Chapter 2 Routine Clinical Electromyography
NERVE CONDUCTION STUDIES
There are three types of NCS that are used in clinical practice: motor, sensory, and mixed NCS. The motor fibers are assessed indirectly by stimulating a nerve while recording from a muscle and analyzing the evoked compound muscle action potential (CMAP), also referred to as the motor response or the M wave (M for motor). The sensory fibers are evaluated by stimulating and recording from a nerve and studying the evoked sensory nerve action potential (SNAP), also referred to as the sensory response. Mixed NCSs are less commonly used and assess directly the sensory and motor fibers in combination by stimulating and recording from a mixed nerve and analyzing the evoked mixed nerve action potential (MNAP).
Stimulation Principles and Techniques
Supramaximal stimulation of nerves that results in depolarization of all the available axons is a paramount prerequisite to all NCS measurements. To achieve supramaximal stimulation, current (or voltage) intensity is slowly increased until it reaches a level where the recorded potential is at its maximum. Then, the current should be increased an additional 20–30% to ensure that the potential does not increase in size further (Figure 2-1). Stimulation via a needle electrode deeply inserted near a nerve is used less often in clinical practice. This is usually reserved for circumstances where surface stimulation is not possible, such as in deep-seated nerves (e.g., sciatic nerve or cervical root stimulation).
Recording Electrodes and Techniques
Surface electrodes are most often used for nerve conduction recordings. Surface recording electrodes are often made as small discs that are placed over the belly of the muscle or the nerve (Figure 2-2). The advantages of surface recording are that the evoked response is reproducible and changes only slightly with the position of the recording electrode. Also, the size (amplitude and area) of the response is a semiquantitative measure of the number of axons conducting between the stimulating and recording electrodes.
With motor conduction studies, the active recording electrode is placed over the belly of the muscle that correlates with the endplate zone. This ensures that muscle activity at the moment of depolarization is recorded as soon as the nerve action potential has arrived at the endplate. Ring electrodes are convenient to record the antidromic sensory potentials from hand digital nerves over the proximal and distal interphalangeal joints (Figure 2-3). These ring electrodes could act as stimulation points with orthodromic recording from hand digits.
Needle recording is also possible but is less popular and reserved for situations where the recording sites are deep-seated muscles or nerves. Needle recordings are also useful to improve the recording from small atrophic muscles or a proximal muscle not excitable in isolation. In contrast to surface recording, needle electrode recording registers only a small portion of the muscle or nerve action potentials and the amplitude of the evoked response is extremely variable and highly dependent on the exact location of the needle. Hence, amplitude and area measurement are not reproducible which renders this technique not clinically valuable such as in assessing conduction block or estimating the extent of axonal loss (see below).
Sensory Nerve Conduction Studies
Sensory NCSs are performed by stimulating a nerve while recording the transmitted potential from the same nerve at a different site. Hence, SNAPs are true nerve action potentials. Antidromic sensory NCSs are performed by recording potentials directed toward the sensory receptors while orthodromic studies are obtained by recording potentials directed away from these receptors. Sensory latencies and conduction velocities are identical with either method, but SNAP amplitudes are higher in antidromic studies and, hence, more easily obtained without the need for averaging techniques. Since the thresholds of some motor axons are similar to those of large myelinated sensory axons, superimposition of muscle action potentials may obscure the recorded antidromic SNAPs. These volume-conducted muscle potentials often occur with mixed nerve stimulation or may result from direct muscle co-stimulations. Fortunately, SNAPs can still be measured accurately in most cases because the large-diameter sensory fibers conduct 5–10% faster than motor fibers. This relationship may change in disease states that selectively affect different fibers. In contrast to the antidromic studies, the orthodromic responses are small in amplitude, more difficult to obtain, and might require averaging techniques (Figure 2-4).
