Skeletal muscle relaxants (neuromuscular blocking agents)
After reading this chapter, the reader will be able to:
1. Define terms that pertain to skeletal muscle relaxants
2. Define neuromuscular blocking agents (NMBAs)
4. Describe the physiology of the neuromuscular junction
5. Describe the makeup of nondepolarizing agents
6. Describe the makeup of depolarizing agents
Enzyme that breaks down the neurotransmitter acetylcholine at the synaptic cleft so that the next nerve impulse can be transmitted across the synaptic gap.
Having the ability to cause total or partial loss of memory.
Accidental inhalation of food particles, fluids, or gastric contents into the lungs.
Involuntary contractions or twitching of groups of muscle fibers.
Autoimmune neuromuscular disorder characterized by chronic fatigue and exhaustion of muscles.
Neuromuscular blocking agent (NMBA)
Substance that interferes with the neural transmission between motor neurons and skeletal muscles.
A basic functional unit of the nervous system that is specialized to transmit electrical nerve impulses and carry information from one part of the body to another. A neuron consists of a cell body, axons, and dendrites.
Chemical that is released from a nerve ending to transmit an impulse from a nerve cell to another nerve, muscle, organ, or other tissue.
Pneumonia that is acquired in a health care setting.
Molecular structure inside or outside the cell that binds to a specific substance to elicit a physiologic response.
Production of a restful state of mind, particularly by the use of drugs that have a calming effect, relieving anxiety and tension.
Part of the nervous system that controls muscles that are under voluntary control.
Exacerbation of asthma that does not respond to standard treatment.
At least 30 minutes of continuous seizure activity without full recovery between seizures.
Uses of neuromuscular blocking agents
The clinical uses of NMBAs are as follows:
• To facilitate endotracheal intubation
• To obtain muscle relaxation during surgery, particularly of the thorax and abdomen
• To enhance patient-ventilator synchrony
• To reduce intracranial pressure in intubated patients with uncontrolled intracranial pressure
• To reduce oxygen consumption
• To terminate convulsive status epilepticus and tetanus in patients refractory to other therapies
• To facilitate procedures or diagnostic studies
• To paralyze selected patients who must remain immobile (e.g., trauma patients)
Physiology of the neuromuscular junction
The autonomic nervous system consists of the central nervous system (CNS) and the peripheral nervous system (PNS). The CNS consists of the brain and the spinal cord, and the PNS includes all the nerves outside of the CNS. The PNS is divided further into the somatic motor neurons, sensory afferent neurons, and autonomic motor neurons. The somatic motor neurons include all the peripheral nerves that control skeletal muscle over which humans have control of “voluntary” movement. Examples of skeletal muscles include the quadriceps, biceps, diaphragm, and accessory muscles of ventilation, which are responsible for motor functions such as movement, lifting, and breathing. The autonomic motor neurons include peripheral nerves that control smooth muscle (e.g., wall of the digestive system, vascular smooth muscle), cardiac muscle (rate and force of contraction), and glands (e.g., adrenal medulla, sweat glands, exocrine glands of the pancreas). For a review, refer to Chapter 5.
The basic nerve cell, or neuron, consists of a cell body, axons, and dendrites (Figure 18-1). The cell bodies of somatic motor neurons, located in the spinal cord, stimulate skeletal muscles via the axons running through the peripheral nerves. These axons are large myelinated nerve fibers extending from the peripheral nerve cell bodies to the muscle fibers. A single peripheral nerve branches and innervates many different muscle fibers as a motor unit. The area between the nerve and muscle, or synapse, is specialized into a motor end plate. This area between the axon and the skeletal muscle fiber is also termed the neuromuscular junction (Figure 18-2).
