CHAPTER 89 Physiology of the Oral Cavity
The oral cavity is a complex organ comprising muscle, glands, teeth, and specialized sensory receptors. For most animals, the orosensory and oromotor apparatus is critical for successful defense, reproduction, exploration, nutrition, and vocalization.1 In humans, vocalization has evolved into complex speech production, but other human behaviors depend less on the mouth and tongue than on the eye and hand. In all animals, however, the mouth is essential for the ingestion of nutrients. The incorporation of nutrients by mastication and drinking involves a high degree of coordination within and between different oral motor systems. Chewing requires the reciprocal activation of antagonist trigeminal muscles to open and close the jaws and the tongue to position food between the teeth. A diverse array of highly specialized sensory systems guides these complex oromotor responses and initiates secretion of digestive enzymes. Mechanoreceptors in the tongue, palate, and periodontal ligament (PDL) all contribute to a three-dimensional (stereognostic) perception of the oral cavity.2 The sense of taste serves in both food selection and protection from ingesting potentially toxic substances.
Recent reviews provide comprehensive coverage of specific aspects of oral function including mastication,* swallowing,3,4 oral mechanoreception,5,6 and the sense of taste.7–10 In addition, several recent papers have reviewed oral pain11,12 and taste dysfunction.13
Sensory Function
Oral Somesthesia
Overall, the anterior oral cavity displays greater tactile sensitivity than does the posterior oral structure.1,14 The tip of the tongue is particularly sensitive, with a discriminative capability equivalent to that of the digits (Fig. 89-1). The midline of the palate and tongue are more sensitive than lateral regions. A similar pattern of sensitivity applies to the teeth.15 Adults with complete dentition could detect a 1-g von Frey hair applied to the anterior (midline) teeth but require nearly 10 g to detect stimulation of the first molar. The sensitivity to warm and cold stimuli also varies widely across oral tissues. Sensitivity to warm stimuli is relatively high on the tip of tongue but not particularly so on either the palate or buccolabial surfaces.16,17 In contrast, the sensitivity to a cool stimulus is less differentiated within the oral cavity, and the sensitivity of the tongue tip, palate, and buccolabial surfaces is essentially equal. In general, the sensitivity to cool stimuli is greater compared with warm stimuli.
Recording from single human lingual fibers innervating the anterior tongue18 confirms the small receptive fields and high sensitivity to low-threshold forces observed psychophysically (Fig. 89-2). On the basis of their small receptive fields and low thresholds, lingual fibers could be divided into those innervating the superficial (mucosal) surface of the tongue and those innervating deeper muscle tissue. The majority of the superficial fibers were rapidly adapting—a characteristic in common with other highly sensitive structures used in exploratory activity (e.g., the hand). In contrast, the deeper receptors were all slowly adapting and may provide information about the position of the tongue.
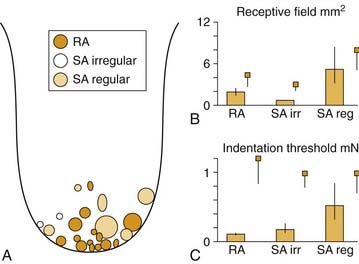
Figure 89-2. Receptive field properties of superficial mechanoreceptive afferents recorded from the human lingual nerve. A, Size and location of receptive fields of three types of mechanoreceptors: rapidly adapting (RA), slowly adapting (SA) regular, and slowly adapting irregular. B, Receptive field area. C, Receptive field threshold. Small squares indicate corresponding data from human median nerve.70 Vertical bars indicate standard error.
(From Trulsson M, Essick GK. Low-threshold mechanoreceptive afferents in the human lingual nerve. J Neurophysiol. 1997;77:737-748.)
