. The wall thickness at which simple diffusion from the lumen becomes insufficient is 1 mm.
Pericytes
Pericytes are present at the outer surface of capillaries and the smallest venules (postcapillary venules), where an adventitia is absent and there are no muscle cells. They are elongated cells, whose long cytoplasmic processes are wrapped around the endothelium. Pericytes are scattered in a discontinuous layer around the outer circumference of capillaries. They are generally absent from fenestrated capillaries, but form a more continuous layer around postcapillary venules (Fig. 6.21). They are gradually replaced by smooth muscle cells as vessels converge and increase in diameter.
Cerebral vessels
As arteries enter the subpial space and penetrate the brain, they lose their elastic laminae, and consequently the cerebral cortex and white matter typically contain only arterioles, venules and capillaries. The exceptions are the large penetrating vessels in the basal ganglia, where many arteries retain their elastic laminae and thick smooth muscle media. Enlarged perivascular spaces form around these large arteries in ageing individuals. Arterioles and venules in the cortex and white matter can be distinguished from each other because arterioles are surrounded by a smooth muscle coat, and the veins and venules have larger lumina and thinner walls.
Cerebral capillaries are the site of the blood–brain barrier (p. 49). They are lined by endothelial cells which are joined by tight junctions. The endothelial cytoplasm contains a few pinocytotic vesicles. The cells are surrounded by a basal lamina (see Fig. 3.13): at points of contact with perivascular astrocytes the intervening basal lamina is formed by fusion of the endothelial and glial basal laminae. Pericytes, completely surrounded by basal lamina, are present around capillaries. Perivascular macrophages are attached to the outer walls of capillaries and to other vessels: they are phenotypically distinct from parenchymal microglia, which are also of monocytic origin. A thin layer of meningeal cells derived from the pia mater surrounds arterioles but disappears at the level of capillaries. For further descriptions of cerebral vessels, see Ch. 17.
LYMPHATIC VESSELS
Lymph is formed from interstitial fluid, which is derived from blood plasma via the microcirculation. Much of this fluid is returned to the venous system. Lymphatic vessels take up residual fluid (about one tenth) by passive diffusion and the transient negative pressures in their lumina which are generated intrinsically by contractile activity of smooth muscle in the largest lymphatic vessel walls, and extrinsically, by movements of other tissues (muscles, arteries) locally. The unidirectional flow of lymph is maintained by the presence of valves in the larger vessels (see Fig. 6.22). Lymphatic capillaries are prevented from collapsing by anchoring filaments which tether their walls to surrounding connective tissue structures and exert radial traction.
The larger lymphatic vessels differ from small veins in having many more valves (Fig. 6.22). The valves are semilunar, generally paired and composed of an extension of the intima. Their edges point in the direction of the current, and the vessel wall downstream is expanded into a sinus, which gives the vessels a beaded appearance when they are distended. Valves are important in preventing the backflow of lymph.
CARDIAC MUSCLE
MICROSTRUCTURE
The myocardium, the muscular component of the heart, constitutes the bulk of its tissues. It consists predominantly of cardiac muscle cells, which are usually 120 μm long and 20–30 μm in diameter in a normal adult. Each cell has one or two large nuclei, occupying the central part of the cell, whereas skeletal muscle has multiple, peripherally placed nuclei. The cells are branched at their ends, and the branches of adjacent cells are so tightly associated that the light microscopic appearance is of a network of branching and anastomosing fibres (Fig. 6.23). Cells are bound together by elaborate junctional complexes, the intercalated discs (Fig. 6.23, Fig. 6.24, see Fig. 6.26).
Fine fibrocollagenous connective tissue is found between cardiac muscle fibres. Although this is equivalent to the endomysium of skeletal muscle, it is less regularly organized because of the complex three-dimensional geometry imposed by the branching cardiac cells. Numerous capillaries and some nerve fibres are found within this layer. Coarser connective tissue, equivalent to the perimysium of skeletal muscle, separates the larger bundles of muscle fibres, and is particularly well developed near the condensations of dense fibrous connective tissue that form the ‘skeleton’ of the heart (see Ch. 56). The ventricles of the heart are composed of spiralling layers of fibres which run in different directions. Consequently, microscope sections of ventricular muscle inevitably contain the profiles of cells cut in a variety of orientations. A linear arrangement of cardiac muscle fibres is found only in the papillary muscles and trabeculae carneae.
Electron micrographs of cardiac muscle cells in longitudinal section (Fig. 6.25) show that the myofibrils separate before they pass around the nucleus, leaving a zone that is occupied by organelles, including sarcoplasmic reticulum, Golgi complex, mitochondria, lipid droplets, and glycogen. At the light microscopic level, these zones appear in longitudinal sections as unstained areas at the poles of each nucleus. They often contain lipofuscin granules, which accumulate there in individuals over the age of 10; the reddish-brown pigment may be visible even in unstained longitudinal sections.
The sarcolemma of ventricular cardiac muscle cells invaginates to form T-tubules with a wider lumen than those of skeletal muscle; atrial muscle cells have few or no T-tubules. Unlike skeletal muscle, most T-tubules penetrate the sarcoplasm at the level of the Z-discs (Fig. 6.24). The T-tubules are interconnected at intervals by longitudinal branches to form a complex network. They probably serve a similar function in skeletal and cardiac muscle, i.e. to carry the wave of depolarization into the core of the cells. The actin-binding proteins, spectrin and dystrophin, are important components of the cardiac muscle cell cytoskeleton, which associate independently with the sarcolemma to provide mechanical support.
