Chapter 15 Respiratory disease
Structure of the respiratory system
The trachea, bronchi and bronchioles
The first seven divisions are bronchi that have:
walls consisting of cartilage and smooth muscle
epithelial lining with cilia and goblet cells
submucosal mucus-secreting glands
endocrine cells – Kulchitsky or APUD (amine precursor and uptake decarboxylation) containing 5-hydroxytryptamine.
The next 16–18 divisions are bronchioles that have:
no cartilage and a muscular layer that progressively becomes thinner
a single layer of ciliated cells but very few goblet cells
granulated Clara cells that produce a surfactant-like substance.
The ciliated epithelium is a key defence mechanism. Each cell bears approximately 200 cilia beating at 1000 beats per minute in organized waves of contraction. Each cilium consists of nine peripheral parts and two inner longitudinal fibrils in a cytoplasmic matrix (Fig. 15.1). Nexin links join the peripheral pairs. Dynein arms consisting of ATPase protein project towards the adjacent pairs. Bending of the cilia results from a sliding movement between adjacent fibrils powered by an ATP-dependent shearing force developed by the dynein arms (see also p. 22). Absence of dynein arms leads to immotile cilia. Mucus, which contains macrophages, cell debris, inhaled particles and bacteria, is moved by the cilia towards the larynx at about 1.5 cm/min (the ‘mucociliary escalator’, see below).
The bronchioles finally divide within the acinus into smaller respiratory bronchioles that have alveoli arising from the surface (Fig. 15.2). Each respiratory bronchiole supplies approximately 200 alveoli via alveolar ducts. The term ‘small airways’ refers to bronchioles of <2 mm; the average lung contains about 30 000 of these.
The alveoli
There are approximately 300 million alveoli in each lung. Their total surface area is 40–80 m2. The epithelial lining consists mainly of type I pneumocytes (Fig. 15.3). These cells have an extremely thin layer of cytoplasm, which only offers a thin barrier to gas exchange. Type I cells are connected to each other by tight junctions that limit the movements of fluid in and out of the alveoli. Alveoli are not completely airtight – many have holes in the alveolar wall allowing communication between alveoli of adjoining lobules (pores of Kohn).
The lungs
The lungs are separated into lobes by invaginations of the pleura, which are often incomplete. The right lung has three lobes, whereas the left lung has two. The positions of the oblique fissures and the right horizontal fissure are shown in Figure 15.4. The upper lobe lies mainly in front of the lower lobe and therefore physical signs on the right side in the front of the chest are due to lesions of the upper lobe or the middle lobe.
Physiology of the respiratory system
Breathing
Lung ventilation can be considered in two parts:
The mechanical process of inspiration and expiration
The control of respiration to a level appropriate for metabolic needs.
The control of respiration
The pulmonary blood flow of 5 L/min carries 11 mmol/min (250 mL/min) of oxygen from the lungs to the tissues.
Ventilation at about 6 L/min carries 9 mmol/min (200 mL/min) of carbon dioxide out of the body.
The normal pressure of oxygen in arterial blood (PaO2) is between 11 and 13 kPa.
The normal pressure of carbon dioxide in arterial blood (PaCO2) is 4.8–6.0 kPa.
Ventilation is controlled by a combination of neurogenic and chemical factors (Fig. 15.5).
The airways of the lungs
Airflow
As air flows from the alveoli towards the mouth there is a gradual drop of pressure owing to flow resistance (Fig. 15.6a).
In forced expiration, as mentioned above, the driving pressure raises both the alveolar pressure and the intrapleural pressure. Between the alveolus and the mouth, there is a point (C in Fig. 15.6b) where the airway pressure equals the intrapleural pressure, and the airway collapses. However, this collapse is temporary, as the transient occlusion of the airway results in an increase in pressure behind it (i.e. upstream) and this raises the intra-airway pressure so that the airways open and flow is restored. The airways thus tend to vibrate at this point of ‘dynamic collapse’.
As lung volume decreases during expiration, the elastic recoil pressure of the lungs decreases and the ‘collapse point’ moves upstream (i.e. towards the smaller airways – see Fig. 15.6c). Where there is pathological loss of recoil pressure (as in chronic obstructive pulmonary disease, COPD), the ‘collapse point’ is located even further upstream and causes expiratory flow limitation. The measurement of the forced expiratory volume in 1 second (FEV1) is a useful clinical index of this phenomenon. To compensate, patients with COPD often ‘purse their lips’ in order to increase airway pressure so that their peripheral airways do not collapse. During inspiration, the intrapleural pressure is always less than the intraluminal pressure within the intrathoracic airways, so increasing effort does not limit airflow. Inspiratory flow is limited only by the power of the inspiratory muscles.
Flow-volume loops
The relationship between maximal flow rates and lung volume is demonstrated by the maximal flow-volume (MFV) loop (Fig. 15.7a).
In subjects with healthy lungs, maximal flow rates are rarely achieved even during vigorous exercise. However, in patients with severe COPD, limitation of expiratory flow occurs even during tidal breathing at rest (Fig. 15.7b). To increase ventilation these patients have to breathe at higher lung volumes and allow more time for expiration, both of which reduce the tendency for airway collapse. To compensate they increase flow rates during inspiration, where there is relatively less flow limitation.
The volume that can be forced in from the residual volume in 1 second (FIV1) will always be greater than that which can be forced out from TLC in 1 second (FEV1). Thus, the ratio of FEV1 to FIV1 is below 1. The only exception to this occurs when there is significant obstruction to the airways outside the thorax, such as tracheal tumour or retrosternal goitre. Expiratory airway narrowing is prevented by tracheal resistance and expiratory airflow becomes more effort-dependent. During forced inspiration this same resistance causes such negative intraluminal pressure that the trachea is compressed by the surrounding atmospheric pressure. Inspiratory flow thus becomes less effort-dependent, and the ratio of FEV1 to FIV1 exceeds 1. This phenomenon, and the characteristic flow-volume loop, is diagnostic of extrathoracic airways obstruction (Fig. 15.7c).
Ventilation and perfusion relationships
For optimum gas exchange there must be a match between ventilation of the alveoli () and their perfusion (
). However, in reality there is variation in the (
) ratio in both normal and diseased lungs (Fig. 15.8). In the normal lung both ventilation and perfusion are greater at the bases than at the apices, but the gradient for perfusion is steeper, so the net effect is that ventilation exceeds perfusion towards the apices, while perfusion exceeds ventilation at the bases. Other causes of (
) mismatch include direct shunting of deoxygenated blood through the lung without passing through alveoli (e.g. the bronchial circulation) and areas of lung that receive no blood (e.g. anatomical deadspace, bullae and areas of underperfusion during acceleration and deceleration, e.g. in aircraft and high performance cars).