SNAPs may be obtained by (1) stimulating and recording a pure sensory nerve (such as the sural and radial sensory responses), (2) stimulating a mixed nerve while recording distally over a cutaneous branch (such as the antidromic median and ulnar sensory responses), or (3) stimulating a distal cutaneous branch while recording over a proximal mixed nerve (such as the orthodromic median and ulnar sensory studies). The active recording electrode (G1) is placed over the nerve and the reference electrode (G2) is positioned slightly more distal with antidromic recordings or slightly more proximal with orthodromic techniques. The distance between G1 and G2 electrodes should be fixed (usually at about 3–4 cm), since it has a significant effect on SNAP amplitude. The SNAP is usually triphasic with an initial small positive phase, followed by a large negative phase and a positive phase. Several measurements may be recorded with sensory NCSs (Figure 2-5):
Motor Nerve Conduction Studies
A belly-tendon recording is a typical electrode placement to obtain a CMAP: a pair of recording electrodes are used with an active lead (G1) placed on the belly of the muscle and a reference lead (G2) on the tendon (see Figure 2-2). Both active and reference electrodes locations are an essential determinant of the CMAP size, shape, and latency. The propagating muscle action potential, originating near the motor point and under G1, gives rise to a simple biphasic waveform with an initial large negative phase followed by a smaller positive phase. With incorrect positioning of the active electrode away from the endplate, the CMAP will show an initial positive phase that corresponds to the approaching electrical field of the impulses from muscle fibers toward the electrode. Similar initial positivity is also recorded with a volume-conducted potential from distant muscles activated by anomalous innervation or by accidental spread of stimulation to other nerves.
Whenever possible, the nerve is stimulated at two or more points along its course. Typically, it is stimulated distally near the recording electrode and more proximally to evaluate its proximal segment. Several measurements are evaluated with motor NCSs (Figure 2-6):
Mixed Nerve Conduction Studies
Mixed NCSs are done by stimulating and recording from nerve trunks with sensory and motor axons. Often, these tests are done by stimulating a nerve trunk distally and recording more proximally, since the reverse is often contaminated by large CMAP that obscures the relatively low-amplitude MNAPs. In situations where the nerve is deep (such as the elbow or knee), the MNAP may be very low in amplitude or unelicitable, due to considerable tissue interposing between the nerve and recording electrode. Hence, these studies are not popular in clinical practice and are restricted to evaluating mixed nerves in distal nerve segments, such as in the hand or foot during the evaluation of carpal tunnel syndrome and tarsal tunnel syndrome, respectively (Figure 2-7).
Physiologic Variabilities
Temperature. Nerve impulses propagate slower by 2.4 m/s or approximately 5% per degree Celsius as the limb cools from 38 to 29°C. Also, cooling results in a higher CMAP and SNAP amplitude and longer duration probably because of accelerated and slowed Na+ channel inactivation. Hence, a CMAP or SNAP with high amplitude and slow distal latency or conduction velocity should be highly suspicious of a cool limb (Figure 2-8).
Age. Nerve conduction velocities are slow at birth since myelination is incomplete. They are roughly one-half the adult value in full-term newborns and one-third that of term newborns in 23- to 24-week premature newborns. They reach adult values at 3–5 years. Then, motor and sensory nerve conduction velocities tend to slightly increase in the arms and decrease in the legs during childhood up to 19 years. With aging, conduction velocities slowly decline after 30–40 years of age, that the mean conduction velocity is reduced about 10% at 60 years of age.
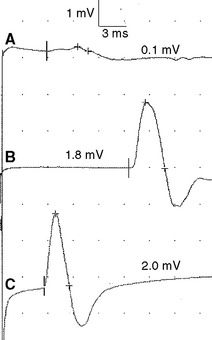
Figure 2-13 Prominent accessory deep peroneal nerve anomaly. Note here that the distal CMAP was extremely low in amplitude (A) while the proximal CMAP is higher (B). Similar to Figure 2-12, stimulation behind the lateral malleolus yielded a relatively large CMAP (C). However, in this example, most fibers were directed to the extensor digitorum brevis through the accessory deep peroneal nerve, leaving only a few to travel through the main trunk of the deep peroneal nerve.