Trigeminal endings mediating somesthetic and thermal sensitivity of the tongue and palate range from free nerve endings to an intermediate group of “semi-organized” endings19 to more highly organized endings variously referred to as Krause end bulbs,20 mucocutaneous end organs,19 or coiled terminations.21 All investigators agree that there are no Pacinian corpuscles in the oral mucosa. Based on ultrastructural criteria, Munger22 refers to many highly organized oral mucosa endings as Meissner corpuscles, similar to those found in glabrous skin of the hand. However, despite all this variation in nomenclature, many of the illustrations of the specialized endings are quite similar and show “finely wound nonmyelinated fibers” without a clearly defined capsule.19 Ultrastructural studies further reveal that some of these organized endings in the palate (but not the lingual epithelium) send axonal processes into the overlying epithelial pegs and are associated with Merkel cells.22,23 In the hand, Merkel cells are correlated physiologically with slowly adapting mechanoreceptors; however, a similar correlation has not been made in the palate, and their apparent absence in the lingual epithelium does not preclude slowly adapting mechanoreceptors in this structure (see Fig. 89-2). Thus unlike the hand, a correlation between the morphology of oral receptor endings and their response properties as rapidly or slowly adapting has not been demonstrated.
Mechanoreceptors in the PDL have been studied in some detail (see reviews by Jacobs and Steenberghe24 and Maeda and colleagues25). In addition to detecting forces directed against the teeth, PDL receptors initiate oral reflexes of jaw opening and salivation and, together with receptors in the temporomandibular joint, contribute to interdental discrimination and oral stereognosis.2,24 As many as six varieties of receptor morphology are found in the PDL, ranging from complex Ruffini-like branched endings to free nerve endings.26,27 The cell bodies for PDL receptors are located peripherally in the trigeminal ganglion and centrally in the mesencephalic trigeminal nucleus.28 Mesencephalic trigeminal innervation of the PDL is primarily in the apical region near the root and consists mostly of small, myelinated, Ruffini-like endings.29 Trigeminal ganglion innervation extends from the apical region to the more superficial region and includes small unmyelinated nerve endings.
Both rapidly and slowly adapting mechanoreceptors are found in the PDL, and it is likely that it is the location of the receptor in the ligament that determines its response characteristic. Because the tooth rotates about its fulcrum, forces directed laterally to the crown will translate to greater stretch at the root of the tooth compared with the fulcrum. Thus it is perhaps not surprising that lower-threshold fibers are found near the root and that they tend to be slowly adapting compared with receptors located near the fulcrum.30 In addition, individual Ruffini endings are not uniformly distributed around the tooth and thus display directional sensitivity to the force required to activate them. Recordings from human nerves (microneurography) demonstrate the directional sensitivity of PDL receptors31 (Fig. 89-3) and further indicate mechanical coupling between the teeth. Single fibers respond to stimulation of multiple (adjacent) teeth; however, there is no anatomic evidence that individual fibers innervate multiple teeth.32
The differential innervation of the PDL by the trigeminal ganglion and mesencephalic trigeminal nucleus has functional significance. Mesencephalic receptors are primarily medium and rapidly adapting receptor types, many with directional sensitivity. The central termination of these mesencephalic force detectors includes inhibitory connections to trigeminal jaw closer motoneurons via the supratrigeminal area.33 Thus these receptors serve a protective role in preventing potentially damaging tooth contact during mastication. In contrast, trigeminal ganglion receptors include slowly adapting mechanoreceptors (position detectors) and high-threshold C fibers (nociceptors) in addition to rapidly adapting mechanoreceptors. Because these periodontal receptors from the trigeminal ganglion terminate centrally in the sensory trigeminal complex, the source for the ascending sensory pathway to the thalamus and cortex, they provide information about tooth displacement and dental pain to the forebrain.
Although mechanoreceptors in the PDL are not encapsulated, their response characteristics are influenced by the elastic properties of the ligament. When the attachment of the ligament is compromised (e.g., during periodontitis that loosens the connective attachments of the ligament), a corresponding loss in interdental force discrimination is observed.34 Periodontal receptors also contribute to the regulation of bite force. Individuals with dentures could not bite as hard as normal dentulous subjects and could not perceive variations in their own bite force.2,35 Similar results were obtained by anesthetizing the inferior alveolar nerve.36 In contrast, anesthetizing the temporomandibular joint does not affect bite force discrimination but does impair jaw-positioning performance. Thus different populations of oral receptors may regulate sensing jaw position and controlling bite force during mastication.
