Chapter 3 Respiratory Mechanics
Introduction
This chapter describes the physical properties of the lungs and chest wall involved in the cyclic processes of ventilation supporting the metabolic needs of the body. The contribution of respiratory muscles to these processes is reviewed here; their function is described more fully in Chapter 6. Clinical measurements of some of these mechanical properties are an important part of pulmonary function testing, as discussed in Chapter 9.
Structure of the Thorax and Lungs
Thorax
The bony thorax protects the lungs, heart, and great vessels but also allows the lungs to change volume from a minimum of 1.5 to 2.0 L to a maximum of 6 to 8 L. This large expansion is made possible by the articulation of the ribs with the spine and the sternum, the arrangement of the muscles, and the motion of the diaphragm. The ribs articulate with the transverse processes of the thoracic vertebrae and have flexible cartilaginous connections with the sternum. The ribs angle down, both from back to front and from midline to side, so that as they elevate, both the anteroposterior and the transverse dimensions of the thorax increase (Figure 3-1). The external intercostal muscles that angle down from posterior to anterior (Figure 3-2) are well situated to elevate the ribs. With deep inspiratory efforts, the first and second ribs are elevated and stabilized by the accessory muscles of respiration in the neck. If the upper extremities are fixed, the pectoralis muscles also can act to raise the ribs (e.g., leaning onto a chair back or against a wall when out of breath). Expiration normally is passive, driven by the elastic recoil of the lung, but can be assisted by the internal intercostal muscles. Forced expiration or a cough requires the abdominal muscles to force the diaphragm upward.
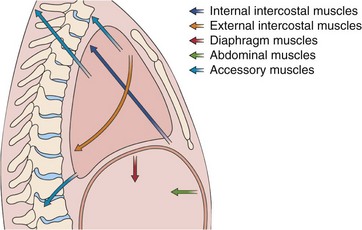
Figure 3-2 Action of the major respiratory muscle groups—intercostals, accessories, diaphragm, and abdominals.
The intercostal muscles are innervated from the thoracic spine at their own level, and the abdominal muscles are innervated from lower thoracic and lumbar level, but the diaphragm is served by the phrenic nerves, which originate at the cervical level (C3 to C5). Thus, the diaphragm remains functional in patients who have spinal injuries below the midcervical level. The long course of each phrenic nerve along the mediastinum, however, makes it vulnerable to both transient and permanent interruptions by disease, injury, or surgery. Occasionally, local irritation of a phrenic nerve leads to intractable singultus (i.e., hiccups). The respiratory muscles are more fully discussed in Chapter 6.
Airways
The number of airway generations required to reach the respiratory zone varies with pathway length, so that areas near the hilum may be reached in 15 generations, whereas those in the periphery may require 25 generations. Although the size of individual airways becomes smaller, the number of airways approximately doubles with each new generation, so that the total cross-sectional area of the combined air path increases. This is especially so in the smaller bronchi and bronchioles, where the “daughters” of each division are only slightly smaller than the “parent.” The rapidly increasing total cross-sectional area of small airways, shown diagrammatically in Figure 3-3, means that their contribution to airflow resistance in the lungs is small. Thus, diseases that affect these peripheral airways may be functionally silent until they reach an advanced state.
Respiratory Mechanics
Lung Volumes
The total gas-containing capacity of the lungs can be divided into a series of “volumes,” as shown in Figure 3-4, which, in combination, give lung “capacities.” The largest amount of air that can be held in the lungs at full inspiration is the total lung capacity (TLC). After a complete forced exhalation, the lungs are not empty but contain a residual volume (RV). The difference between TLC and RV—that is, the greatest volume of air that can be inhaled or exhaled—is the vital capacity (VC). The vital capacity can be affected by factors that either limit expansion of the lung (restrictive processes) or limit lung emptying (airflow obstruction).
A normal breath has a tidal volume (VT) that is only a small portion of the vital capacity (approximately 10%), and even during strenuous exercise, VT increases to only 50% to 60% of VC. Increases in VT occur by extending into the inspiratory reserve and expiratory reserve volumes as shown in Figure 3-4. At the end of a relaxed tidal exhalation, the lungs and chest wall return to a resting position, which normally is approximately 50% of TLC. The volume contained in the lungs at this end-tidal position is the functional residual capacity (FRC), and the volume that can be inhaled from this point is the inspiratory capacity (IC).
The Lung–Chest Wall System
Volumes of Elastic Structures
The recoil tendency of a spring can be expressed in terms of its unstressed or resting length and its length-tension relationship. Similarly, for expandable volumetric structures, the relevant properties are the unstressed volume and the relationship between volume and the transmural pressure required to achieve that volume (Figure 3-5). By convention, transmural pressures are expressed as the difference between the pressure inside and the pressure outside the structure (Pin – Pout). It is convenient to think of this as the distending pressure required to achieve a certain volume. In addition, this distending pressure also represents the recoil pressure, or the tendency of the structure to return to its unstressed volume (where transmural pressure is zero). A positive recoil pressure indicates a tendency to become smaller. A structure distorted to a volume below its unstressed volume has a negative recoil pressure, which indicates its tendency to become larger.