Physiological temporal dispersion affects the SNAP more than the CMAP. This is related to two factors. First is the disparity between sensory fiber and motor fiber conduction velocities. The range of conduction velocities between the fastest and slowest individual human myelinated sensory axons is almost double that of the motor axons (25 m/s versus 12 m/s). This results in more dispersion of individual action potentials and leads to more prominent phase cancellation. The second factor is the difference in duration of individual unit discharges between nerve and muscle. With short-duration biphasic sensory spikes, a slight latency difference could line up the positive peaks of the fast fibers with the negative peaks of the slow fibers and cancel both. In longer duration motor unit potentials, the same latency shift would only partially superimpose peaks of opposite polarity, and cancellation would be less of a factor (Figures 2-14 and 2-15).
Intertrial variability. Principal factors contributing to an intertrial variability include errors in determining surface distance and in measuring latencies and amplitudes of the recorded response. A slight shift in recording site results in significant amplitude variability. NCSs are more reproducible when done by the same examiner, because of the significant degree of inter-examiner variability.
Common Sources of Error
Recording or Reference Electrode Misplacement
With the belly-tendon method of recording, the CMAP shows an initial positive deflection if the active (G1) recording electrode is not placed over the endplate. This occurs since the volume-conducted depolarization potential first occurs at a distance from the recording electrode. This electrode misplacement results in error in measuring the latency and the CMAP amplitude may be reduced.
NEEDLE ELECTROMYOGRAPHIC EXAMINATION
Motor Units and Muscle Fibers
Muscle fibers are classified based on their mechanical properties and resistance to fatigue. Based on the speed of the actin–myosin reaction and the Ca2+-dependent activation and relaxation regulatory systems, muscle fibers are either slow or fast. They are also either fatigue-resistant with higher mitochondrial content, or fatigable. Hence, muscle fibers are usually labeled as type I (slow and fatigue-resistant), type II A (fast and fatigue-resistant), or type II B (fast and fatigable) fibers (Table 2-1). All muscle fibers of each individual motor unit are of one specific type. The distribution of muscle fibers of a single motor unit within a muscle is wide with considerable overlap among the territories of motor units.
Principles
The skeletal muscle fiber has a resting potential of 90 mV, with negativity inside the cell. These fibers, as well as neurons and other excitable cells, generate action potentials when the potential difference across the plasma membrane is depolarized past a specific threshold. This follows an “all-or-none” rule, which means that increasing the stimulus does not change the shape of the action potential. The generation of an action potential reverses the transmembrane potential, which then becomes positive inside the cell. An extracellular electrode, as used in needle EMG, records the activity resulting from this switch of polarity as a predominantly negative potential (usually triphasic, positive–negative–positive waveforms). However, when recording near a damaged region, action potentials consist of a large positivity followed by a small negativity.
Concentric and Teflon-coated monopolar needle electrodes are equally satisfactory in recording muscle potentials, with few appreciable differences (Table 2-2). Though monopolar needles are less painful, they require an additional reference electrode nearby which often results in greater electrical noise due to electrode impedance mismatch between the intramuscular active electrode and the surface reference disk.
Table 2-2 Difference Between Monopolar and Concentric Needle Electrodes
Concentric | Monopolar |
---|---|
Does not requires an independent reference electrode | Requires an independent reference electrode |
More painful | Less painful |
More expensive | Less expensive |
Low baseline noise | High baseline noise |
Lower MUAP amplitude | Higher MUAP amplitude |
Sharper MUAP rise time | MUAP rise time not as sharp |
Shorter MUAP duration | Longer MUAP duration |
Techniques
Insertional and Spontaneous Activity
Normal Insertional and Spontaneous Activity
Endplate spikes. These are intermittent spikes and represent discharges of individual muscle fibers generated by activation of intramuscular nerve terminals irritated by the needle. Their characteristic irregular firing pattern distinguishes them from the regular-firing fibrillation potentials (Figure 2-16). The waveform of endplate spike is also distinguished by its initial negative deflection since the generator of the potential is usually underneath the needle’s tip. Endplate spikes fire irregularly at 5–50 impulses per second, and measures 100–200 μV in amplitude, and 3–4 ms in duration. They have a cracking sound on the loudspeaker, imitating “sputtering fat in a frying pan.”