Common Chemical Sense
Stimulation of the oral cavity with high concentrations of salts, acids, alkaloids, and other compounds elicits intense taste sensations but also evokes nontaste sensations ranging from stinging and burning to warm, cool, and painful. This sensitivity of the oral cavity, mediated by nonspecialized free nerve endings and shared by all mucosal membranes, is referred to as the common chemical sense or chemesthesis and should not be confused with taste. Although free nerve endings respond to many traditional gustatory stimuli, they typically display a much lower sensitivity. Electrophysiologic recordings from the lingual nerve, for example, indicate that single fibers require concentrations of sodium chloride (NaCl) 1000 times higher than those necessary to elicit a response from a gustatory fiber in the chorda tympani nerve (reviewed by Bryant and Silver37). Much lower concentrations of other types of chemical stimuli (e.g., menthol [10−4]), however, are adequate to elicit a response in trigeminal nerve fibers. The types of chemical stimuli that elicit low-threshold responses in trigeminal fibers suggest that one function of the common chemical sense is to protect the oral cavity. Responses to common chemical stimuli include reflex salivation and coughing that function to diffuse and remove offending stimuli from the mouth. The common chemical sense is not purely protective, however. Spices such as horseradish, ginger, and red pepper are effective stimuli for trigeminal afferent fibers and contribute to the flavor of food. One of the receptors for chemesthetic stimulation was recently cloned.38 A member of the TRP family of G-protein-coupled receptors, the vanilloid receptor termed “VR1” responds to both noxious heat and low concentrations of protons in addition to vanilloid compounds such as capsaicin, found in chili pepper. Stimulation of this receptor results in the opening of a cation channel, thus depolarizing the afferent fiber.
Dental Pain
Persons usually describe dental pain as either dull/burning or sharp.39 Sensations of dull burning pain are associated with stimulation of C fibers terminating in the pulp chamber, whereas sharp “bright” dental pain is associated with A-delta fiber innervation that extends a short distance into the dentinal tubules matrix interposed between the pulp chamber and the enamel covering of the tooth (reviewed in Burgess and colleagues40). Unmyelinated C fibers constitute the majority of pulpal innervation (50% to 75%); however, endings within the pulp chamber may be unmyelinated terminals of A-delta (myelinated) afferent fibers. Polymodal C fibers innervating the pulp chamber respond to thermal stimuli and, in particular, to inflammatory mediators including histamine and bradykinin, endogenous factors associated with pulp pathology. C fibers innervating the pulp chamber contain neuropeptides such as substance P and calcitonin gene-related peptide.41 The peripheral release of these neuropeptides on C fiber activation produces local vasodilation, thus increasing the pressure within the rigid pulp chamber and further augmenting C fiber activation (i.e., peripheral sensitization). The release of substance P in infected teeth has been directly measured in human patients using microdialysis, and patients with irreversible pulpitis had significantly higher levels of substance P in the pulp chamber compared with noninfected teeth.42 Although the release of neuropeptides augments pain, there is evidence that it may also reduce inflammation and promote recovery. In experiments with animals, eliminating the afferent terminal release of neuropeptides by denervating the teeth reduced wound healing after lesions were experimentally induced.43
Sharp pain is mediated by A-delta fibers extending 0.2 to 0.3 mm into the dentinal tubules encasing the pulp chamber.44 These nerve fibers respond to heat, mechanical, and osmotic stimuli applied to the distal end of the dentinal tubules that become exposed to environmental stimuli when the enamel layer is breached.45 Because the dentinal tubules are filled with a fluid, the fluid transmits mechanical, thermal, and osmotic stimuli to the proximal end of the dentinal tubules where the nerve endings are located. This “hydrodynamic” theory of dental pain has gained anatomic, physiologic, and psychophysical support and further offers an explanation of dentinal hypersensitivity. When the dentinal tubules are exposed by a cavity or other lesion, patients report sharp pain in response to innocuous stimuli such as mild temperature or osmotic stimuli (e.g., sweet compounds). However, the theory predicts that if the tubules are covered, thus limiting exposure to environmental stimuli, stimulated pain should be reduced. This had been experimentally assessed in human volunteers in whom a small cavity in a tooth was prepared (in a tooth scheduled for removal) and a conical chamber positioned over the cavity through which regulated air pressure could be delivered.46 Creating a smear layer of amorphous tooth particles in the cavity, or dissolving it away with solvents, controlled the interface between the exposed dentinal tubules and the air pressure stimulus. When the smear layer was intact, covering the dentinal tubules, it took more air pressure to induce the perception of sharp pain than when the smear layer was dissolved.
