CHAPTER 18 RESUSCITATION FLUIDS
The practice of giving intravenous fluids or medication has been used since the 1600s. Slow progress was made after William Harvey provided a modern description of the circulatory system in 1638. William O’Shaughnessy theorized that patients suffering from volume loss secondary to cholera would benefit from restoration of blood to its natural specific gravity. This became the first concept of contemporary intravenous fluid therapy. Thomas Latta was credited with actually applying O’Shaughnessy’s theory and treating victims of cholera in 1832. Later in the century, Sydney Ringer described a physiologic solution with a focus on electrolyte concentrations in his animal models. Despite these early trials, application of these concepts was criticized and largely forgotten until the 20th century. The advent of modern surgery renewed interest in resuscitation as therapy to maintain intravascular volume.
CLASSES OF SHOCK
Shock is inadequate tissue perfusion. The humoral sympathetic response to shock causes vasoconstriction in the clinical appearance of the patient. These findings were observed by Cannon when he correlated the volume of blood loss with the patient’s clinical signs, symptoms, and blood pressure. His findings have since been validated with repeated observational studies in humans, as well as experimental studies in animals. The classes of shock are now well defined, and provide a quick means of assessing a patient’s blood loss and the level of resuscitation required (Table 1).
AUTORESUSCITATION
Vasoconstriction and Reduction of Capillary Hydrostatic Pressure
The subsequent decrease in capillary hydrostatic pressure reduces the volume of plasma lost from the vasculature into the interstitial space, and increases return of interstitial fluid into the vascular space. This is an additional method of increasing venous return to the heart. Given the large capacitance of the venous system, larger volumes of circulating blood are stored within the veins compared to the arteries. According to the Frank-Starling laws of cardiac performance, the cardiac output is directly proportional to the venous return to the heart (preload) (Figure 1). As venous return decreases, so does cardiac output, and thereby arterial pressure to perfuse vital organs.
Hormonal Response
The reduction of capillary hydrostatic pressure and the hormonal response increase venous return during the first 1–2 hours following injury, but this may be inconsequential in the setting of severe hemorrhage. Cessation or slowing of ongoing hemorrhage also contributes to a spontaneous rise in blood pressure by limiting ongoing fluid losses. The remainder of the response is carried out through vasoconstriction and the redistribution of blood flow, accounting for the majority of increased venous return. If prolonged, this can have a damaging, and sometimes irreversible effect upon the tissues supplied by the splanchnic circulation. This will result in ischemia and potential reperfusion injury.
Phases of Shock
Combined, the aforementioned physiologic effects produce the classic signs and symptoms exhibited by patients in hypovolemic shock. The progression from compensated to uncompensated shock gives rise to the physical findings of the systemic insult (Figure 2). Initially, compensated shock affects the periphery, manifesting the symptoms of cool extremities, cyanosis, mottling of the skin, and decreased capillary reperfusion (blanching). As the severity of shock progresses, vasoconstriction affects the torso, producing oliguria as the main clinical sign. Finally, in severe classes of shock, perfusion of the heart and brain are affected, producing cardiac dysrhythmias and a reduced level of consciousness. Over the hours following injury, these mechanisms are in constant flux, in efforts to restore homeostasis to the injured system.
HYPOTENSIVE RESUSCITATION
Choice of Fluids
The use of crystalloids versus colloids has been an ongoing debate for decades. In the Vietnam War, isotonic crystalloids were used when laboratory work from the 1960s by Shires and others showed larger volume resuscitation with isotonic crystalloids resulted in the best survival. They also noted that extracellular fluid redistributed into both intravascular and intracellular spaces during shock, and rapid correction of this extracellular deficit required an infusion of a 3:1 ratio of crystalloid fluid to blood loss. Figure 3 shows the influence of fluids on the extracellular fluid compartments. Using this resuscitation strategy, the rate of mortality and acute renal failure decreased but a new entity of shock lung, now better known as acute respiratory distress syndrome (ARDS), was discovered. In reviewing studies comparing crystalloids and colloids with respect to pleural effusions and pulmonary dysfunction, two trials reported no differences. Two other series showed more pulmonary complications among patients resuscitated with colloid. When mortality was used as an endpoint, the use of crystalloids in trauma patients was associated with increased survival.
Crystalloid Solutions
The choice of lactated Ringer’s (LR) and normal saline (NS) appears to be less controversial. Both are hypo-oncotic, balanced salt solutions (Table 2). It is well known that large volumes of NS resuscitation can lead to hyperchloremic metabolic acidosis. While large volumes of LR infused rapidly can increase lactate levels, this is not associated with acidosis. Koustova et al. have shown that LR influences neutrophil function by increasing production of reactive oxygen species and affects leukocyte gene expression, but Watters et al. showed that NS and LR have equivalent effects on alveolar neutrophils in a swine model of hemorrhagic shock. Healy et al. showed, in a rat model of massive hemorrhage and resuscitation, animals were significantly more acidotic (pH 7.14 ± 0.06 vs. 7.39 ± 0.04) and had a significantly worse survival (50% vs. 100%) when resuscitated with NS compared to LR. NS has been theoretically preferred when giving blood because of the concern that the calcium in LR could exceed the chelating capabilities of the citrate, resulting in clots that may enter the microcirculation. However, Lorenzo et al. showed that when fluid is given rapidly, LR does not increase clots, and at present there is no evidence that this is a clinically significant issue. In theory, NS is favored over LR for the treatment of severe head injuries due to its higher sodium content, which would reduce intracerebral swelling. Despite this concern, there is no literature supporting the use of NS over LR in this setting.