Hypoxaemia occurs more readily than hypercapnia because of the different ways in which oxygen and carbon dioxide are carried in the blood. Carbon dioxide can be considered to be in simple solution in the plasma, the volume carried being proportional to the partial pressure. Oxygen is carried in chemical combination with haemoglobin in the red blood cells, with a non-linear relationship between the volume carried and the partial pressure (Fig. 15.5, p. 341). Alveolar hyperventilation reduces the alveolar PCO2 (PACO2) and diffusion leads to a proportional fall in the carbon dioxide content of the blood (PaCO2). However, as the haemoglobin is already saturated with oxygen, there is no significant increase in the blood oxygen content as a result of increasing the alveolar PO2 through hyperventilation. The hypoxaemia of even a small amount of physiological shunting cannot therefore be compensated for by hyperventilation.
Defence mechanisms of the respiratory tract
Pulmonary disease often results from a failure of the normal host defence mechanisms of the healthy lung (Fig. 15.9). These can be divided into physical, physiological, humoral and cellular mechanisms.
Humoral and cellular mechanisms
Nonspecific soluble factors
α-Antitrypsin (α1-antiprotease, see p. 341) in lung secretions is derived from plasma. It inhibits chymotrypsin and trypsin and neutralizes proteases including neutrophil elastase.
Antioxidant defences include enzymes such as superoxide dismutase and low-molecular-weight antioxidant molecules (ascorbate, urate) in the epithelial lining fluid. In addition, lung cells are protected by an extensive range of intracellular defences, especially members of the glutathione S-transferase (GST) superfamily.
Lysozyme is an enzyme found in granulocytes that has bactericidal properties.
Lactoferrin is synthesized from epithelial cells and neutrophil granulocytes and has bactericidal properties.
Interferons are produced by most cells in response to viral infection and are potent modulators of lymphocyte function.
Complement in secretions is also derived from plasma. In association with antibodies, it plays a major role in cytotoxicity.
Surfactant protein A (SPA) is one of four species of surfactant proteins which opsonizes bacteria/particles, enhancing phagocytosis by macrophages.
Defensins are bactericidal peptides present in the azurophil granules of neutrophils.
Innate and adaptive immunity
With infection, neutrophils migrate out of pulmonary capillaries into the air spaces and phagocytose and kill microbes with, for example, antimicrobial proteins (lactoferrin), degradative enzymes (elastase) and oxidant radicals. In addition, neutrophil extracellular traps (NET) ensnare and kill extracellular bacteria. Neutrophils also generate a variety of mediators, e.g. TNF-α, IL-1 and chemokines which activate dendritic cells and B cells and produce the T-cell-activating cytokine IL-12. The latter enhances neutrophil-mediated defence during pneumonia. Dendritic cells are antigen presenting cells and are key to the adaptive immune response (p. 58).
Symptoms
Runny, blocked nose and sneezing
Nasal symptoms (see also p. 691) are extremely common and both common colds and allergic rhinitis cause ‘runny nose’ (rhinorrhoea), nasal blockage and attacks of sneezing. In allergic rhinitis, symptoms may be intermittent, following contact with pollens or animal danders, or persistent, especially when house-dust mite is the allergen. Colds are frequent during the winter but if the symptoms persist for weeks the patient probably has perennial rhinitis rather than persistent viral infection.
Nasal secretions are usually thin and runny in allergic rhinitis but thicker and discoloured with viral infections. Nose bleeds and blood-stained nasal discharge are common and rarely indicate serious pathology. However, a blood-stained nasal discharge associated with nasal obstruction and pain may be the presenting feature of a nasal tumour (p. 1051). Nasal polyps typically present with nasal blockage and loss of smell.
Cough
Cough (see also p. 822) is the commonest symptom of lower respiratory tract disease. It is caused by mechanical or chemical stimulation of cough receptors in the epithelium of the pharynx, larynx, trachea, bronchi and diaphragm. Afferent receptors go to the cough centre in the medulla where efferent signals are generated to the expiratory musculature. Smokers often have a morning cough with a little sputum. A productive cough is the cardinal feature of chronic bronchitis, while dry coughing, particularly at night, can be a symptom of asthma. Cough also occurs in asthmatics after mild exertion or following forced expiration. Cough can also occur for psychological reasons without any definable pathology.
Sputum
Haemoptysis (blood-stained sputum) varies from small streaks of blood to massive bleeding.
The commonest cause of mild haemoptysis is acute infection, particularly in exacerbations of chronic obstructive pulmonary disease (COPD) but it should not be attributed to this without investigation.
Other common causes are pulmonary infarction, bronchial carcinoma and tuberculosis.
In lobar pneumonia, the sputum is usually rusty in appearance rather than frankly blood-stained.
Pink, frothy sputum is seen in pulmonary oedema.
In bronchiectasis, the blood is often mixed with purulent sputum.
Massive haemoptyses (>200 mL of blood in 24 hours) are usually due to bronchiectasis or tuberculosis.
Uncommon causes of haemoptyses are idiopathic pulmonary haemosiderosis, Goodpasture’s syndrome, microscopic polyangiitis, trauma, blood disorders and benign tumours.
Breathlessness
Orthopnoea (see p. 675) is breathlessness on lying down. While it is classically linked to heart failure, it is partly due to the weight of the abdominal contents pushing the diaphragm up into the thorax. Such patients may also become breathless on bending over.
Hyperventilation is inappropriate overbreathing. This may occur at rest or on exertion and results in a lowering of the alveolar and arterial PCO2 (see p. 1178).
Paroxysmal nocturnal dyspnoea (see p. 798) is acute episodes of breathlessness at night, typically due to heart failure.
Examination of the respiratory system
The chest
Examination of the chest
Inspection
Cyanosis (see p. 676) is a dusky colour of the skin and mucous membranes, due to the presence of more than 50 g/L of desaturated haemoglobin. When due to central causes, cyanosis is visible on the tongue (especially the underside) and lips. Patients with central cyanosis will also be cyanosed peripherally. Peripheral cyanosis without central cyanosis is caused by a reduced peripheral circulation and is noted on the fingernails and skin of the extremities with associated coolness of the skin.
Finger clubbing is present when the normal angle between the base of the nail and the nail fold is lost. The base of the nail is fluctuant owing to increased vascularity, and there is an increased curvature of the nail in all directions, with expansion of the end of the digit. Some causes of clubbing are given in Table 15.1. Clubbing is not a feature of uncomplicated COPD.