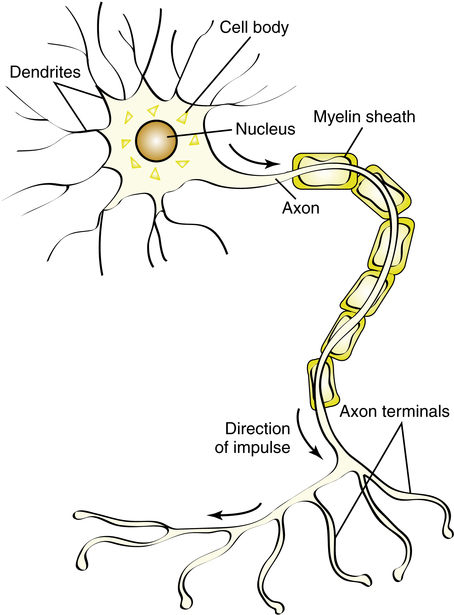
1. Competitive inhibition: The binding and blocking of the Ach receptors without depolarization; this is the action of the nondepolarizing agents
2. Prolonged occupation and persistent binding of the Ach receptors: Resulting in sustained depolarization of the neuromuscular junction; this is the action of the depolarizing agents
Both depolarizing and nondepolarizing agents resemble the neurotransmitter Ach. Table 18-1 reviews both types of neuromuscular blocking agent, including major chemical classification, duration of action, and elimination route.
TABLE 18-1
Classification of Neuromuscular Blocking Agents (NMBAs)
AGENT | CHEMICAL CLASS | PHARMACOLOGIC PROPERTIES | TIME OF ONSET (min) | CLINICAL DURATION (min) | MODE OF ELIMINATION |
Depolarizing NMBAs | |||||
Succinylcholine (Anectine) | Dicholine ester | Ultra-short duration | 1-1.5 | 10-15 | Hydrolysis by plasma cholinesterases |
Nondepolarizing NMBAs | |||||
Tubocurarine | Natural alkaloid (cyclic benzylisoquinoline) | Long duration; competitive | 4-6 | 80-120 | Renal elimination; liver clearance |
Atracurium (Tracrium) | Benzylisoquinoline ester | Intermediate duration; competitive | 2-4 | 30-60 | Hofmann degradation; hydrolysis by plasma esterases; renal elimination |
Cisatracurium (Nimbex) | Benzylisoquinoline ester | Intermediate duration; competitive | 2-3 | 40-60 | Hofmann degradation; hydrolysis by plasma esterases; renal elimination |
Doxacurium (Nuromax) | Benzylisoquinoline ester | Long duration; competitive | 4-6 | 90-120 | Renal elimination |
Mivacurium (Mivacron) | Benzylisoquinoline ester | Short duration; competitive | 2-4 | 12-18 | Hydrolysis by plasma cholinesterases |
Pancuronium (Pavulon) | Ammonio steroid | Long duration; competitive | 4-6 | 120-180 | Renal elimination |
Pipecuronium (Arduan) | Ammonio steroid | Long duration; competitive | 2-4 | 80-100 | Renal elimination; liver metabolism and clearance |
Rocuronium (Zemuron) | Ammonio steroid | Intermediate duration; competitive | 1-2 | 30-60 | Liver metabolism |
Vecuronium (Norcuron) | Ammonio steroid | Intermediate duration; competitive | 2-4 | 60-90 | Liver metabolism and clearance; renal elimination |
Data from Brunton LL, Lazo JS, Parker KL, editors: Goodman & Gilman’s the pharmacological basis of therapeutics, ed 10, New York, 2005, McGraw-Hill.
Nondepolarizing agents
Mechanism of action
Nondepolarizing agents cause muscle paralysis by affecting the postsynaptic cholinergic receptors at the neuromuscular junction. By either blocking the channel externally, occupying the channel pore, or affecting the receptor from the internal side of the muscle membrane, these agents reduce the frequency of channel opening. Nondepolarizing agents compete against endogenous Ach for receptor occupancy. Muscle contraction does not occur if enough sites are blocked by these agents. This is illustrated in Figure 18-3, in which the drug (ND) occupies and then blocks the postsynaptic site at the neuromuscular junction. With nondepolarizing agents, depolarization of the postsynaptic membrane becomes a function of the amount of drug and the amount of Ach located around the receptor. In other words, because nondepolarizing agents act by competitive inhibition, their effect is dose related: Larger doses overcome the effects of Ach and block more receptors. The receptor blockade by nondepolarizing agents can be reversed by making more Ach available to compete for receptor sites. Inhibitors of AchE, an enzyme that breaks down Ach, can be used to reverse the competitive blockade. Neostigmine is an example of a cholinesterase inhibitor.