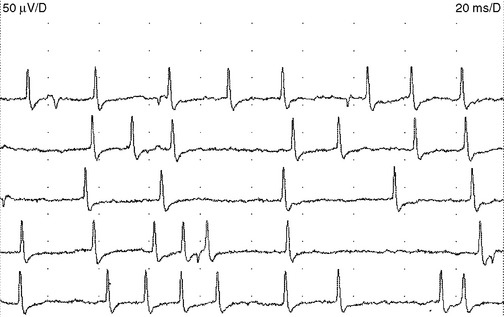
Figure 2-16 Endplate spikes. Note the irregular firing pattern and the biphasic morphology with an initial negative deflection that separates this from the brief spike form of fibrillation potentials. (Compare the waveforms in this figure with those in Figure 2-18.)
Endplate noise. The tip of the needle approaching the endplate region frequently registers recurring irregular negative potentials, 10–50 μV in amplitude and 1–2 ms in duration (Figure 2-17). These potentials are the extracellularly recorded miniature endplate potentials that, in turn, are nonpropagating depolarizations caused by spontaneous release of acetylcholine quanta. Endplate potentials produce a characteristic sound on loudspeaker much like a “seashell held to the ear.”
Abnormal Insertional Activity
Decreased insertional activity. A reduction or absence of insertional muscle activity suggests either fibrotic or severely atrophied muscles. Rarely, this represents functionally inexcitable muscles such as during the acute attacks of periodic paralysis.
Abnormal Spontaneous Activity
Fibrillation potentials are seen following muscle denervation that occurs with motor axon loss lesions to the anterior horn cells of the spinal cord, root, plexus, or peripheral nerve. Fibrillation potentials appear after 1–2 weeks of acute denervation but do not become full till after 3 weeks after nerve injury. They disappear late in the course of denervation when muscle fibers become reinnervated or fibrotic and severely atrophied. Hence, fibrillation potentials may be absent in very acute or chronic denervation.
Fasciculation potentials are encountered most commonly in motor neuron diseases, but are seen also in radiculopathies, entrapment neuropathies, peripheral polyneuropathies, and the cramp-fasciculation syndrome. They are seen also in tetany, thyrotoxicosis, and overdose of anticholinesterase medication. In addition, they may occur in healthy individuals, and there is no reliable method of distinguishing “benign” from “malignant” fasciculation potentials except that the fasciculation potentials in motor neuron disease tend to fire slower, are more complex, and less stable. Most importantly, benign fasciculation potentials are not associated clinically with weakness and wasting, or with other electrophysiologic signs of denervation including fibrillation potentials and neurogenic MUAP changes (Figure 2-20).
Complex repetitive discharges. A complex repetitive discharge is often referred to as CRD and was formerly known as bizarre repetitive discharge. It is a composite waveform that contains several distinct spikes and often fires at a constant and fast rate of 30 to 50 Hz. Occasionally, the discharge frequency is slow or extremely fast, ranging from 5 to 100 Hz. The individual CRD ranges from 50 μV to 1 mV in amplitude and up to 50–100 ms in duration. It remains uniform from one discharge to another, a feature that helps distinguishing it from myokymic discharge (Figure 2-21). CRDs typically begin and cease abruptly. On loudspeaker, CRD produces a noise that mimics the sound of a “machine.” Pathophysiologically, CRD results from the near synchronous firing of a group of muscle fibers that communicates ephaptically. One fiber in the complex serves as a pacemaker, driving one or several other fibers so that the individual spikes within the complex fire in the same order as the discharge recurs. One of the late-activated fibers re-excites the principal pacemaker to repeat the cycle. The chain reaction eventually blocks resulting in abrupt cessation. CRDs are abnormal discharges but rather nonspecific since they accompany a variety of chronic neurogenic as well myopathic disorders. They may also be found in the iliopsoas or cervical parapsinal muscles of apparently healthy individuals, probably implying a clinically silent neuropathic process.