Palpation and percussion
Check the position of the trachea and apex beat. Examine the supraclavicular fossa for enlarged lymph nodes. The distance between the sternal notch and the cricoid cartilage (three to four finger breadths in full expiration) is reduced in patients with severe airflow limitation. Check chest expansion. A tape measure is used if precise or serial measurements are needed, e.g. in ankylosing spondylitis. Local discomfort over the sternochondral joints suggests costochondritis. In rib fractures, compression of the chest laterally and anteroposteriorly produces localized pain. On percussion, liver dullness is usually detected anteriorly at the level of the sixth rib. Liver and cardiac dullness disappear when lungs are over-inflated (Table 15.2).
Investigation of respiratory disease
Imaging
Chest X-ray
Centring of the film. The distance between each clavicular head and the spinal processes should be equal
Penetration (check film is not too dark)
View. Routine films are taken postero-anterior (PA), i.e. the film is placed in front of the patient with the X-ray source behind. Anteroposterior (AP) films are taken only in very ill patients who are unable to stand up or be taken to the radiology department; the cardiac outline appears bigger and the scapulae cannot be moved out of the way. Lateral chest X-rays were often performed in the past to localize pathology, but CT scans have replaced these.
X-ray abnormalities
Collapse and consolidation
Simple pneumonia is easy to recognize (see Fig. 15.33) but look carefully for any evidence of collapse (Fig. 15.10, Table 15.3). Loss of volume or crowding of the ribs are the best indicators of lobar collapse. The lung lobes collapse in characteristic directions. The lower lobes collapse downwards and towards the mediastinum, the left upper lobe collapses forwards against the anterior chest wall, while the right upper lobe collapses upwards and inwards, forming the appearance of an arch over the remaining lung. The right middle lobe collapses anteriorly and inward, obscuring the right heart border. If a whole lung collapses, the mediastinum will shift towards the side of the collapse. Uncomplicated consolidation does not cause mediastinal shift or loss of lung volume, so any of these features should raise the suspicion of an endobronchial obstruction.
Pleural effusion
Pleural effusions (see Fig. 15.45) need to be larger than 500 mL to cause much more than blunting of the costophrenic angle. On an erect film they produce a characteristic shadow with a curved upper edge rising into the axilla. If very large, the whole of one hemithorax may be opaque, with mediastinal shift away from the effusion.
Fibrosis
Localized fibrosis causes streaky shadowing, and the accompanying loss of lung volume causes mediastinal structures to move to the same side. More generalized fibrosis can lead to a honeycomb appearance (see p. 849), seen as diffuse shadows containing multiple circular translucencies a few millimetres in diameter.
Round shadows
Lung cancer is the commonest cause of large round shadows but many other causes are recognized (Table 15.4).
Miliary mottling
This term, derived from the Latin for millet, describes numerous minute opacities, 1–3 mm in size. The commonest causes are tuberculosis, pneumoconiosis, sarcoidosis, idiopathic pulmonary fibrosis and pulmonary oedema (see Fig. 14.15), although pulmonary oedema is usually perihilar and accompanied by larger, fluffy shadows. Pulmonary microlithiasis is a rare but striking cause of miliary mottling.
Computed tomography
CT provides excellent images of the lungs and mediastinal structures (Fig. 15.11). Narrow slice, high-resolution CT scans show the lung parenchyma well, while thicker slice staging CT scans are used for diagnosis of malignant disease. Mediastinal structures are shown more clearly after injecting intravenous contrast medium.
Evaluating diffuse disease of the lung parenchyma, including sarcoidosis, hypersensitivity pneumonitis, occupational lung disease, and any other form of interstitial pulmonary fibrosis.
Diagnosis of bronchiectasis. HRCT has a sensitivity and specificity >90%.
Distinguishing emphysema from diffuse parenchymal lung disease or pulmonary vascular disease as a cause of a low gas transfer factor with otherwise normal lung function.
Suspected opportunistic lung infection in immunocompromised patients
Scintigraphic imaging
Ventilation–perfusion scan
Xenon-133 gas is inhaled and its distribution is detected at the same time as the perfusion scan. Areas affected by pulmonary embolism will have reduced perfusion relative to ventilation (see Chapter 14). Other lung diseases (e.g. asthma or pneumonia) impair both ventilation and perfusion. Unfortunately, a pulmonary embolus can affect the lung substance (e.g. atelectasis) leading to reduced ventilation. Nevertheless, this is a better technique than perfusion scan alone.
Respiratory function tests (Table 15.5)
Tests of ventilatory function
These tests are used mainly to assess the degree of airflow limitation during expiration.
Spirometry
The patient takes a maximum inspiration followed by a forced expiration (for as long as possible) into the spirometer. The spirometer measures the one second forced expiratory volume (FEV1) and the total volume of exhaled gas (forced vital capacity, FVC). Both FEV1 and FVC are related to height, age and sex (Fig. 15.12).
Peak expiratory flow rate (PEFR)
This is an extremely simple and cheap test. Subjects take a full inspiration to total lung capacity and then blow out forcefully into the peak flow meter (Fig. 15.13). The best of three attempts is recorded.
Although reproducible, PEFR is mainly dependent on the flow rate in larger airways and it may be falsely reassuring in patients with moderate airflow limitation. PEFR is mainly used to diagnose asthma, and to monitor exacerbations of asthma and response to treatment. Regular measurements of peak flow rates on waking, during the afternoon, and before going to bed demonstrate the wide diurnal variations in airflow limitation that characterize asthma and allow objective assessment of response to treatment (Fig. 15.14).
Other ventilatory function tests
Flow-volume loops
Plotting flow rates against expired volume (flow-volume loops, see Fig. 15.7) shows the site of airflow limitation within the lung. At the start of expiration from TLC, maximum resistance is from the large airways, and this affects the flow rate for the first 25% of the curve. As air is exhaled, lung volume reduces and the flow rate becomes dependent on the resistance of smaller airways. In chronic obstructive pulmonary disease (COPD), where the disease mainly affects the smaller airways, expiratory flow rates at 50% or 25% of the vital capacity are disproportionately reduced when compared with flow rates at larger lung volumes. Flow-volume loops will also show obstruction of large airways, e.g. tracheal narrowing due to tumour or retrosternal goitre.
Lung volumes
The subdivisions of lung volume are shown in Figure 15.15. Tidal volume and vital capacity can be measured using a simple spirometer, but alternative techniques are needed to measure TLC and RV. TLC is measured by inhaling air containing a known concentration of helium and measuring its dilution in the exhaled air. RV can be calculated by subtracting the vital capacity from the TLC.
Haematological and biochemical tests
Sputum
Sputum should be inspected for colour:
Yellowish-green indicates inflammation (infection or allergy)
Blood suggests neoplasm or pulmonary infarct (see haemoptysis, p. 798).