Central Projections of Trigeminal System
Afferent fibers of the trigeminal nerve enter the brainstem in the pons, bifurcate, and terminate in either the principal sensory nucleus or descend to terminate in the spinal trigeminal complex in the medulla. The bifurcation of the trigeminal nerve at the level of the pons reflects a tendency toward a segregation of function.47 In general, low-threshold mechanoreceptors predominate in the principal trigeminal sensory nucleus, indicative of a tactile discriminative function. In contrast, considerable evidence implicates the subnucleus caudalis in orofacial pain mechanisms, and many neurons in the subnucleus caudalis respond to noxious stimuli applied to the head and neck.11 These neurons include those specifically activated by noxious stimuli (nociceptive-specific neurons) and wide-dynamic-range neurons, responsive to both low- and high-intensity stimulation.
Because the receptive fields for many nociceptive neurons in the subnucleus caudalis are large and include responses to nociceptive stimuli applied to the masticatory muscles, tooth pulp, and temporomandibular joint, a role for these neurons in referred pain has been suggested.48 Anatomic studies confirm that afferent fibers innervating the oral cavity, tooth pulp, oropharynx, temporomandibular joint, masticatory muscles, and superficial skin all converge in the subnucleus caudalis.49,50 In many patients, lesions in mandibular teeth have a high likelihood of producing referred pain to the maxillary region, the cheek, and the ear, in addition to the mandible itself.51 Likewise, lesions in the maxillary teeth are often referred to the mandible, as well as the maxilla, temple, and orbital region.
In addition to subnucleus caudalis, other parts of the sensory trigeminal complex are also involved in trigeminal pain. Nociceptive responses have been obtained from extensive areas of the sensory trigeminal complex, and destruction of the subnucleus caudalis does not prevent all trigeminal pain function (reviewed in Sessle52). Case studies of patients who have undergone trigeminal tractotomy for intractable pain associated with cancer are completely analgesic on the face, but pulpal pain is intact.52 Likewise, when the principal trigeminal nucleus and subnucleus oralis were damaged after a stroke, oral and perioral pain sensitivity was diminished, as well as normal tactile sensitivity from these structures.53
Neurons in both the rostral sensory trigeminal complex (subnucleus oralis) and the subnucleus caudalis may also form a substrate for “central sensitization” in which central neurons in the pain pathway have their response characteristics magnified as a result of peripheral stimulation.54 These changes can last a variable amount of time and potentially contribute to both short-term hyperalgesia and long-term chronic pain. Fundamental to the concept of central sensitization is that neurons initially only responsive to high-threshold (nociceptor) input become responsive to low-threshold, non-nociceptive input. The increased responsiveness is thought to be mediated by A-β (non-nociceptive) input that only becomes functionally active after intense peripheral nociceptor input. One neural mechanism for the nascent response to non-nociceptive input has been studied in great detail. An intense afferent barrage of nociceptor input, following peripheral tissue damage or inflammation, “sensitizes” a central neuron via structural modification of an N-methyl-D-aspartate (NMDA) glutamate receptor. NMDA receptors are voltage sensitive and will not pass current, even in the presence of a ligand, unless the cell is sufficiently depolarized. The central release of a neuropeptide (e.g., substance P) by nociceptor afferents, however, may provide sufficient depolarization to modify NMDA glutamate receptors via intracellular signaling pathways, thereby allowing glutamate released by non-nociceptive (A-β) input to activate central neurons. A-β activation of a central nociceptor thus provides a neural mechanism for allodynia. Similar mechanisms have been demonstrated in the brainstem sensory trigeminal complex and may provide a substrate for chronic oral and facial pain.11 Experimental studies demonstrate that neuropharmacologically blocking NMDA receptors prevents temporomandibular joint or tooth pulp afferents from inducing hyperactivity in central trigeminal neurons (i.e., central sensitization).55,56