Pharmacokinetics of nondepolarizing agents
The onset of paralysis and the duration of action of nondepolarizing blockers vary widely among members of this group of drugs. Tubocurarine, one of the first agents in this class, has a maximal paralyzing action that lasts 35 to 60 minutes, and complete recovery may take several hours. Pancuronium, pipecuronium, and doxacurium have durations of action similar to tubocurarine, and all of these agents are considered to be long-acting NMBAs. Atracurium, cisatracurium, vecuronium, and rocuronium are redistributed more rapidly and have shorter durations of action than tubocurarine. These agents are considered intermediate-acting NMBAs.1
As previously noted, the magnitude of effect, rate of onset of maximum blockade, and duration of action of NMBAs is dose-dependent; this is illustrated in Table 18-2 for the drug rocuronium when used in adults. Factors such as advanced age generally increase the length of neuromuscular blockade activity. Hepatic or renal failure can cause decreased clearance, increased blood levels, and prolonged duration of action for agents metabolized and eliminated by the liver and kidney.
TABLE 18-2
Dose-dependent Effects of Rocuronium in Adults
DOSE (mg/kg) | TIME TO MAXIMUM BLOCK (min) | CLINICAL DURATION (min) |
0.45 | 3 | 22 |
0.6 | 1.8 | 31 |
0.9 | 1.4 | 58 |
1.2 | 1 | 67 |
Data from Drug facts and comparisons, St Louis, 2006, Facts & Comparisons, Wolters Kluwer Health.
Metabolism
Atracurium further differs from cisatracurium because it has a breakdown product of Hofmann degradation called laudanosine. Laudanosine, which is eliminated primarily by the kidneys and is slowly metabolized by the liver, has a long half-life and can cross the blood-brain barrier. Laudanosine has been associated with neurostimulatory effects. CNS excitation and seizures should be considered as a possible complication, especially in patients receiving atracurium who have impaired renal function or liver failure.2 Cisatracurium has less laudanosine production than atracurium and is considered more potent. The risk of further brain injury from seizures in patients with poor intracranial compliance (e.g., severe head injury) may make these drugs poor choices in these patients. Seizure activity might be masked by these agents, complicating assessment of the patient.
Mivacurium is one of the shortest acting NMBAs available (effect of 10 to 20 minutes in usual doses). It is unique among nondepolarizing agents in that it is eliminated by plasma cholinesterase, similar to succinylcholine, a depolarizing agent. Metabolism of mivacurium is independent of kidney or liver function.3 However, patients with renal failure or liver dysfunction may have decreased levels of plasma cholinesterase, prolonging the duration of action of mivacurium. The effects of organ failure on NMBA duration are illustrated in Table 18-3.
TABLE 18-3
AGENT | PROLONGATION OF EFFECT WITH RENAL FAILURE | PROLONGATION OF EFFECT WITH HEPATIC FAILURE | ALTERNATIVE METABOLISM |
Atracurium | 0 | 0 | Hofmann degradation, ester hydrolysis |
Cisatracurium | 0 | 0 | Hofmann degradation, ester hydrolysis |
Tubocurarine | + | + | |
Doxacurium | +++ | 0 | |
Mivacurium | 0 | 0 | Plasma cholinesterase* |
Pancuronium | +++ | + | |
Pipecuronium | +++ | 0 | |
Rocuronium | + | ++ | |
Succinylcholine | 0 | 0 | Plasma cholinesterase* |
Vecuronium | ++† | + |
0, No effect; +, ++, +++, degree of effect.
*Atypical pseudocholinesterase may prolong relaxant effect dramatically.
†Metabolic product is one-third as potent as the parent compound and is entirely removed by renal excretion.
Adverse effects
Cardiovascular effects
It is important to understand that the nondepolarizing NMBAs also competitively block Ach receptors at the autonomic ganglia, producing cardiovascular side effects on heart rate and blood pressure. They may cause a vagolytic effect, which produces tachycardia, and an increase in mean arterial pressure by promoting an increase in norepinephrine, a potent vasoconstrictor.3 Pancuronium has the greatest potential to cause cardiovascular side effects, especially tachycardia and hypertension. Agents such as vecuronium, doxacurium, and cisatracurium have minimal effects on heart rate and blood pressure.