Intercostal drainage
This is carried out when large effusions are present, producing severe breathlessness, or for drainage of an empyema (see Practical Box 15.1). Drains should be inserted with ultrasound guidance. Pleurodesis is performed for recurrent/malignant effusion.
Practical Box 15.1
Intercostal drainage
Explain to the patient the nature of the procedure.
Fibreoptic bronchoscopy
See Practical Box 15.2 and Fig. 15.16.
Practical Box 15.2
Fibreoptic bronchoscopy
Indications
Lesions requiring biopsy seen on chest X-ray
Positive sputum cytology for malignant cells with no chest X-ray abnormality
Collection of bronchial secretions for bacteriology, especially tuberculosis
Recurrent laryngeal nerve paralysis of unknown aetiology
Infiltrative lung disease (to obtain a transbronchial biopsy)
Investigation of collapsed lobes or segments and aspiration of mucus plugs.
Disadvantages
All patients require sedation to tolerate the procedure.
Minor and transient cardiac dysrhythmias occur in up to 40% of patients on passage of the bronchoscope through the larynx. Monitoring is required.
Oxygen supplementation is required in patients with PaO2 below 8 kPa.
Fibreoptic bronchoscopy should be performed with care in the very sick, and transbronchial biopsies avoided in ventilated patients owing to the increased risk of pneumothorax.
Massive bleeding may occur after biopsy of vascular lesions or carcinoid tumours. Rigid bronchoscopy may be required to allow adequate access to the bleeding point for haemostasis.
Video-assisted thoracoscopic (VATS) lung biopsy
This technique has largely replaced open thoracotomy when a lung biopsy is required (p. 850).
Smoking and air pollution
Smoking
The dangers
Cigarette smoking is addictive and harmful to health (Table 15.6). People usually start smoking in adolescence for psychosocial reasons and, once they smoke regularly, the pharmacological properties of nicotine encourage persistence, by their effect on the smoker’s mood. Very few cigarette smokers (<2%) can limit themselves to occasional or intermittent smoking.
|
Significant dose–response relationships exist between cigarette consumption, airway inflammation (Table 15.7) and lung cancer mortality. Sputum production and airflow limitation increase with daily cigarette consumption, and effort tolerance decreases. Smoking 20 cigarettes daily for 20 years increases the lifetime risk of lung cancer by about 10 times compared to a lifelong non-smoker. Smoking and asbestos exposure are synergistic risk factors for lung cancer, with a combined risk of about 90 times that of unexposed non-smokers.
Table 15.7 Effects of smoking on the lung
|
FURTHER READING
Gu Dongfeng, Kelly TN, Wu Xigui et al. Mortality attributable to smoking in China. N Engl J Med 2009; 360:150–159.
Hales S, Howden-Chapman P. Effects of air pollution on health. BMJ 2007; 335:314–315.
Lippmann M. Health effects of airborne particulate matter. N Engl J Med 2007; 57:2395–2397.
Oberg M, Jaakkola MS, Woodward A et al. Worldwide burden of disease from exposure to second-hand smoke: a retrospective analysis of data from 192 countries. Lancet 372:139–46.
Oncken C. Nicotine replacement for smoking cessation during pregnancy. N Engl J Med 2012; 366:846–847.
WHO. WHO Air quality guidelines for particulate matter, ozone, nitrogen dioxide and sulfur dioxide. Global update 2005. Copenhagen: World Health Organization; 2005.
Air pollution and epidemiology
Although it has been proposed that air pollution may cause asthma and other allergic diseases, there is no current evidence for this (Table 15.8). However, air pollution does adversely affect lung development in teenage children, while both NO2 and ozone enhance the nasal and lung airway responses to inhaled allergen in people with established allergic disease.
Diseases of the upper respiratory tract
Rhinitis
For a limited period of the year (seasonal or intermittent rhinitis)
Throughout the whole year (perennial or persistent rhinitis).
Seasonal rhinitis
Nasal irritation, sneezing and watery rhinorrhoea are the most troublesome symptoms, but many also suffer from itching of the eyes and soft palate and occasionally even itching of the ears because of the common innervation of the pharyngeal mucosa and the ear. In addition, approximately 20% suffer from seasonal wheezing. The common seasonal allergens are shown in Figure 15.17. Since pollination of plants that give rise to high pollen counts varies from country to country, seasonal rhinoconjunctivitis and accompanying wheeze may occur at different times of year in different regions.
Perennial rhinitis
Perennial allergic rhinitis
The commonest cause is allergy to the faecal particles of the house-dust mite Dermatophagoides pteronyssinus or D. farinae; these are under 0.5 mm in size, invisible to the naked eye (Fig. 15.18), and found in dust throughout the house, particularly in older, damp dwellings. Mites live off desquamated human skin scales and the highest concentrations (4000 mites/g of surface dust) are found in human bedding. Their faecal particles are approximately 20 µm in diameter (Fig. 15.18), and impact in the nose rather than the lungs, unless the patient breathes through their mouth.
Pathogenesis
Sneezing, increased secretion and changes in mucosal blood flow are mediated both by efferent nerve fibres and by released mediators (see p. 827). Mucus production results largely from parasympathetic stimulation. Blood vessels are under both sympathetic and parasympathetic control. Sympathetic fibres maintain tonic contraction of blood vessels, keeping the sinusoids of the nose partially constricted with good nasal patency. Stimulation of the parasympathetic system dilates these blood vessels. This stimulation varies spontaneously in a cyclical fashion so that air intake alternates slowly over several hours from one nostril to the other. The erectile cavernous nasal sinusoids can be influenced by emotion, which, in turn, can affect nasal patency.
Treatment
Allergen avoidance
The house-dust mite infests most areas of the house, but particularly the bedroom. Mite allergen exposure can be reduced by enclosing bedding in fabric specifically designed to prevent the passage of mite allergen, while allowing water vapour through. This is both comfortable and reduces symptoms. Acaricides are less effective and cannot be recommended. Increased room ventilation and reduced soft furnishings including carpets, curtains and soft toys are all helpful in reducing the mite load. However, a meta-analysis has questioned the value of house-dust mite control measures in asthma (p. 829).
Immunotherapy
This is used for patients with seasonal allergic rhinitis who have not responded to the above. Both oral and injectable vaccines are available (p. 69). Other forms of desensitizing vaccines are under development.
Pharyngitis
Over several decades, the proportion of sore throats due to bacterial infections, e.g. haemolytic streptococcus, has fallen. Many different pathogens have been implicated in pharyngitis but most do not require specific treatment. Persistent and severe tonsillitis should be treated with phenoxymethylpenicillin 500 mg four times a day or cefaclor 250 mg three times daily. Amoxicillin and ampicillin should be avoided if there is a possibility of infectious mononucleosis (p. 100).