Somatosensory information reaches the ventrobasal complex of the thalamus from all major subdivisions of the trigeminal sensory complex.11 Many cells in the ventrobasal complex respond to low-intensity stimulation, indicative of a tactile discriminatory function; however, other neurons require high-intensity stimulation. The small receptive fields of both types of neurons suggest a role in localization. Other nuclei, including the posterior thalamic nuclei and the nucleus submedius, respond preferentially to high-intensity stimulation and may be involved in affective components of pain.57 Both nociceptive and non-nociceptive trigeminally activated neurons from the thalamus project to the somatosensory cortex. Electrophysiologic mapping studies in primates indicate a complex, sometimes discontinuous somatotopic map of the facial and oral region.58 In general, the face is represented medially on the cortical surface adjacent to the representation of the hand, with successively lateral representations of the teeth and tongue. Magnetic resonance imaging (MRI) in humans confirms this somatotopic representation.59
Motor Function
Oral motor functions include mastication, swallowing, respiration, and vocalization. This review will focus on mastication and the oral components of swallowing and respiration. One of the dominant concepts in oral motor physiology is central pattern generation. Chewing, swallowing, and breathing are each produced by brainstem central pattern generators that control the fundamental rate and pattern of muscle contractions that define each function. Although sensory pathways from the mouth play an intimate role in oral motor function, fundamental to the concept of central pattern generation is that afferent activity is not necessary to evoke rhythmic activity and does not provide the critical timing information for coordinated motor output.60 Although organized in the brainstem, central pattern generators for chewing, swallowing, and respiration are influenced by descending inputs from virtually all major regions of the neuraxis. Detailed reviews of oromotor central pattern generation can be found in works by Nakamura and Katakura,61 Rekling and Feldman,62 and Jean.3
Fundamental to oral motor function is the complex interplay between behaviors competing for the same muscles. Chewing, swallowing, and respiration all require the coordinated activity of masticatory, lingual, facial, and infrahyoid muscles. Swallowing and respiration further depend on pharyngeal and abdominal muscles. Motor coordination takes place on multiple levels. At a behavioral or molar level, swallowing and respiration must be coordinated to prevent aspiration of food into the airway. How this coordination is achieved is only beginning to be understood but likely involves both interactions between central pattern generators and peripheral feedback. However, individual oral motor functions also require a high level of coordination. Bolus formation during mastication requires the coordinated activity of masticatory, lingual, and facial muscles, muscles innervated by motoneuron groups highly segregated in the brainstem. Although the jaws and tongue can function independently,63 oftentimes the tongue and jaw appear inextricably “linked.”64,65 The nature of this linkage, and whether it relies on interactions between central pattern generators, reflex control, or peripheral mechanical linkage, represents a significant problem in oral motor control.
In addition to the complexity of coordination between functions and coordination between motor groups, another level of complexity in oral motor control can be found within the muscles themselves. Individual masticatory and lingual muscles are not homogeneously functioning units; muscles are oftentimes composed of multiple compartments, with muscle fibers oriented in multiple directions. Thus different parts of a muscle can be more or less active during a given behavior.66 Further adding to the complexity of oral musculature are the multiple isoforms of myosin heavy chain (MHC) proteins that form the contractile elements of the muscle fibers. The differential distribution of MHCs within different muscles and muscle compartments imparts additional degrees of freedom to motor output.