Elastic Properties of the Lung
The lungs are elastic structures with a tendency to recoil to a small “unstressed volume” (usually slightly less than RV). To maintain any lung volume larger than this unstressed volume requires a force that distends the lungs; this force is the difference between the alveolar pressure (PA) and the pressure surrounding the lungs, the intrapleural pressure (Ppl). The elastic properties of the lungs and their tendency to recoil are represented by a plot of the relationship between lung volume and transmural pressure (Figure 3-6). Such graphs apply to an excised lung being inflated by a pump, an in vivo lung inflated by a ventilator, or the more physiologic normal lung inflated by expanding the chest (to create a more negative pleural pressure). In each case, the curve of volume versus the transpulmonary pressure difference (PA − Ppl) is the same.
Elastic Properties of the Chest Wall
The chest wall has elastic properties that can be expressed in the same way as for those of the lung (Figure 3-7). The chest wall differs from many common elastic structures in that its unstressed volume (where recoil pressure = 0) normally is quite high. When expanded above its unstressed volume, it recoils inward, but if the chest wall is “distorted” to a smaller volume, its tendency is to recoil outward. Recoil pressure for the relaxed chest wall is Ppl − Patm, or simply Ppl, because Patm is taken to be zero (Figure 3-8; Table 3-1). The compliance of the chest wall is similar to that of the lungs in the midvolume range; of note, however, at TLC, the chest wall remains as distensible as it is at FRC. At low thoracic volumes, the chest wall compliance becomes very low, resisting further exhalation, and this is the mechanism determining residual volume in children and young adults. By middle age, losses in the elasticity of the tissue attachments supporting small airways cause airway closure to be the mechanism limiting further active exhalation.
Table 3-1 Recoil Pressures of the Lungs, Chest Wall, and Respiratory System, Measured as the Transmural Pressure Difference*
Locus of Measurement | Pressure Components |
---|---|
Lungs | Alveolar pressure (PA) – pleural pressure (Ppl) |
Chest wall | Ppl – atmospheric pressure (Patm), or simply Ppl |
Respiratory system | (PA – Ppl) + (Ppl – Patm) = PA – Patm |
Lung and Chest Wall: The Respiratory System
In the intact thorax, the lungs and chest wall must move together. The muscular effort required to inspire a volume of air, or the pressure that must be developed by a ventilator to achieve the same volume change, is determined by the pressure-volume curve of the combined respiratory system, shown by the red line in Figure 3-7. The lungs and chest wall normally contain the same volume of air, so that only points at the same horizontal level in Figure 3-7 can coexist. Because both the lungs and the chest wall are expanded together, the distending pressure for the respiratory system is the sum of the distending pressures required by the lungs and chest wall. The transmural pressure for the respiratory system is PA – Patm (see Table 3-1). Figure 3-7 shows that a greater pressure change is required to add volume to the respiratory system than to either of its components alone, and thus the compliance of the respiratory system is lower than that of either lungs or chest wall at the same volume. This may at first seem paradoxical, because the tendency of the chest wall to expand might be thought to help lung expansion; however, as the system volume is increased, the outward recoil of the chest wall decreases, and this force must be replaced by additional work.
The third mechanical factor, muscle force, is not considered in Figure 3-7. Thus, the pressure difference across the lung, which has no muscle, can always be taken from its curve, but the pressure across the chest wall (and diaphragm) may reflect muscle tension and is described by this curve only during complete relaxation. Similarly, the curve for the respiratory system shows the pressure that would be measured by a manometer held tightly in the mouth after the subject has inhaled or exhaled to a particular volume and then relaxed all muscle effort.
At the resting end-tidal position of the respiratory system (FRC), no active muscular forces are applied, and PA = Patm (distending pressure = 0). The lung is distended above its low unstressed volume, and the chest wall is held below its relatively high unstressed volume. The relaxed FRC is the volume at which the opposing tendencies of the lungs to recoil inward and the chest wall to recoil outward are evenly balanced. Any change in the unstressed volume or the compliance of either lungs or chest wall results in a new FRC. For example, obesity reduces the unstressed volume of the chest wall and thus also reduces the FRC (and expiratory reserve volume) (see Chapter 62). Emphysema increases both compliance and unstressed volume of the lung, which results in a higher FRC and a “shift to the left” of the respiratory system pressure-volume curve.