Myokymic discharges. Myokymic discharge is defined as groups of motor unit potentials that fire repetitively in a quasi-rhythmical fashion with intervening period of silence. The burst composed of about 2–15 spikes with frequent variability in the number of spikes per discharge (Figure 2-22). The intraburst frequency is about 30–40 Hz, while the interburst frequency is much slower and ranges from 1 to 5 Hz, which gives myokymia the sound of “marching soldiers” on the loudspeaker. Clinically, myokymic discharges often give rise to sustained muscle contractions, which have an undulating appearance beneath the skin (bag of worms). Myokymic discharges probably originate ectopically in motor fibers and decrease in intensity with progressively distal nerve blocks. They may be amplified by increased axonal excitability, such as after hyperventilation-induced hypocapnia.
Myokymic discharges may be restricted to focal areas such as the in face with brainstem glioma or multiple sclerosis, a single extremity with radiation plexopathy, or the thenar eminence with carpal tunnel syndrome. They also may be generalized as encountered in association with gold toxicity or the syndrome of continuous motor unit activity (Isaac syndrome) (Table 2-3).
Focal | ||
---|---|---|
Facial | Limb | Generalized |
* CIDP = chronic inflammatory demyelinating polyneuropathy.
† HNPP = hereditary neuropathy with liability to pressure palsy (tomaculous neuropathy).
Neuromyotonic discharges. Neuromyotonic discharges are extremely rare discharges in which motor units fire repetitively at high frequency (150–250 Hz), either continuously or in recurring decrementing bursts, producing a “pinging sound” on loudspeaker (Figure 2-23). The discharge continues during sleep, and diminishes in intensity with progressively distal nerve blocks, implicating the entire axon as the site of generation. The syndrome of continuous motor unit activity (Isaac syndrome) which may have an autoimmune etiology, with the target antigen likely being peripheral nerve potassium channels, is often associated with neuromyotonia and myokymia. Other conditions associated with neuromyotonia include anticholinesterase poisoning, tetany, and chronic spinal muscular atrophies.
Myotonic discharges. Like fibrillation potentials, myotonic discharges appear either as a sustained run of sharp positive waves or brief spikes (Figure 2-24). Positive sharp waves are initiated by needle insertion injuring muscle membrane, whereas the brief spikes tend to occur at the beginning of slight volitional contraction. Both types of discharges typically wax and wane in amplitude (range = 10 μV–1 mV), and frequency (range = 20–150 Hz), which gives rise to a characteristic noise over the loudspeaker, simulating a “dive-bomber” or an “accelerating–decelerating motorcycle or chain saw.”
Myotonic discharges may occur with or without clinical myotonia in the myotonic dystrophies, myotonia congenital, and paramyotonia congenita. They may also accompany acid maltase deficiency, colchicine myopathy, myotubular myopathy, and hyperkalemic periodic paralysis (Table 2-4).
Table 2-4 Common Causes of Electrical Myotonia
Voluntary Motor Unit Action Potentials
Motor Unit Action Potential Morphology
The motor unit action potential (MUAP) is the sum of the extracellular potentials of muscle fiber action potentials of a motor unit. The waveform is dictated by the inherent properties of the motor unit and the spatial relationships between the needle and individual muscle fibers. The extracellularly recorded MUAP, recorded along the length of the muscle fibers and away from the endplate region, has a triphasic waveform (Figure 2-25). The initial positive deflection represents the action potential propagating towards the electrode. As the potential passes in front of the electrode the main positive–negative deflection is recorded. When the action potential propagates away from the electrode the potential returns to the baseline. Slight repositioning of the electrode causes major changes in the electrical profile of the same motor unit. Therefore, one motor unit can give rise to MUAPs of different morphology at different recording sites. If the electrode is placed immediately over the endplate area, the initial positive defection will not be recorded and the potential will have a biphasic waveform with an initial negative deflection.