A myriad of “simpler” oral reflexes serve protective functions and contribute to complex rhythmic output. Muscle spindles in jaw-closer muscles, for example, may contribute to load regulation during chewing, and oral reflexes may assist coordination between the jaw and tongue. Autonomic oral reflexes modulate salivation and initiate digestive processes. Several recent reviews of oral reflex function are available.63,67,68
Muscles of Mastication and Reflex Control
The muscles of mastication can be divided into jaw openers and jaw closers. However, human jaw movement is more complex, even during stereotyped rhythmic mastication. During opening, the jaw translates forward; during closing it translates backward.69,70 A given muscle is not isomorphic with a single movement. The masseter, temporalis, medial pterygoid, and superior head of the lateral pterygoid muscle have major jaw-closing (mandible elevation) functions, but contraction of the masseter and lateral pterygoid protrude the mandible, whereas contraction of the temporalis muscle retracts the mandible. Contraction of the anterior belly of the digastric opens retrudes the jaw; contraction of the inferior head of the lateral pterygoid lowers and laterally directs the mandible. Contraction of the mylohyoid muscle also depresses the mandible, as does contraction of the geniohyoid muscle, a muscle innervated by the hypoglossal nucleus.
Individual muscle fibers are physiologically classified as slow (S), fast fatigue resistant (FR), or fast fatigable (FF) and correlate to a high degree with specific isoforms of MHC contractile proteins.71 Thus S fibers express the MHC-I isoform, FR units express the MHC-IIA isoform, and FF units express the MHC-IIB isoform. Individual fibers frequently contain more than one MHC isoform (i.e., hybrid isoforms),72 and overall, masticatory muscles contain a larger proportion of hybrid isoforms compared with limb and trunk muscles.73 Moreover, masticatory muscles express MHC isoforms not found in the limb and trunk, specifically MHC-fetal and MHC-cardiac-α. Thus muscle fibers in jaw-closing muscles (masseter, temporalis, and pterygoid) include numerous hybrids that expressed MHC-I combined with MHC-fetal and MHC-cardiac-α. Muscle fibers in jaw-opening muscles differ from their jaw-closing counterparts.73 Overall, jaw-opening muscles have fewer hybrid fibers and a different distribution and relative weighting of the constitutive isoforms. In the anterior (and posterior) belly of the digastric, mylohyoid, and geniohyoid muscles, there were less MHC-I, MHC-fetal, and MHC-cardiac-α compared with jaw-closing muscles, but more MHC-IIA. The presence of both MHC-fetal and MHC-cardiac-α isoforms may reflect developmental factors, but functionally, hybrid isoforms confer intermediate contraction speeds and thus greater flexibility in motor output.71
Differences in MHC isoform reflect different motor demands on jaw-opening and jaw-closing muscles. The preponderance of slow and hybrid fiber isoforms in jaw-closing muscles reflects a muscle that contracts slowly and requires flexibility against a load during mastication. In contrast, jaw opening is more ballistic and does not normally work against a load. Even the relative distribution of MHC isoforms in different muscle compartments reflects functional specialization. Jaw-closing muscles that are particularly active during mastication (e.g., the anterior temporalis and the deep masseter muscles) have more MHC-I fibers than the posterior temporalis and the superficial masseter, which are less active. The cross-sectional size of individual masticatory motor units also imparts additional degrees of freedom in muscle control. Individual efferent axons from the motor trigeminal nucleus innervate a relatively small area of the target muscle, on the order of 5%, compared with innervation patterns of the limb and trunk, which are much greater.71 Small cross-sectional innervation patterns allow specific areas of a muscle to be differentially controlled.