Influenza (see also p. 108)
The influenza virus belongs to the orthomyxovirus group and exists in two main forms, A and B. Influenza B is associated with localized outbreaks of mild disease, whereas influenza A causes worldwide pandemics (see p. 108).
Inhalation of foreign bodies
When the foreign body is large it may impact in the trachea. The person chokes and then becomes silent; death ensues unless the material is quickly removed (see Emergency Box 15.1).
Emergency Box 15.1
Treatment of inhaled foreign bodies (Heimlich manoeuvre)
More often, impaction occurs in the right main bronchus and produces:
Diseases of the lower respiratory tract
Chronic obstructive pulmonary disease (COPD)
Epidemiology and aetiology
Climate and air pollution are lesser causes of COPD, but mortality from COPD increases dramatically during periods of heavy atmospheric pollution (p. 341). Urbanization, social class and occupation may also play a part in aetiology, but these effects are difficult to separate from that of smoking. Some animal studies suggest that diet could be a risk factor for COPD, but this has not been proven in humans.
Pathophysiology
The most consistent pathological finding in COPD is increased numbers of mucus-secreting goblet cells in the bronchial mucosa, especially in the larger bronchi (Fig. 15.19). In more advanced cases, the bronchi become overtly inflamed and pus is seen in the lumen.
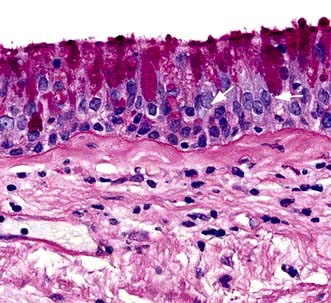
Figure 15.19 COPD. Section of bronchial mucosa stained for mucus glands by PAS showing increase in mucus-secreting goblet cells.
(Courtesy of Dr J Wilson and Dr S Wilson, University of Southampton.)
Microscopically, there is infiltration of the walls of the bronchi and bronchioles with acute and chronic inflammatory cells; lymphoid follicles may develop in severe disease. In contrast to asthma, the lymphocytic infiltrate is predominantly CD8+. The epithelial layer may become ulcerated and, with time, squamous epithelium replaces the columnar cells. The inflammation is followed by scarring and thickening of the walls which narrows the small airways (Fig. 15.20).
Centri-acinar emphysema. Distension and damage of lung tissue is concentrated around the respiratory bronchioles, whilst the more distal alveolar ducts and alveoli tend to be well preserved. This form of emphysema is extremely common; when of modest extent, it is not necessarily associated with disability. Severe centri-acinar emphysema is associated with substantial airflow limitation.
Pan-acinar emphysema. This is less common. Distension and destruction affect the whole acinus, and in severe cases the lung is just a collection of bullae. Severe airflow limitation and
mismatch occur. α1-Antitrypsin deficiency is associated with this type of emphysema (see p. 341).
Irregular emphysema. There is scarring and damage affecting the lung parenchyma patchily independent of acinar structure.
The classic Fletcher and Peto studies (Fig. 15.21) show that there is a loss of 50 mL per year in FEV1 in patients with COPD compared with 20 mL per year in healthy people. A recent study has shown a 40 mL loss per year but only in 38% of the patients studied. Biomarkers to indicate the rate of decline have been unhelpful although CC16 (Clare cell secretory protein 16) was shown to be a reasonable indicator in a study.
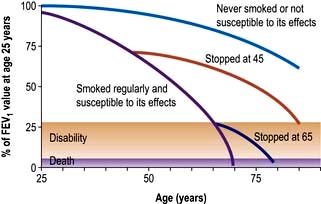
Figure 15.21 Influence of smoking on airflow limitation.
(From Fletcher CM, Peto R. The natural history of chronic airflow abstruction. British Medical Journal 1977; 1:1645.)
Pathogenesis
α1-Antitrypsin deficiency
α1-Antitrypsin (see also p. 341) is a proteinase inhibitor which is produced in the liver, secreted into the blood and diffuses into the lung. Here it inhibits proteolytic enzymes such as neutrophil elastase, which are capable of destroying alveolar wall connective tissue.
More than 75 alleles of the α1-antitrypsin gene have been described. The three main phenotypes are MM (normal), MZ (heterozygous deficiency) and ZZ (homozygous deficiency). About 1 child in 5000 in Britain is born with the homozygous deficiency, but not all develop chest disease. Those who do develop breathlessness under the age of 40 years have radiographic evidence of basal emphysema and are usually, but not always, cigarette smokers. Hereditary deficiency of α1-antitrypsin accounts for about 2% of emphysema cases. A small minority develop liver disease (see p. 341).
Clinical features
Signs
In mild COPD, there may be no signs or just quiet wheezes throughout the chest. In severe disease, the patient is tachypnoeic, with prolonged expiration. The accessory muscles of respiration are used and there may be intercostal indrawing on inspiration and pursing of the lips on expiration (see p. 792). Chest expansion is poor, the lungs are hyperinflated, and there is loss of the normal cardiac and liver dullness.
Diagnosis
This is usually clinical (Table 15.9) and based on a history of breathlessness and sputum production in a chronic smoker. In the absence of a history of cigarette smoking asthma is a more likely explanation unless there is a family history suggesting α1-antitrypsin deficiency.
Table 15.9 Classification of severity of Airflow limitation in COPD (2011)
Gold stage | FEV1 / FVC | % Predicted |
---|---|---|
1. Mild |
<70% |
≥80% |
2. Moderate |
<70% |
<80% |
3. Severe |
<70% |
<50% |
4. Very severe |
<70% |
<30% |
Modified from Global Institute for Chronic Obstructive Lung Disease, 2011. www.goldcopd.com.
Investigations
Lung function tests show evidence of airflow limitation (see Figs 15.7 and 15.12). The FEV1: FVC ratio is reduced and the PEFR is low. In many patients the airflow limitation is partly reversible (usually a change in FEV1 of <15%), and it can be difficult to distinguish between COPD and asthma. Lung volumes may be normal or increased; carbon monoxide gas transfer factor is low when significant emphysema is present.
Chest X-ray is often normal, even when the disease is advanced. The classic features are overinflation of the lungs with low, flattened diaphragms, and sometimes the presence of large bullae. Blood vessels may be ‘pruned’ with large proximal vessels and relatively little blood visible in the peripheral lung fields.
High-resolution CT scans are useful, particularly when the plain chest X-ray is normal.
Haemoglobin level and PCV can be elevated as a result of persistent hypoxaemia (secondary polycythaemia, see p. 404).