Blood Transfusions
Early clinical studies concluded that hemoglobin (HgB) levels of 10 g/dl were optimal for shock resuscitation; however, recent consensus panels have suggested that a lower concentration is adequate. A prospective randomized trial showed that ICU patients who received blood transfusion for HgB less than 7.0 g/dl and were maintained at HgB 7.0–9.0 g/dl did as well and possibly better than patients who were liberally transfused for a HgB level less than 10 g/dl and maintained at 10–12 g/dl. All these patients were without ongoing bleeding, acute myocardial infarction, or unstable angina. Subgroup analysis looking at the safety of a restricted red blood cell transfusion strategy in trauma patients showed there was no statistically significant difference in mortality, multiple organ dysfunction, or length of stay, when compared to those managed with the liberal transfusion strategy. Randomized, controlled investigations need to be conducted to provide evidence to help dictate the use of blood in critically ill trauma patients.
COMPLICATIONS OF RESUSCITATION
The focus in resuscitation has shifted to large-volume fluid resuscitation in acutely injured patients. With severe hemorrhagic shock and uncontrolled hemorrhage, interventions can commonly cause iatrogenic complications. Continued large volume crystalloid resuscitation in the setting of ongoing bleeding inevitably leads to acidosis, hypothermia, and coagulopathy. Individually, each of these conditions can worsen the other (Figure 4).
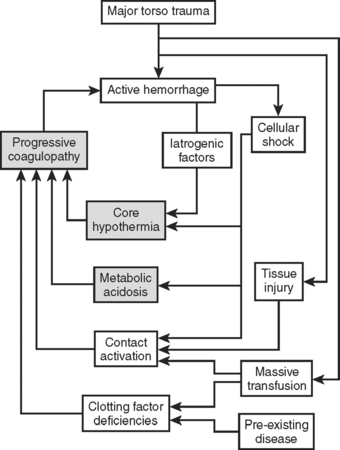
Figure 4 The lethal triad.
(Adapted from Moore EE: Staged laparotomy for the hypothermia, acidosis, coagulopathy syndrome. Am J Surg 172:405–410, 1996, with permission of publisher.)
Hypothermia
Because of the strong association of hypothermia to mortality and the other elements of the “lethal triad,” rewarming and prevention of ongoing heat loss should be a priority of resuscitation (Table 3). The first step is to obtain an accurate core temperature. Esophageal or bladder temperatures have been shown to be more reliable than rectal or axillary measurements. Given the high rate of heat loss with infusion of room temperature fluids, all resuscitation solutions should be warmed. Operating room temperatures should be elevated to minimize losses due to conduction and radiation. Once the secondary evaluation is complete, the patient should be covered. A Bair Hugger or similar device can be used to actively warm the patient. For profound hypothermia, active internal rewarming can be used. This is done either with lavage of a body cavity (peritoneal or thoracic) or by intravascular rewarming. Options for intravascular rewarming include veno-venous rewarming, arteriovenous rewarming, or cardiopulmonary bypass. Continuous arteriovenous rewarming (Figure 5) has been shown to decrease early mortality in critically injured hypothermic trauma patients.
Table 3 Approximate Rate of Heat Transfer with Available Rewarming Methods
Rewarming Technique | Rate of Heat Transfer |
---|---|
Airway rewarming | 33.5–50.3 |
Overhead radiant warmer | 71.2 |
Heating blankets | 83.8 |
Convective warmers | 62.8–108.9 |
Body cavity lavage | 150.8 |
Continuous arteriovenous rewarming | 385.5–582.4 |
Cardiopulmonary bypass | 2974.9 |
Source: Adapted from Gentilello LM: Practical approaches to hypothermia. In Maull KI, Cleveland HC, Feliciano DV, Rice CL, Trunkey DD, Wolferth CC, editors: Advances in Trauma and Critical Care, vol. 9. St. Louis, MO, Mosby, 1994, pp. 39–79, with permission.