Jaw-opener and jaw-closer muscles differ in their investment with muscle spindles, and hence reflex function. Muscle spindles, found only in jaw-closer muscles, are involved in multiple reflexes.68,74,75 A “jaw-jerk” reflex that elevates the mandible (jaw-closing) can be elicited by a rapid depression of the mandible (i.e., tapping on the chin). This reflex, analogous to the patellar knee reflex, is mediated by muscle spindle afferents that respond to the rapid stretching of jaw-closing muscles and monosynaptically excite jaw-closing motoneurons. During mastication, these muscle spindle afferents potentially play an important role. During jaw closing against a food bolus, resistance to the load results in intrafusal fibers in the spindle that are (momentarily) shorter than the extrafusal motor fibers in which they are embedded. The consequent stretch of the spindle afferent adds excitatory drive to closer motoneurons, thus compensating for the load. This reflex action is termed the jaw-closing reflex.75
Muscle spindle afferents can also mediate a protective unloading reflex. When the jaws unexpectedly break through hard or brittle food, the rapid downward movement differentially shortens the extrafusal fibers compared with the intrafusal fibers, and muscle spindle afferent activity is decreased, thus producing a “silent period” in the jaw-closer muscle that limits excessive, potentially damaging forces directed against the teeth. These muscle spindle afferents can also indirectly potentiate jaw-opening during the unloading reflex through indirect, polysynaptic pathways. In addition to a monosynaptic excitatory synapse on jaw-closing motoneurons, these afferents can inhibit jaw-opening motoneurons through an inhibitory interneuron (Fig. 89-4). Thus during the jaw-closing phase of mastication, if there is concurrent excitation to jaw-opener motoneurons from a central pattern generator, jaw opening could be disinhibited by a lack of muscle spindle afferent input during unloading, thereby allowing the background excitation to dominate jaw-opener motoneuron activity. Damaging occlusal forces would thus be mitigated by a simultaneous lack of excitation to jaw closers and released excitation to jaw openers (see Fig. 89-4). The cell bodies for muscle spindle afferent fibers are located centrally in the mesencephalic trigeminal nucleus. Monosynaptic projections to jaw-closer motoneurons are well characterized; however, the location of inhibitory interneurons to jaw-opener motoneurons is more speculative.76
Jaw-opening muscles do not have muscle spindles; thus during the jaw-closing phase of mastication, the corresponding lengthening of the jaw-opener muscles does not itself provide an afferent signal for a reciprocal reflex. However, stimulation of mechanoreceptors located in the PDL, tongue, and other soft tissues of the mouth can initiate reflexive jaw opening, at least in many nonprimate mammals.68,74,75 The jaw-opening reflex is, at a minimum, disynaptic through neurons in the trigeminal sensory complex and may well involve additional interneurons (Fig. 89-5). Although the reflex can be elicited by non-noxious stimulation, it is generally thought to serve a protective function by protecting soft tissues (e.g., the tongue) against potentially damaging occlusal forces. The existence of a jaw-opening reflex in humans is still in doubt. Although it cannot be as readily demonstrated with sensory stimuli sufficient to produce it in experimental animals, robust electrical stimulation delivered to the upper lip produces electromyographic (EMG) activity in the anterior digastric muscle of humans.76 It is of long latency, consistent with a polysynaptic substrate as suggested by animal studies.
Lingual Muscles and Reflexes
The tongue is composed of both intrinsic and extrinsic muscles innervated by the hypoglossal nerve (reviewed in Travers77). Extrinsic lingual muscles include the major tongue protruder muscle, genioglossus, major tongue retractor muscles, styloglossus and hyoglossus, as well as palatoglossus muscle. Intrinsic muscles of the tongue consist of the vertical, transverse, superior, and inferior longitudinal muscles. The geniohyoid often functions with the lingual muscles during tongue protrusion.78,79 Most lingual movements involve both extrinsic and intrinsic muscles. The hydrostatic model of lingual function, in which the tongue is modeled as a closed bag, postulates that during tongue protrusion by contraction of the extrinsic genioglossus and geniohyoid muscles, the tongue is further lengthened by the simultaneous contraction of the intrinsic vertical and horizontal intrinsic muscles.80 Likewise, shortening of the tongue during retraction is augmented by contraction of the longitudinal muscles together with the extrinsic hyoglossus and styloglossus muscles. Coactivation of different combinations of intrinsic muscles can curl or deviate the tongue.
Expression of different MHC isoforms varies across different human lingual muscles.73,81,82 Intrinsic muscles of the anterior tongue have a large proportion of type MHC-IIA fast fibers in contrast to the posterior tongue, in which MHC-I (slow) and hybrid MHCs predominate. The geniohyoid, as with other suprahyoid muscles, has a large proportion of MHC-I fibers. The distribution of type II (fast) fibers in the anterior tongue is consistent with a role in fast, flexible movements compared with posterior tongue activity.