Blood gases are often normal at rest, but patients desaturate on exercise. In more advanced cases there is resting hypoxaemia and there may also be hypercapnia.
Sputum examination is not useful in ordinary cases. Strep. pneumoniae and H. influenzae are the only common organisms to produce acute exacerbations. Occasionally, Moraxella catarrhalis may cause infective exacerbations.
Electrocardiogram is often normal. In advanced pulmonary hypotension the P wave is tall (P pulmonale) and there may be right bundle branch block and evidence of right ventricular hypertrophy (see p. 764).
Echocardiogram is useful to assess cardiac function where there is disproportionate dyspnoea.
α1-Antitrypsin levels and genotype are worth measuring in premature disease or lifelong non-smokers.
FURTHER READING
Barnes PJ. Small airways in COPD. N Engl J Med 2004; 350:2635–2637.
Niewoehner DE. Outpatient management of severe COPD. N Engl J Med 2012; 362:1407–1416.
Qaseem A, Snow V, Shekelle P et al. Diagnosis and management of stable chronic obstructive pulmonary disease: A practice guideline from the American College of Physicians. Ann Intern Med 2007; 147:633–638.
Management
See Figure 15.22 for management strategies.
Smoking cessation
The single most useful measure is to persuade the patient to stop smoking. Even in advanced disease this may slow down the rate of deterioration and prolong the time before disability and death occur (see Fig. 15.22). Smoke from burning biomass fuels in poorly ventilated homes should also be reduced or get to non-smoking facilities if possible.
Drug therapy
This is used both for the short-term management of exacerbations and for the long-term relief of symptoms. Many of the drugs used are similar to those used in asthma (see p. 829).
Bronchodilators
β-Adrenergic agonists. Many patients with mild COPD feel less breathless after inhaling a β-adrenergic agonist such as salbutamol (200 µg every 4–6 hours). In more severe airway limitation (moderate and severe COPD), a long-acting β2 agonist should be used, e.g. formoterol 12 µg powder inhaled twice daily or salmeterol 50 µg twice daily. Indacaterol 150–300 µg daily is also effective.
Antimuscarinic drugs. More prolonged and greater bronchodilatation is achieved with antimuscarinic agents: tiotropium (long-acting) (18 µg daily), ipratropium (40 µg four times daily) or oxitropium (200 µg twice daily). Tiotropium improves function and quality of life but does not affect the decline in FEV1. Compound bronchodilators, a selective β2 agonist and an antimuscarinic agent, are used. If patients find inhalers difficult to use, spacer devices improve delivery. Objective evidence of improvement in peak flow rate or FEV1 may be small and decisions to continue or stop therapy are based mainly on the patient’s reported symptoms.
Theophyllines. Long-acting preparations of theophylline are of little benefit in COPD.
Oxygen therapy
Two controlled trials (chiefly in males) have shown improved survival with the continuous administration of oxygen at 2 L/min via nasal prongs to achieve an oxygen saturation of greater than 90% for large proportions of the day and night. Survival curves from these two studies are shown in Figure 15.23.
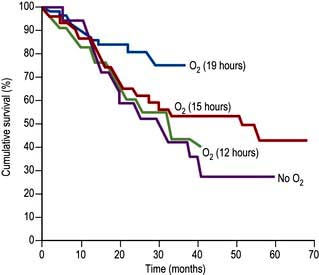
Figure 15.23 Cumulative survival curves for patients receiving oxygen. Oxygen doses are in hours per day.
PaO2 of <7.3 kPa (55 mmHg) when breathing air. Measurements should be taken on two occasions at least 3 weeks apart after appropriate bronchodilator therapy (Box 15.1).
PaO2 7.3–8 kPa with secondary polycythaemia, nocturnal hypoxaemia, peripheral oedema or evidence of pulmonary hypertension.
Carboxyhaemoglobin of <3% (i.e. patients who have stopped smoking).
Box 15.1
Guidelines for domiciliary oxygen (Royal College of Physicians, 1999)
Chronic obstructive pulmonary disease with PaO2 <7.3 kPa when breathing air during a period of clinical stability
Chronic obstructive pulmonary disease with PaO2 7.3–8 kPa in the presence of secondary polycythaemia, nocturnal hypoxaemia, peripheral oedema or evidence of pulmonary hypertension
Severe chronic asthma with a PaCO2 <7.3 kPa or persistent disabling breathlessness
Diffuse lung disease with PaO2 <8 kPa and in patients with PaO2 >8 kPa with disabling dyspnoea
Cystic fibrosis when PaO2 <7.3 kPa or if PaO2 7.3–8 kPa in the presence of secondary polycythaemia, nocturnal hypoxaemia, pulmonary hypertension or peripheral oedema
Pulmonary hypertension without parenchymal lung involvement when PaO2 <8 kPa
Neuromuscular or skeletal disorders, after specialist assessment
Obstructive sleep apnoea despite continuous positive airways pressure therapy, after specialist assessment
Pulmonary malignancy or other terminal disease with disabling dyspnoea
Heart failure with daytime PaO2 <7.3 kPa (on air) or with nocturnal hypoxaemia
Nocturnal hypoxia
Inhibition of intercostal and accessory muscles in REM sleep
Shallow breathing in REM sleep, which reduces ventilation, particularly in severe COPD
An increase in upper airway resistance because of a reduction in muscle tone.
Additional measures
Vaccines. Patients with COPD should receive a single dose of the polyvalent pneumococcal polysaccharide vaccine and yearly influenza vaccinations.
α1-Antitrypsin replacement. Weekly or monthly infusions of α1-antitrypsin have been recommended for patients with serum levels below 310 mg/L and abnormal lung function. Whether this modifies the long-term progression of the disease remains to be determined.
Heart failure should be treated (p. 718).
Secondary polycythaemia requires venesection if the PCV is >55%.
Pulmonary hypertension can be partially relieved by the use of oral β-adrenergic agonists such as salbutamol (4 mg three times daily), but the long-term value is unknown.
The sensation of breathlessness can be reduced by either promethazine 125 mg daily or dihydrocodeine 1 mg/kg by mouth. Although opiates are the most effective treatment for intractable breathlessness they depress ventilation and carry the risk of increasing respiratory failure.
Antileukotriene agents have been tried but are rarely effective (p. 831).
Air travel. Commercial aircraft are pressurized to the equivalent of 2000–2400 m altitude. In healthy people, this causes PaO2 to fall from 13.5 to 10 kPa, causing a trivial 3% drop in oxygen saturation, but patients with moderate COPD may desaturate significantly. The desaturation associated with air travel can be simulated by breathing 15% oxygen at sea level. Patients whose saturation drops below 85% within 15 minutes should be advised to contact their airline to request supplemental oxygen during flight.