Coagulopathy
Hypothermia has a profound effect on coagulation activity. Reed and Rohrer in different experiments showed that hypothermia resulted in prolonged partial thromboplastin time (PTT) and prothrombin time (PT) independent of the actual level of enzymes. Gubler et al. showed that the effect of a dilutional coagulopathy is additive to this hypothermic effect (Figure 6). In addition to these enzyme effects, fibrinolysis is thought to be enhanced by hypothermia. Animal studies have suggested that platelets are sequestered by the spleen in the setting of hypothermia. Platelet adhesion is also decreased in hypothermic patients. A recent in vitro study suggests that platelet effects cause the majority of hypothermic coagulopathy at temperatures above 33° C. In this trial, enzymatic dysfunction did not have a significant effect until the temperature was below 33° C. Studies have shown that acidotic and hypothermic patients with adequate blood, plasma, and platelet replacement still develop clinically significant bleeding.
As with any disease, treatment of coagulopathy begins with recognizing the problem. Any trauma patient with evidence of significant tissue injury or ongoing bleeding should be screened with an (INR), PTT, platelet count, and fibrinogen level. In the setting of active traumatic bleeding, the platelet count should be kept above 50,000/mcl, the INR is less than 2, the fibrinogen greater than 100 mg/dl, and the PTT less than 1.5 times normal. Again, close monitoring of these parameters is recommended as empiric therapy often yields unpredictable results. In the absence of laboratory results, empiric therapy with platelets that contains plasma may be started.
Acidosis
Compartment Syndromes
Bladder pressures should be monitored in patients showing clinical signs of ACS or patients in shock who are receiving large resuscitations. A tense abdomen in the setting of low urine output, high airway pressures, and hypotension are diagnostic of ACS. Maxwell’s series observed that nonsurvivors of secondary ACS had a time to OR of 25 hours versus 3 hours for survivors. This indicates that secondary ACS can happen very early in resuscitation. Furthermore, prompt diagnosis and treatment may be beneficial for survival.
Alderson P, Schierhout G, Roberts I, et al. Colloids versus crystalloids for fluid resuscitation in critically ill patients. Cochrane Database Syst Rev. 2000;2:CD000567.
Balogh Z, et al. Supranormal trauma resuscitation causes more cases of abdominal compartment syndrome. Arch Surg. 2003;138(6):637.
Bickell WH, et al. Immediate versus delayed fluid resuscitation for hypotensive patients with penetrating torso injuries. N Engl J Med. 1994;331:1105-1109.
Cannon WB, et al. The preventative treatment on wound shock. JAMA. 1918;70:618-621.
Cooper DJ, Myles PS, McDermott FT, et al. Prehospital hypertonic saline resuscitation of patients with hypotension and severe traumatic brain injury: a randomized controlled trial. JAMA. 2004;291(11):1350-1357.
Dutton MD, et al. Hypotensive resuscitation during active hemorrhage: impact on in-hospital mortality. J Trauma. 2002;52(6):1141-1146.
Gregory JS, et al. Incidence and timing of hypothermia in trauma patients undergoing operations. J Trauma. 1991;31(6):795-798.
Gubler KD, et al. The impact of hypothermia on dilutional coagulopathy. J Trauma. 1994;36(6):847-851.
Hebert PC, Wells G, Blajchman MA, et al. A multicenter, randomized, controlled clinical trial of transfusion requirements in critical care. N Engl J Med. 1999;340:409-417.
Jurkovich GJ, et al. Hypothermia in trauma victims: an ominous predictor of survival. J Trauma. 1987;27(9):1019-1024.
Malone DL, Dunne J, Tracy JK, et al. Blood transfusion, independent of shock severity, is associated with worse outcome in trauma. J Trauma. 2003;54:898-905.
Martini WZ, et al. Independent contributions of hypothermia and acidosis to coagulopathy in swine. J Trauma. 2005;58(5):1002-1009.
McIntyre L, Hebert PC, Wells G, et al. Is a restrictive transfusion strategy safe for resuscitated and critically ill trauma patients? J Trauma. 2004;57(3):563-568.
Reed RD, et al. Hypothermia and blood coagulation: dissociation between enzyme activity and clotting factor levels. Circ Shock. 1990;32(2):141-152.
Rohrer MJ, Natale AM. Effect of hypothermia on the coagulation cascade. Crit Care Med. 1992;20(10):1402-1405.
Schreiber MA, Aoki N, Scott BG, Beck JR. Determinants of mortality in patients with severe blunt head injury. Arch Surg. 2002;137(3):285-290.
Todd SR, Malinoski D, Schreiber MA. Lactated Ringer’s is superior to normal saline in uncontrolled hemorrhagic shock. J Trauma. 2006;61(1):57-64. discussion 64–5
Watters JM, Brundage JM, Todd SR, et al. Resuscitation with lactated Ringer’s does not increase inflammatory response in a Swine model of uncontrolled hemorrhagic shock. Shock. 2004;22(3):283-287.
Watters JM, Tieu BH, Differding JA, Muller PJ, Schreiber MA. A single bolus of 3% hypertonic saline with 6% dextran provides optimal resuscitation after uncontrolled hemorrhagic shock. J Trauma. 2006;61(1):75-81.
Wolberg AS, et al. A systematic evaluation of the effect of temperature on coagulation enzyme activity and platelet function. J Trauma. 2004;56(6):1221-1228.