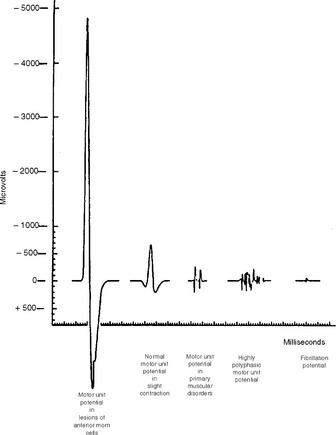
Figure 2-25 Relative average durations and amplitudes of some MUAPs seen in myopathic and neurogenic disorders.
(Reproduced from Daube J. Needle electromyography in clinical electromyography. Muscle Nerve 1991;14:685–700, with permission.)
Amplitude. MUAP amplitude is the maximum peak-to-peak amplitude and ranges from several hundred microvolts to a few millivolts with a concentric needle, and is substantially greater with a monopolar needle. At a short distance between the recording electrode and the potential generators (muscle fibers), the MUAP has a short rise time and high amplitude with a “crisp” or “sharp” sound on the loudspeaker. In contrast, the MUAP recorded from distant muscle fibers has a long rise time and a low amplitude that sounds “dull” or “muffled” on the loudspeaker. For example, the MUAP amplitude decreases to less than 50% at a distance of 200–300 μm from the source and to less than 1% a few millimeters away. Therefore, only a small number of individual muscle fibers located near the tip of the recording electrode determine the amplitude of an MUAP (probably less than 20 muscle fibers lying within a 1 mm radius of the electrode tip). In general, amplitude indicates muscle fiber density and not the motor unit territory. High MUAP amplitude, when isolated, is considered a nonspecific abnormality except when it is significantly increased (more than twice the upper normal limit); then, it indicates a neurogenic process.
Long-duration MUAPs are the best indicators of reinnervation. They occur with increased number or density of muscle fibers, or a loss of synchrony of fiber firing within a motor unit as seen with lower motor neuron disorders. These MUAPs may also show high amplitude (see Figure 2-25). In contrast, short-duration MUAPs often have low amplitude and are indicators of muscle fiber loss as seen with necrotizing myopathies.
Motor Unit Action Potential stability
Motor units normally discharge semirhythmically, with successive potentials showing nearly identical configuration due to firing of all muscle fibers of the motor unit during every discharge. The morphology of a repetitively firing unit may fluctuate if individual muscle fibers intermittently block within the unit. This instability may be evident in neuromuscular junction disorder, such as myasthenia gravis, the myasthenic syndrome, or botulism. Also during reinnervation such as motor neuron disease, subacute radiculopathy, or polyneuropathy, the newly formed endplates are immature and demonstrate poor efficacy of neuromuscular transmission. This results in unstable MUAP waveforms with moment-to-moment MUAP variability (Figure 2-26). The MUAP instability disappears when reinnervation is complete and well established and helps to distinguish between a subacute and chronic neurogenic process.
Motor Unit Action Potential Firing Patterns
The firing rate of the motor unit equal to the number of MU discharges in a one second time interval, and is measured in hertz (Hz). When several MUAPs are discharging they superimpose, which makes MUAP identification and firing rate analysis difficult requiring automated methods. When one or two MUAPs are firing, such as during minimal voluntary effort or when there is marked decrease in the number of MUAPs firing, this analysis become quite easy. The firing rate may be estimated manually by freezing a 100 ms epoch and multiplying the number of discharges of an MUAP by 10 to obtain a one second epoch. For example, a motor unit appearing twice in a 100 ms sweep has a firing rate of 2 × 10 = 20 Hz. The multiplication factor can be adjusted depending on the analyzed epoch, being 5 for a 200 ms epoch and 2 for a 500 ms epoch (Figure 2-27). Another way of calculating the firing rate of a motor unit is by dividing 1000 by the time interval between successive MUAP discharges in ms. For example, a firing rate of a unit with an interval of 50 ms is 20 Hz.
Activation is the central control of motor units that allows an increase in the firing rate and force. Failure of descending impulses also limits recruitment, although here the excited motor units discharge more slowly than expected for normal maximal contraction. Thus, a decreased number of voluntary MUAPs with a slow firing rate (poor activation) is a feature of an upper motor neuron disorder (such as stroke or myelopathy) but may be seen with volitional lack of effort (such as due to pain, conversion reaction, or malingering). This stands in sharp contrast to a fast firing rate associated with a disorder of the lower motor neuron (decreased recruitment).