Surgery. Some patients have large emphysematous bullae which reduce lung capacity. Surgical bullectomy can enable adjacent areas of collapsed lung to re-expand and start functioning again. In addition, carefully selected patients with severe COPD (FEV1 <1 L) have been treated with lung volume reduction surgery. This increases elastic recoil, which reduces the expiratory collapse of the airway and decreases expiratory airflow limitation. It also enables the diaphragm to work at a better mechanical advantage. Initial studies suggested that ventilation was improved and patients felt less breathless, although mortality was unchanged. However, a controlled trial in severe emphysema found increased mortality and no improvement in the patients’ condition. Single lung transplantation (see p. 822) is used for end-stage emphysema, with 3-year survival rates of 75%. The principal benefit is improved quality of life but it does not extend survival.
Acute respiratory failure in COPD
Oxygen therapy. COPD is by far the commonest cause of respiratory failure (Fig. 15.24). In managing respiratory failure the main goal is to improve the PaO2 by continuous oxygen therapy. In type II respiratory failure the PaCO2 is elevated and the patient is dependent on hypoxic drive. In this setting, giving additional oxygen will nearly always cause a further rise in PaCO2. Small increases in PaCO2 can be tolerated but the pH should not be allowed to fall below 7.25; if it does, increased ventilation must be achieved either by artificial ventilation or by using a respiratory stimulant. In COPD exacerbations, a fixed-percentage mask (Venturi mask; Fig. 15.25) is used to deliver controlled concentrations of oxygen. Initially, 24% oxygen is given, and the concentration of inspired oxygen can be gradually increased provided the PaCO2 does not rise unacceptably.
Removal of retained secretions. Patients should be encouraged to cough up secretions. Physiotherapy is helpful. If this fails, secretions can be aspirated by bronchoscopy or via an endotracheal tube
Respiratory support (see p. 895). Non-invasive ventilatory techniques can be very helpful in avoiding the need for endotracheal intubation. The best current technique uses tight-fitting facial masks to deliver bilevel positive airway pressure ventilatory support (BiPAP). Assisted ventilation with an endotracheal tube is occasionally used for patients with COPD with severe respiratory failure but only when there is a clear precipitating factor and the overall prognosis is reasonable. Assessing the likelihood of reversibility in an acute setting can present a difficult ethical problem.
Respiratory stimulants. Respiratory stimulants such as doxapram were widely used in the past, but have fallen out of favour due to improvements in non-invasive ventilation.
Corticosteroids, antibiotics and bronchodilators should be administered in the acute phase of respiratory failure but decisions on long-term use should wait until the patient has recovered (see above).
Prognosis of COPD
Predictors of a poor prognosis are increasing age and worsening airflow limitation, i.e. decreasing FEV1. A predictive index (BODE: Body mass index, degree of airflow Obstruction, Dyspnoea and Exercise capacity) is shown in Box 15.2.
Obstructive sleep apnoea
OSA affects 1–2% of the population and occurs most often in overweight middle-aged men. It can occur in children, particularly those with enlarged tonsils. The major symptoms and their frequency are listed in Table 15.10. During sleep, activity of the respiratory muscles is reduced, especially during REM sleep when the diaphragm is virtually the only active muscle. Apnoeas occur when the airway at the back of the throat is sucked closed when breathing in during sleep. When awake, this tendency is overcome by the action of opening muscles of the upper airway, the genioglossus and palatal muscles, but these become hypotonic during sleep (Fig. 15.26). Partial narrowing results in snoring, complete occlusion causes apnoea and critical narrowing causes hypopnoeas. Apnoea leads to hypoxia and increasingly strenuous respiratory efforts until the patient overcomes the resistance. The combination of the central hypoxic stimulation and the effort to overcome obstruction wakes the patient from sleep. These awakenings are so brief that the patient remains unaware of them but may be woken hundreds of times per night leading to sleep deprivation, especially a reduction in REM sleep, with consequent daytime sleepiness and impaired intellectual performance. Contributory factors are obesity, narrow pharyngeal opening and co-existent COPD.
(%) | |
---|---|
Loud snoring |
95 |
Daytime sleepiness |
90 |
Unrefreshed sleep |
40 |
Restless sleep |
40 |
Morning headache |
30 |
Nocturnal choking |
30 |
Reduced libido |
20 |
Morning drunkenness |
5 |
Ankle swelling |
5 |
Correctable factors occur in about one-third of cases and include:
Encroachment on pharynx – obesity, acromegaly, enlarged tonsils
Nasal obstruction – nasal deformities, rhinitis, polyps, adenoids
Respiratory depressant drugs – alcohol, sedatives, strong analgesics.
Diagnosis
Relatives often provide a good history of the snore-silence-snore cycle. The Epworth Sleepiness Scale (Table 15.11) helps discriminate OSA from simple snoring. The diagnosis is supported by overnight pulse oximetry performed at home. Characteristically, oxygen saturation falls in a cyclical manner giving a sawtooth appearance to the tracing. If oximetry is negative or equivocal, inpatient assessment with oximetry and video-recording is indicated, preferably in a room specifically adapted for sleep studies. Full polysomnographic studies are rarely needed for clinical diagnosis but are useful in research labs. These involve oximetry, direct measurements of thoracic and abdominal movement to assess breathing, and electroencephalography to record patterns of sleep and arousal. Some centres also measure oronasal airflow.
How likely are you to doze off or fall asleep in the following situations, in contrast to just feeling tired? This refers to your usual way of life in recent time. Even if you have not done some of these things recently, try to work out how they would have affected you. Use the following scale to choose the most appropriate number for each situation. |
|
0 = would never doze |
|
1 = slight chance of dozing |
|
2 = moderate chance of dozing |
|
3 = high chance of dozing |
|
Situation |
Chance of dozing |
Sitting and reading |
_______________ |
Watching TV |
_______________ |
Sitting and inactive in a public place (theatre or meeting) |
_______________ |
As a passenger in a car for an hour without a break |
_______________ |
Lying down to rest in the afternoon when circumstances permit |
_______________ |
Sitting and talking to someone |
_______________ |
Sitting quietly after lunch (without alcohol) |
_______________ |
In a car, while stopped for a few minutes in the traffic |
_______________ |
TOTAL |
_______________ |
Normal 5 ± 4 |
The diagnosis of sleep apnoea/hypopnoea is confirmed if there are more than 10–15 apnoeas or hypopnoeas in any 1 hour of sleep. There is, however, overlap with central sleep apnoea (see p. 1069).