The opposing forces of lung and chest wall create a subatmospheric (negative) pressure in the intrapleural space at the FRC (see Figure 3-8). Because the lungs and chest wall are not directly linked, it is actually the intrapleural pressure that opposes lung recoil and chest wall recoil. Thus, at a relaxed FRC, it must have the same magnitude as each of these recoil forces. The average pleural pressure normally is approximately −5 cm H2O at FRC.
Events of the Respiratory Cycle
Respiratory Muscle Effort
The maximum inspiratory and expiratory pressures measure the maximal efforts of the respiratory muscles (Figure 3-9). That is, with an inhalational effort against a closed pressure manometer, the maximum negative pressure that can be generated at the mouth is approximately 100 cm H2O at a low lung volume. At TLC, no negative pressure can be generated, so no more air can be drawn into the chest. Maximum expiratory pressures are somewhat greater, measuring 150 to 200 cm H2O at high lung volume, and fall to zero at RV.
Surface Tension
At the surface of a liquid, the intermolecular forces are not balanced by the more widely spaced molecules of the gas phase, which creates a surface tension. The surface tension of the air-liquid interface that lines the alveoli contributes an important part of the elastic properties of the lung shown by the pressure-volume curve. If a lung is filled with liquid, surface forces are abolished, and the resultant pressure-volume curve (Figure 3-10) reflects only the tissue properties of the lung. This liquid-filled curve is shifted to the left, indicating that the lung can be distended with much less pressure. The air-filled lung, in addition to requiring greater pressures, demonstrates marked hysteresis; that is, the pressure-volume curve during inflation is different from that during deflation.
• The work needed to expand the lungs is greatly reduced.
• Stability of alveoli and terminal airways is maintained. (If pressure increased within an alveolus as it became smaller, the alveolus would tend to empty into interconnected, larger alveoli with lower pressure.)
• Inwardly directed forces of surface tension in the “corners” of alveoli act to draw fluid from the capillaries and interstitium into the alveoli, so lowering surface tension helps prevent alveolar edema (discussed in Chapter 4).
Pulmonary surfactant is produced in alveolar type II cells in the form of lamellar bodies, appears in the alveolar lining liquid as tubular myelin, and then spreads as a monolayer at the air-liquid interface. The major component, and the component that is primarily responsible for the surface tension–lowering effects, is dipalmitoyl phosphatidylcholine (DPPC). DPPC has a nonpolar end, made up of two saturated fatty acid chains, and a polar end that tends to have a positive charge. At the air-liquid interface, the molecules orient with the hydrophilic polar end in the liquid and the fatty acid chains projecting into the air (Figure 3-11). Both ends have similar cross-sectional area allowing them to pack closely together. The molecules may also adsorb directly to the epithelial surface, which tends to have a negative charge, in areas where a liquid subphase is absent.
Flow Resistance
Airflow resistance (Raw) is affected by the following factors:
All of these factors are similar during both inspiration and expiration, except the last. During inspiration, the intrathoracic pressure that surrounds the airways is more negative than the intraluminal pressure, so airways tend to be distended (Figure 3-12). During passive exhalation, the magnitude of the airway distending force is lower, so airflow resistance is somewhat higher. With active expiratory efforts, the pleural pressure becomes positive, and with the addition of lung recoil, the pressure in the alveoli is even higher. However, the intraluminal pressure decreases progressively in airways mouthward of the alveoli, reflecting both frictional losses and a decrease in lateral pressure through the Bernoulli effect, because the decreasing cross-sectional area of the composite airway requires a marked increase in velocity of air movement (convective acceleration). Because their cartilaginous structure is incomplete, airways are compressed under such forces, and calculated resistance is much higher.
Maximum airflow rates are evaluated by having the subject take a full inspiration to TLC and blow the air out as forcefully and completely (to RV) as possible. With use of a spirometer, this forced vital capacity (FVC) is recorded as an expiratory spirogram (volume versus time), or if the flow rate is directly measured, the same information can be recorded as a maximum expiratory flow versus volume curve (Figure 3-13). A remarkable feature of this maneuver is that the maximum flow rate for any volume, except the higher lung volumes near the beginning of the exhalation, is achieved with submaximal effort and cannot be exceeded with further effort. This flow limitation, or “effort independence,” is demonstrated in Figure 3-14 and is a consequence of the dynamic compression noted previously. The mechanism of this flow limitation is related to the rate of propagation of a pressure wave through a compliant tube, but the result can be understood with a simpler conceptual model of dynamic compression. Because this compression begins just beyond the point at which intraairway pressure falls to equal pleural pressure, the effective pressure driving flow from the alveoli to this point becomes PA − Ppl (i.e., in Figure 3-12, for forced expiration, 30 − 20 = 10 cm H2O). This is the same as the elastic recoil pressure of the lung and is a function of lung volume, not effort. If, in the example of Figure 3-12, a greater expiratory effort is made and the pleural pressure is raised to 40 cm H2O at the same lung volume, the alveolar pressure becomes 50 cm H2O and the effective driving pressure = (50 − 40) = 10 cm H2O, so the resultant flow rate remains unchanged.