Histamine release
All of the nondepolarizing agents have a tendency to release histamine from mast cells. However, the potential for adverse cardiac effects varies among the different agents. Clinically, histamine release can cause hypotension secondary to direct vasodilation, reflex tachycardia, and bronchospasm, leading to increased airway resistance. The vasodilatory effect may also give the appearance of skin flushing. The degree of histamine release for several NMBAs is shown in Table 18-4. Tubocurarine is the most potent releaser of histamine and causes the most profound problems with intravenous bolus administration. Among the newer agents, mivacurium and atracurium have been reported to stimulate the most histamine release. It is recommended that these agents be given at a reduced rate or at lower doses to avoid these effects. Antihistamines may also be administered as pretreatment to avoid such effects.
TABLE 18-4
Comparison of Side Effects of Neuromuscular Blocking Agents
AGENT | HISTAMINE RELEASE | BLOCKADE OF AUTONOMIC GANGLIA | BLOCKADE OF VAGAL RESPONSE | VAGAL STIMULATION |
Atracurium | ++ | 0 | 0 | 0 |
Cisatracurium | + | 0 | 0 | 0 |
Tubocurarine | ++++ | +++ | 0 | 0 |
Doxacurium | + | 0 | 0 | 0 |
Mivacurium | ++ | 0 | 0 | 0 |
Pancuronium | + | ++ | ++ | 0 |
Pipecuronium | + | 0 | 0 | 0 |
Rocuronium | + | 0 | + | 0 |
Succinylcholine | + | 0 | 0 | +++ |
Vecuronium | 0 | 0 | 0 | 0 |
Reversal of nondepolarizing blockade
Muscle paralysis caused by nondepolarizing NMBAs can be reversed by use of cholinesterase inhibitors such as neostigmine. Neostigmine inhibits the cholinesterase that would normally break down Ach. This action allows for more Ach to be available at the neuromuscular junction to compete with and displace the blocker from receptor sites. Other cholinesterase inhibitors include edrophonium and pyridostigmine. Edrophonium is rapid-acting but also has the shortest duration of action. Pyridostigmine has a slower onset and is the longest acting; it is often used to treat myasthenia gravis and can be given orally. Neostigmine is intermediate in onset and duration of action. Table 18-5 summarizes these agents with recommended doses to reverse neuromuscular blockage produced by the nondepolarizing agents.4
TABLE 18-5
Agents Used for Reversal and Antimuscarinic Effects with Nondepolarizing Blocking Agents
AGENT | DOSE (mg/kg) | TIME FOR EFFECT |
Reversal Agents | ||
Edrophonium | 0.3-1.0 | Rapid onset, short acting |
Pyridostigmine | 0.1-0.25 | Slowest onset, longest acting |
Neostigmine | 0.01-0.035 | Intermediate onset and duration |
Antimuscarinic Agents | ||
Atropine | 0.008-0.018 | Rapid onset, short acting |
Glycopyrrolate | 0.002-0.016 | Rapid onset, short acting |
Data from Buck ML, Reed MD: Use of nondepolarizing neuromuscular blocking agents in mechanically ventilated patients, Clin Pharm 10:32, 1991.
Because the reversing agents increase the levels of Ach, they also increase the effects of Ach at parasympathetic ganglia, producing cholinergic autonomic side effects. Major side effects of these agents include severe bradycardia and salivation. To reduce these adverse effects, agents such as atropine or glycopyrrolate are also given in conjunction with cholinesterase inhibitors. As vagolytic and anticholinergic agents, atropine and glycopyrrolate, respectively, prevent bradycardia, increased salivation, and hyperperistalsis associated with excessive Ach.5
Depolarizing agents
Mechanism of action
The initial action of depolarizing NMBAs is to open sodium channels and depolarize the postsynaptic muscle membrane in the same manner as Ach. Depolarizing agents are resistant to the effects of AchE, allowing for a persistent and longer duration at the neuromuscular junction. Because depolarization lasts longer, the membrane is unable to repolarize, resulting in flaccid muscles.5 This is illustrated in Figure 18-4, in which molecules of succinylcholine (S) have occupied two Ach receptors, each opening a pore and allowing the local membrane to become permeable to sodium. If enough receptors are activated, depolarization occurs and is maintained until succinylcholine leaves the receptors. Further stimulation and contraction of the muscle fiber is impossible until the drug is removed by redistribution and metabolism.