Chaudhry V, Cornblath DR, Mellits ED, et al. Inter- and intra-examiner reliability of nerve conduction measurements in normal subjects. Ann Neurol. 1991;30:841-843.
Daube J. Needle electromyography in clinical electromyography. Muscle Nerve. 1991;14:685-700.
Erim Z, de Luca CJ, Mineo K, et al. Rank-ordered regulation of motor units. Muscle Nerve. 1996;19:563-573.
Gutmann L. Important anomalous innervations of the extremities. Muscle Nerve. 1993;36:899-990.
Hammer K. Nerve conduction studies. Springfield, IL: C Thomas Publishers, 1982.
Harik SI, Baraka AS, Tomeh GF, et al. Autonomous peripheral nerve activity causing generalized muscle stiffness and fasciculations: report of a case with physiological, pharmacological, and morphological observations. Johns Hopkins Med J. 1976;139(S):49-60.
Hart IK, Waters C, Vincent A, et al. Autoantibodies detected to expressed K+ channels are implicated in neuromyotonia. Ann Neurol. 1997;41:238-246.
Iyer V, Fenichel GM. Normal median nerve proximal latency in carpal tunnel syndrome: a clue to coexisting Martin-Gruber anastomosis. J Neurol Neurosurg Psychiatry. 1976;39:449-452.
Katirji B. Clinical electromyography. Neurology clinics. Philadelphia, PA: WB Saunders, 2002.
Katirji B. Clinical electromyography. In: Bradley WG, Daroff RB, Fenichel GM, Jankovic J, editors. Neurology in clinical practice. 4th ed. Boston, MA: Butterworth-Heinemann; 2004:491-520.
Kimura J. Collision technique. Physiologic block of nerve impulses in studies of motor nerve conduction velocity. Neurology. 1976;26:680-682.
Kimura J. The carpal tunnel syndrome: localization of conduction abnormalities within the distal segment of the median nerve. Brain. 1979;102:619-635.
Kimura J. Facts, fallacies, and facies of nerve conduction studies: twenty-first annual Edward H. Lambert lecture. Muscle Nerve. 1997;20:777-787.
Lambert EH. The accessory deep peroneal nerve: a common variation in innervation of extensor digitorum brevis. Neurology. 1969;19:1169-1176.
McDonald WI. The physiological consequences of demyelination. In: Sumner AJ, editor. The physiology of peripheral nerve disease. Philadelphia, PA: WB Saunders; 1980:265-286.
McIntosh KA, Preston DC, Logigian EL. Short segment incremental studies to localize ulnar entrapments at the wrist. Neurology. 1998;50:303-306.
Petajan JH. Motor unit recruitment. Muscle Nerve. 1991;14:489-502.
Preston DC, Shapiro BE. Needle electromyography. Fundamentals, normal and abnormal patterns. Neurol Clin N Am. 2002;20:361-396.
Rutkove SB, Kothari MJ, Shefner JM. Nerve, muscle, and neuromuscular junction electrophysiology at high temperature. Muscle Nerve. 1997;20:431-436.
Sander HW, Quinto C, Chokroverty S. Median-ulnar anastomosis to thenar, hypothenar, and first dorsal interosseous muscles: collision technique confirmation. Muscle Nerve. 1997;20:1460-1462.
Shapiro BE, Katirji B, Preston DC. Clinical electromyography. In: Katirji B, Kaminski HJ, Preston DC, Ruff RL, Shapiro EB, editors. Neuromuscular disorders in clinical practice. Boston, MA: Butterworth-Heinemann, 2002.
St lberg E, Antoni L. Electrophysiological cross section of the motor unit. J Neurol Neurosurg Psychiatry. 1980;43:469-474.
Wilbourn AJ. Nerve conduction studies. Types, components, abnormalities and value in localization. Neurol Clin. 2002;20:305-338.