Intercalated discs
Intercalated discs are unique to cardiac muscle. In the light microscope they are seen as transverse lines crossing the tracts of cardiac cells (see Fig. 6.23). They may step irregularly within or between adjacent tracts, and may appear to jump to a new position as the plane of focus is altered. At the ultrastructural level these structures, which are complex junctions between the cardiac muscle cells, are seen to have transverse and lateral portions (see Fig. 6.24, Fig. 6.26). The transverse portions occur wherever myofibrils abut the end of the cell, and each takes the place of the last Z-disc. At this point, the actin filaments of the terminal sarcomere insert into a dense subsarcolemmal matrix which anchors them, together with other cytoplasmic elements such as intermediate filaments, to the plasma membrane. Prominent desmosomes, often with a dense line in the intercellular space, occur at intervals along each transverse portion. This junctional region is homologous with, and probably similar in composition to, the structure found on the cytoplasmic face of the myotendinous junction, and is a type of fascia adherens junction. It provides firm adhesion between cells, and a route for the transmission of contractile force from one cell to the next.
The lateral portions of the intercalated disc run parallel to the myofilaments, and the long axis of the cell, for a distance which corresponds to one or two sarcomeres before it turns again to form another transverse portion. It is therefore responsible for the stepwise progression of the intercalated disc which can be seen microscopically. The lateral portions contain gap junctions, which are responsible for the electrical coupling between adjacent cells (see Fig. 6.26). Conductance channels within these junctions enable the electrical impulse to propagate from one cell to the next, spreading excitation and contraction rapidly along the branching tracts of interconnected cells. In this way the activity of the individual cells of the heart is coordinated so that they function as if they were a syncytium.
EXCITATION–CONTRACTION COUPLING IN CARDIAC MUSCLE
The calcium release channels of the sarcoplasmic reticulum are sensitive to the concentration of free calcium in the gap between the T-tubule and sarcoplasmic reticulum membranes. This underlies ‘calcium-induced calcium release’, which is believed to be the principal, and probably the only, mechanism involved in the liberation of calcium from the sarcoplasmic reticulum during physiological activation. The passage of an action potential depolarizes the sarcolemma and thereby opens sarcolemmal L-type calcium channels, which allows some calcium to enter from the extracellular space. This produces a localized rise in the intracellular free calcium concentration near the calcium release channels, which consequently open, allowing calcium ions to flow down their concentration gradient from the sarcoplasmic reticulum into the cytosol. The rise in cytosolic calcium concentration then activates the contractile machinery.
DEVELOPMENT
Contractile protein isoforms of cardiac muscle
The myosin heavy chain of human cardiac muscle exists in two isoforms, α and β, both of which are present in the fetal heart. The α-form persists as the adult isoform in atrial muscle, whereas the β-form (which is associated with a slower rate of contraction) predominates in ventricular muscle. Interestingly, the β-form of myosin heavy chain in cardiac muscle is identical to the isoform in slow twitch skeletal muscle. This identity between cardiac and slow twitch skeletal protein isoforms is true of several proteins, including ventricular myosin light chains and cardiac troponin-C. Other proteins, such as troponin-I and -T, exist in cardiac-specific forms in the adult, although skeletal isoforms are expressed in the fetus and neonate. The appearance of cardiac-specific isoforms of troponin-I and -T in the blood, following their release from damaged cardiac cells, is now a standard diagnostic test for myocardial infarction.
Lack of regeneration of cardiac muscle
In skeletal muscle, a population of precursor cells, satellite cells, is retained in adult life, and constitutes a pool of myoblasts which is capable of dividing, fusing with existing muscle fibres, and initiating regeneration after damage. Cardiac muscle contains no equivalent of these cells, and is therefore incapable of regeneration. There is experimental evidence that temporary ischaemia injures cardiac cells in a reversible manner (Schwanger et al 1987), whereas longer periods of ischaemia produce irreversible damage.
Imhof BA, Aurrand-Lions M. Angiogenesis and inflammation face-off. Nat Med. 2006;12:171-172.
Owens GK, Kumar MS, Wamhoff BR. Molecular regulation of vascular smooth muscle cell differentiation in development and disease. Physiol Rev. 2004;84:767-801.
Pober JS, Sessa WC. Evolving functions of endothelial cells in inflammation. Nat Revs Immunol. 2007;7:803-815.
Scaal M, Christ B. Formation and differentiation of the avian dermomyotome. Anat Embryol. 2004;208:411-424.
Schwanger M, Fishbein MC, Block M, Wijns W, Selin C, Phelps ME, Schelbert HR. Metabolic and ultrastructural abnormalities during ischemia in canine myocardium: noninvasive assessment by positron emission tomography. Mol Cell Cardiol. 1987;19:25-89.
Sparrow MP, Weichselbaum M, McCray PBJr. Development of the innervation and airway smooth muscle in human fetal lung. Am J Respir Cell Mol Biol. 1999;20:550-560.
Michel Salmon: Anatomic Studies. In: Taylor GI, Razaboni RM. Book 1, Arteries of the Muscles of the Extremities and the Trunk. Book 2, Arterial Anastomotic Pathways of the Extremities. St Louis: Quality Medical Publishing, 1994.