Adverse effects
Succinylcholine produces many side effects, several of which can be life-threatening. The significance of these side effects may be of more concern in the ICU than during routine operating room use. Most adult patients have a sympathomimetic response causing tachycardia and an increase in blood pressure. Repeated bolus doses of succinylcholine may produce vagal responses, including bradycardia and hypotension. This side effect is seen more often in children. Succinylcholine also provokes histamine release, resulting in bronchospasm and hypotension in susceptible individuals.5
Muscle pain and soreness similar to myalgias are common after the administration of succinylcholine. A relationship between the pain and muscle fasciculations has been implicated but not confirmed. Some practitioners administer a small dose of a nondepolarizing blocker (e.g., 10% of the intubating dose) before giving succinylcholine to reduce fasciculations and pain.6 This pretreatment is often referred to as defasciculation. In patients receiving a nondepolarizing agent to prevent fasciculations, higher doses of succinylcholine are needed for complete paralysis because pretreatment reduces the effectiveness of succinylcholine. This practice is considered controversial because increased doses of succinylcholine are required, and pretreatment may cause partial paralysis, necessitating urgent intubation under nonideal conditions.7 Muscle fasciculations can also cause an increase in serum potassium and creatinine phosphokinase, an effect that is also reduced but not totally eliminated by pretreatment.
Succinylcholine can cause an efflux of potassium from muscle cells, causing serum potassium to increase by 0.5 to 1.0 mEq/L in normal individuals.6 Patients with spinal cord injury or upper motor neuron lesions, thermal injuries, and severe trauma, including closed head injury, are at a higher risk of developing life-threatening hyperkalemia if succinylcholine is administered. Effects of severe hyperkalemia include arrhythmias and cardiac arrest.
Succinylcholine can dangerously increase intracranial pressure in patients with cerebral edema and head trauma by a mechanism that is not well understood.6 One of the most serious complications that can occur with succinylcholine is malignant hyperthermia. Malignant hyperthermia is caused by a genetic defect of muscle metabolism. It is a potentially fatal hypermetabolic state of skeletal muscles. An uncontrolled release of calcium from the sarcoplasmic reticulum of muscles occurs, resulting in a host of harmful effects. The clinical features can manifest as intractable spasm of the jaw muscles, rigidity, increased oxygen demand, severe hyperthermia, metabolic acidosis, and tachycardia. Malignant hyperthermia is treated with dantrolene, an agent that blocks the release of intracellular calcium from the sarcoplasmic reticulum. Early recognition and treatment are key to ensuring a full recovery.8
Sensitivity to succinylcholine
As mentioned, succinylcholine is metabolized by plasma cholinesterase, which is also called pseudocholinesterase. Patients with abnormal or deficient pseudocholinesterase do not metabolize succinylcholine effectively and experience a prolonged recovery from paralysis. In these patients, prolonged mechanical ventilation support is warranted. A family history of prolonged paralysis after surgery may suggest an abnormality in the enzyme. Laboratory tests are also available to determine the existence of abnormal cholinesterase.9
Neuromuscular blocking agents and mechanical ventilation
• Acute respiratory distress syndrome (ARDS)
• Status asthmaticus, severe bronchospasm
• Certain modes of ventilatory support (e.g., pressure-controlled inverse ratio ventilation, high-frequency oscillatory ventilation)
• Status epilepticus or other intractable convulsive activity
Precautions and risks
Patients receiving prolonged therapy with NMBAs are at risk for developing prolonged skeletal muscle weakness that persists long after the NMBA is discontinued. Myopathy, which may take months to resolve, may be associated more often with steroid-structured agents such as vecuronium and pancuronium (see Table 18-1 for classification), especially when they are combined with corticosteroids such as prednisone. Daily physical therapy with range of motion exercises may lessen the potential for muscle atrophy or wasting in patients receiving prolonged NMBA therapy. Patients given an NMBA should also be turned frequently to prevent the formation of pressure sores and decubitus ulcers. The risk of developing a deep vein thrombosis (DVT) is increased in these patients because of their immobility, making DVT prophylaxis imperative.