Bronchiectasis
Aetiology
Cystic fibrosis is the most common cause in developed countries. For other causes see Table 15.12.
Investigations
Chest X-ray may show dilated bronchi with thickened bronchial walls and sometimes multiple cysts containing fluid, but can be normal.
High-resolution CT scanning (see p. 791) reveals thickened, dilated bronchi and cysts at the end of the bronchioles (Fig. 15.27). Characteristically the airways are larger than their associated blood vessels.
Sputum examination and culture are essential for adequate treatment. The major pathogens are Staph. aureus, Pseudomonas aeruginosa, H. influenzae and anaerobes. Other pathogens include Strep. pneumoniae and Klebsiella pneumoniae. Aspergillus fumigatus can be isolated from 10% of sputum specimens in cystic fibrosis, but the role of this organism in producing infection is uncertain. Mycobacterium avium-intracellulare complex (MAI) is being increasingly found.
Sinus X-rays. 30% of bronchiectasis patients also have rhinosinusitis.
Serum immunoglobulins. Up to 10% of adults with bronchiectasis have antibody class or subclass deficiency (mainly IgA). Some have normal antibody class levels but fail to respond to respiratory pathogens. Responses to Hib and pneumococcal vaccines may be impaired.
Sweat electrolytes – if cystic fibrosis is suspected (see p. 821).
Mucociliary clearance (nasal clearance of saccharin). A 1 mm cube of saccharin is placed on the inferior turbinate and the time to taste measured (normally <30 minutes).
Treatment
Cystic fibrosis
In cystic fibrosis (CF) there is an alteration in the viscosity and tenacity of mucus produced at epithelial surfaces. The classical form of the syndrome includes bronchopulmonary infection and pancreatic insufficiency, with a high sweat sodium and chloride concentration. It is an autosomal recessive inherited disorder with a carrier frequency in Caucasians of 1 in 22 (see p. 43). There is a gene mutation on the long arm of chromosome 7 in the region of 7q31.2. The commonest abnormality is a specific deletion at position 508 in the amino acid sequence [ΔF508] – which results in a defect in a transmembrane regulator protein (see p. 44). This is the cystic fibrosis transmembrane conductance regulator (CFTR), which is a critical chloride channel (Fig. 15.28). The mutation alters the secondary and tertiary structure of the protein, leading to a failure of opening of the chloride channel in response to elevated cyclic AMP in epithelial cells. This results in decreased excretion of chloride into the airway lumen and an increased reabsorption of sodium into the epithelial cells. With less excretion of salt there is less excretion of water and increased viscosity and tenacity of airway secretions. A possible reason for the high salt content of sweat is that there is a CFTR-independent mechanism of chloride secretion in the sweat gland with an impaired reabsorption of sodium chloride in the distal end of the duct. Many genetic variants are known. The frequency of λF508 mutation in CF is 70% in the USA and UK, under 50% in southern Europe and 30% in Ashkenazi families. A further mutation G551D-CFTR reaches the cell surface but fails to open VX-770 allows it to open momentarily (see p. 811)
Clinical features
Gastrointestinal effects
About 85% of patients have symptomatic steatorrhoea owing to pancreatic dysfunction (see p. 366). Children may be born with meconium ileus owing to the viscid consistency of meconium in CF; later in life they may develop the meconium ileus equivalent syndrome, a form of small intestinal obstruction unique to CF. Cholesterol gallstones occur with increased frequency. Cirrhosis develops in about 5% of older patients and there are increased incidences of peptic ulceration and gastrointestinal malignancy.
Diagnosis
The diagnosis of CF in older children and adults is based on the clinical history and:
a family history of the disease
a high sweat sodium concentration over 60 mmol/L (meticulous technique by laboratories performing regular sweat analysis is essential, but the test is still difficult to interpret in adults)
blood DNA analysis of gene defect
radiology showing features seen in bronchiectasis (see p. 819)
absent vas deferens and epididymis
blood immunoreactive trypsin levels – these are not useful diagnostically but may be useful in screening.
Treatment
General care should include stopping smoking, vaccination with influenza and pneumococcal vaccines and pulmonary rehabilitation (p. 817).
Oxygen therapy should be given as necessary (Box 15.1).
Antibiotic treatment for respiratory infections is as described under bronchiectasis on page 820.
Seventy per cent of adults with CF have Pseudomonas infection in their sputum. Nebulized anti-pseudomonal antibiotic therapy improves lung function and decreases the risk of infective exacerbations and hospitalization.
Drug therapy: β2 agonists (p. 830) and inhaled corticosteroids (p. 831) may provide symptomatic relief but have no effect on long-term survival.
Airway clearance. Inhalation of recombinant DNAse (dornase alfa 2.5 mg daily) has been shown to improve FEV1 by 20% in some patients. Hypertonic saline by inhalation (in concentrations of up to 7%) gives short-term benefit. Amiloride, which inhibits sodium transport, has been used but no overall benefit has been shown in meta-analysis. Acetylcysteine has been shown in vitro to liquefy CF sputum by cleaving disulfide bonds in mucus glycoproteins but clinical studies have been disappointing.
Non-invasive ventilation (p. 895) improves symptoms in chronic respiratory failure but there is no evidence of a survival benefit. It acts as a bridge to lung transplantation (p. 822).
Treatment for pancreatic insufficiency (p. 366) and malnutrition (p. 203).
Human experimental studies have been conducted on the delivery to the epithelium of the normal CFTR gene using, as a vector, a replication-deficient adenovirus containing normal human CFTR complementary DNA which is trophic for epithelial cells. Gentamicin can suppress premature termination codons, and nasal administration has been shown to correct the physiological abnormality. These studies are in their early stages.
Chronic cough (see p. 798)
Pathological coughing results from two mechanisms:
Stimulation of sensory nerves in the epithelium by secretions, foreign bodies, cigarette smoke and tumours
Sensitization of the cough reflex with abnormal increase in the sensitivity of the cough receptors.
ENT examination (p. 1051) and sinus CT for post-nasal drip
Lung function tests and histamine bronchial provocation testing (p. 829) for cough-variant asthma
Ambulatory oesophageal ph monitoring and manometry for oesophageal reflux
Fibreoptic bronchoscopy for inhaled foreign body or tumour
ECG, echocardiography and exercise testing and impedance for cardiac causes
Symptomatic management of unexplained cough can be difficult. Morphine depresses the sensitized cough reflex but its unwanted effects limit its long-term use. Dihydrocodeine linctus may help some patients. Demulcent preparations and cough sweets only provide temporary relief. Patients who cough while taking ACE inhibitors should switch to an angiotensin-II receptor antagonist, e.g. losartan (see p. 719), which does not block bradykinin breakdown.