Work of Breathing
The elastic and frictional components of respiratory work are affected differently by lung volume. At low lung volume, airways are narrower, and resistance (and thus frictional work) increases rapidly (R is proportional to 1/r4). At higher lung volumes, the airways are larger, but muscles must do more elastic work to keep the lungs stretched. The relaxed FRC is the volume at which the static recoil forces of the lung and chest wall are balanced, but Figure 3-15 shows that FRC also is the volume at which work of breathing is least. If either the elastic or frictional contributions to work of breathing change, FRC may change rapidly or chronically.
Restrictive disease processes reduce lung compliance (CL). Accordingly, the force the muscles must generate to stretch the lung increases. The elastic work required to breathe at any lung volume is higher, and this shifts the volume for least work lower. Increased CL, as with emphysema, has the opposite effect. Figure 3-7 shows that the static forces predict the same changes in FRC (greater lung recoil results in lower FRC volume and vice versa).
Summary
The mechanical properties of the lungs and chest wall are described by the interactions of volume and pressure within the thorax, and by flows and resistance during dynamic changes in lung volume. Alterations in these properties underlie many respiratory diseases and contribute to their associated symptoms. Measurement of these properties is important in elucidation of the pathophysiology of lung diseases and in diagnosis and monitoring of disease in the pulmonary function laboratory (see Chapter 9), and the increasing sophistication of modern ventilators makes an understanding of thoracic mechanics essential in the intensive care unit (see Chapter 32).
Controversies and Pitfalls
The principles of lung mechanics discussed in this chapter have been well established for the past 40 to 60 years, but their application in some clinical situations is less clear-cut. Inadequate understanding of lung–chest wall pressure relationships underlies the occasionally heard bedside comment that increasing positive end-expiratory pressure (PEEP) to improve hypoxemia in a mechanically ventilated patient with acute lung injury (ALI) will not affect hemodynamics “because the lungs are stiff and no added pressure will be transmitted to the pleural space.” It is true that low lung compliance will result in a lesser expansion of lung volume for any increase in alveolar pressure, with less increase in average intrapleural pressure than in a normal chest. If there is an improvement in oxygenation, however, the likely mechanism is recruitment of alveoli with an increase in end-expiratory lung volume, and this necessitates that the chest wall also must be expanded along its passive pressure-volume curve (see Figure 3-7). Because this relationship has a positive slope throughout, any increase in thoracic volume must be accompanied by at least some increase in intrapleural pressure, with its potential for a hemodynamic effect.
Faffe DS, Zin WA. Lung parenchymal mechanics in health and disease. Physiol Rev. 2009;89:759–775.
Gibson GJ. Lung volumes and elasticity. In: Hughes JM, Pride NB. Lung function tests: physiologic principles and clinical applications. London: WB Saunders; 1999:45–56.
Hager DN, Krishnan JA, Hayden DL, Brower RG. Tidal volume reduction in patients with acute lung injury when plateau pressures are not high. Am J Respir Crit Care Med. 2005;172:1241–1245.
Heil M, Hazel AL, Smith JA. The mechanics of airway closure. Respir Physiol Neurobiol. 2008;163:214–221.
Hubmayer RD. Straining to make mechanical ventilation safe and simple. Am J Respir Crit Care Med. 2011;183:1289–1290.
Hills BA. Surface-active phospholipids: a Pandora’s box of clinical applications. Part I: the lung and air spaces. Int Med J. 2002;32:170–178.
Loring SH, Garcia-Jacques M, Malhotra A. Pulmonary characteristics in COPD and mechanisms of increased work of breathing. J Appl Physiol. 2009;107:309–314.
Lucangelo U, Bernabe F, Blanch L. Lung mechanics at the bedside: make it simple. Curr Opin Crit Care. 2007;13:64–72.
O’Donnell DE, Laveneziana P. Dyspnea and activity limitation in COPD: mechanical factors. COPD. 2007;4:225–236.
Pride NB. Airflow resistance. In: Hughes JM, Pride NB. Lung function tests: physiologic principles and clinical applications. London: WB Saunders; 1999:27–44.
Ratnovsky A, Elad D, Halpern E. Mechanics of respiratory muscles. Respir Physiol Neurobiol. 2008;168:82–89.
Schurch S, Bachofen H, Possmeyer F. Alveolar lining layer: functions, composition, structures. In: Hlastala MP, Robertson HT. Lung biology in health and disease, vol 121: complexity in structure and function of the lung. New York: Marcel Dekker; 1998:35–98.
Weibel ER. What makes a good lung? The morphometric basis of lung function. Swiss Med Wkly. 2009;139:375–386.