Use of sedation and analgesia
Of all adjunctive therapies patients may receive, it is essential to provide adequate sedation and analgesia for ventilated patients receiving a blocking agent. NMBAs cause muscle paralysis without affecting consciousness or the perception of pain. In 1947, a classic experiment that established this fact was performed by Smith and colleagues,10 in which Smith allowed himself to be paralyzed with tubocurarine. He reported full awareness during the paralysis, including sensations of choking while he was unable to swallow and shortness of breath even though he was being adequately ventilated. Neuromuscular blockade is unthinkable without proper sedation and pain control to prevent the nightmare of paralysis with full consciousness and sensory perception. Although many sedative and analgesia agents can cause hemodynamic instability, it is inappropriate to reduce or discontinue sedation and analgesia while a patient is paralyzed to address hemodynamic instability. Because clinical signs of restlessness, distress, and anxiety are lost with neuromuscular blockade, continuous cardiac monitoring is necessary, and vital signs should be assessed closely. Tachycardia, hypertension, diaphoresis, and lacrimation are physiologic responses that can indicate anxiety caused by inadequate sedation or lack of pain control.
For short procedures including endotracheal intubation, a sedative that has amnestic properties should be administered. In surgery, a sedative and an analgesic agent are recommended for all patients. For patients in the ICU, a sedative should be administered on a continuous basis before initiation of neuromuscular blockade. Continuous analgesia should also be used secondary to poor assessment capabilities for pain and the discomfort associated with the constant suctioning and the endotracheal tube itself. Sedatives that have amnestic effects include propofol (Diprivan), lorazepam (Ativan), and midazolam (Versed). It is important to realize that these agents do not provide pain control. Analgesics commonly used for pain control include fentanyl (Sublimaze), hydromorphone (Dilaudid), and morphine. In many situations, deep sedation with continuously infused sedatives and analgesics may prevent the need for a blocking agent in the ICU. Other suggestions for sedation and analgesia are presented in Chapter 20.
Interactions with neuromuscular blocking agents
Clinical factors such as acidosis, hypokalemia, hyponatremia, hypocalcemia, and hypermagnesemia all potentiate neuromuscular blockade. Alkalosis and hypercalcemia are known to inhibit the effects of blockade. Factors affecting the activity of NMBAs are listed in Table 18-6.
TABLE 18-6
Drugs and Conditions That Interact with Nondepolarizing Neuromuscular Blocking Agents
POTENTIATING FACTORS | ANTAGONIZING FACTORS |
Drugs | |
Data from Feldman S, Karalliedde L: Drug interactions with neuromuscular blockers, Drug Saf 15:261, 1996.
Choice of agents
Characteristics of the perfect NMBA (not yet developed) include the following:
• Predictable and controllable duration of action
• Hemodynamic stability at all levels of block and rate of administration
• Predictable kinetics independent of age, gender, and organ dysfunction
The choice of agent for continuous paralysis involves clinical judgment and preference. Currently available nondepolarizing agents can be compared with one another in regard to adverse-effect profile (histamine release and cardiovascular instability), route of elimination, drug interactions, and cost-effectiveness to guide drug choice for paralysis of ventilated patients. Tables 18-3 and 18-4 compare some of these factors for several NMBAs.
The method of drug elimination (e.g., renal or hepatic) is a very important factor in selecting an agent for patients with multiorgan dysfunction syndrome requiring mechanical ventilation. Agents that depend on the liver and kidney for elimination are poorly suited for patients with disease or failure of these organs. The potential effects of organ failure on each agent are described in Table 18-3. In patients with hepatic or renal failure, atracurium, cisatracurium, and mivacurium have the advantage of plasma metabolism and do no rely on hepatic metabolism or renal excretion. The metabolite laudanosine from atracurium metabolism may be of concern in patients with kidney or liver failure. Cisatracurium results in less laudanosine than does atracurium.
Finally, cost is another important consideration in choosing an agent. Many hospitals limit the number of NMBAs available on formulary because of economic issues. Newer, shorter acting agents are very expensive, especially if used for a prolonged period in the ICU. Most hospitals restrict their use to procedures of short duration. Guidelines for blockade use in the ICU have been published and suggest that cost-effective relaxation can be provided with bolus dosing or continuous infusions of pancuronium (if tachycardia is not a concern) or vecuronium in patients with ischemic cardiovascular issues.2 For patients with hepatic and renal dysfunction, cisatracurium or atracurium is the best option. The clinician is advised to reassess the need for continuous paralysis on a daily basis.
Monitoring of neuromuscular blockade
The most commonly used technique for monitoring blockade is the TOF evaluation. In TOF, a supramaximal stimulus at a frequency of 2 Hz is applied to the nerve over 2 seconds. The nonpainful stimuli are delivered as four pulses, one every 0.5 second. The number of twitches that occur, ranging from 0 (100% blockade) to 4 (less than 75% blockade), are measured. Comparison of the strength of the fourth twitch and first twitch predicts the degree of receptor occupancy (Table 18-7). Clinically, the degree of block can be determined by counting the number of twitches seen. Four equal twitches indicate that less than 75% of the receptors are occupied with a blocker. If only three twitches are seen, approximately 80% of receptors are blocked; if only one or two are seen, 90% to 95% are blocked.
TABLE 18-7
Receptor Occupancy Associated with Various Measurements of Neuromuscular Blockade
RECEPTORS OCCUPIED (%) | TWITCH HEIGHT (%) | TRAIN OF FOUR | CLINICAL OBSERVATIONS |
100 | 0 | 0 | Total paralysis, no voluntary movement of any muscle; no PTF |
98-99 | 0 | 0 | Diaphragm may move; PTF present |
95-98 | 1-5 | 1 or 2 twitches | Diaphragm can move minimally; PTF and fade present |
90-95 | 10-25 | 2 or 3 twitches | Breathing inadequate |
75-90 | 10-25 | 4 twitches, 1st > 4th | Tidal volume restored, voluntary movement apparent, can sustain head lift for 5 seconds (75%-80% occupancy), NIP >55 cm H2O, vital capacity 60%-70% of normal |
50-75 | 100 | 4 equal twitches | Normal strength and movement, cough strength decreased; double burst suppression abnormal |
<30 | 100 | 4 equal twitches | No apparent deficits, double burst suppression normal |
NIP, Negative inspiratory pressure; PTF, posttetanic facilitation.
TOF monitoring allows for an accurate assessment of neuromuscular blockade depth with or without baseline control. To avoid overdosing of patients, the NMBA (bolus or infusion) should be titrated to produce the minimal blockade required to maintain the desired clinical response. Predefined goals, such as decreased oxygen requirements, peak inspiratory pressure, and positive end-expiratory pressure (PEEP) reduction, should be assessed frequently. If the response is adequate, a TOF count of at least 1-2/4 (number of twitches to stimulations) is recommended. It is possible that lesser degrees of blockade may achieve the clinical goal of ventilator synchrony or improved oxygenation. In the ICU, the depth of blockade should be assessed every 2 to 3 hours on initiation until a stable dose is maintained. Thereafter, TOF assessment may occur every 8 to 12 hours. If there is no twitch response or the clinical response is achieved at a higher twitch, the dose of the NMBA should be decreased by 10%. If three or four twitches occur without adequate response, the dose can be increased by 10%. The need for continued paralysis of a patient in the ICU should be assessed daily, and, if appropriate, paralysis should be discontinued as soon as possible.7
Future of neuromuscular blocking agents and reversal
Research is continuing in an effort to develop an ideal NMBA. At the present time, gantacurium (GW280430A) has promise as a new agent. Still in the research phase, gantacurium is a nondepolarizing agent with rapid-onset and short-acting properties. Its organ-independent inactivation is ideal in patients with organ dysfunction. Histamine release has been found to occur, resulting in tachycardia and hypotension. The histamine release seems to be less compared with the histamine release produced by the benzylisoquinolines.11 Although gantacurium offers a promising alternative to currently available NMBAs, it may have drawbacks that have not yet been discovered and requires further evaluation in larger trials.
At present, the method of reversing NMBAs involves the use of AchE inhibitors such as neostigmine, which increase the levels of Ach in the synaptic cleft to compete with NMBA for receptor sites. Sugammadex, an agent currently under study, provides a newer approach to NMBA reversal. The novel mechanism of action involves the actual inactivation and removal of the NMBA from the neuromuscular junction and the body. Sugammadex functions by encapsulating the NMBA to form a complex that can no longer bind to the receptors. The stable complex that is formed is excreted by the kidneys. Sugammadex has been shown to reverse only rocuronium and vecuronium effectively. It is much less effective for reversal of pancuronium, succinylcholine, and the benzylisoquinolines. Adverse effects are mild and include nausea, dry mouth, cough, and taste perversions.12