Although lingual muscles contain muscle spindles,63,83,84 it is unclear whether there are any monosynaptic inputs from sensory afferents onto hypoglossal motoneurons (reviewed in Travers77). Rather, muscle spindle afferents travel in the ansa cervicalis and hypoglossal nerve and terminate in either the sensory trigeminal complex or the nucleus of the solitary tract. Electrical stimulation of the hypoglossal nerve elicits synaptic responses in hypoglossal motoneurons, as well as facial85 and trigeminal motoneurons.86 Lingual reflexes can also be elicited by stimulation of virtually any of the afferent nerves innervating the oral cavity. Depending on the site of stimulation, either a protrusive or retractive movement of the tongue is produced. An overview by Lowe87 on the clinical significance of lingual reflexes emphasizes a protective role, either for the tongue itself during mastication or for the airway during swallowing.
Compounding the complexity of interpreting lingual reflexes are observations that reflex excitation of the tongue rarely influences a single lingual muscle, and contraction of a single lingual muscle can move the tongue in more than one plane.88 For example, although a primarily retrusive movement of the tongue is produced by electrical stimulation of the lingual nerve, both protruder and retractor hypoglossal motoneurons are excited. Electrical stimulation of the glossopharyngeal nerve that innervates mechanoreceptors on the posterior aspect of the tongue and oropharynx also elicits tongue movement. Similar to the lingual nerve, stimulation of the glossopharyngeal nerve excites both protruder and retractor motoneurons, and the movement of the tongue is primarily retrusive. The simultaneous activation of the glossopharyngeal nerve afferent fibers by electrical stimulation, however, may mask a more complex reflex organization. Lowe has suggested that stimulation of lingual receptors innervated by the glossopharyngeal nerve elicits a primarily retrusive movement of the tongue, in contrast to the lingual protrusion produced by stimulating pharyngeal regions innervated by the glossopharyngeal nerve.63 Thus both lingual and glossopharyngeal reflexes may protect the tongue during the occlusal phase of mastication with a retrusive movement.
In contrast, electrical stimulation of the superior laryngeal nerve that innervates laryngeal mechanoreceptors depolarizes protruder motoneurons and produces a protrusive action of the tongue. Thus mechanoreceptors in the oropharynx and larynx innervated by the superior laryngeal and the glossopharyngeal nerve may preserve airway patency during a swallow with a protrusive tongue movement. Lingual reflexes also play a protective role in respiration. When normal respiration is impeded by hypoxia, a normal breathing pattern, eupnoea, is replaced by gasps.88,89 Gasps are associated with the coactivation of lingual protruder and retractor muscles that enlarge the upper airway.90–92
Jaw-Tongue Reflexes
Oromotor reflexes can involve multiple motor systems. Electrical stimulation of either the masseteric or anterior digastric nerves, for example, suppresses genioglossus activity, suggesting that proprioceptive or nociceptive signals from the trigeminal musculature inhibit lingual protrusion.93 In contrast, passively depressing the mandible (in cats) excites the genioglossus muscle, suggesting that lingual protrusion may be reflexively assisted during jaw opening when the tongue is not subject to occlusal force.94 Further evidence that masticatory muscle proprioceptive afferents influence hypoglossal motoneuron activity comes from experimental lesions of Probst’s tract. Ishiwata and colleagues95 showed that such lesions, which destroy descending mesencephalic projections, suppressed hypoglossal activity induced by passive jaw opening but left intact hypoglossal activity induced by stimulating a cutaneous oral sensory nerve. A jaw-tongue reflex in humans may also be mediated by masticatory muscle proprioceptive afferents.96 Stimulation of the hypoglossal nerve, which contains some afferent fibers, inhibited the masseteric (jaw-closing) reflex.86
Autonomic Reflexes
In addition to somatomotor reflexes, stimulation of the oral cavity elicits numerous autonomic responses. Gustatory and mechanical stimuli are highly effective in eliciting the flow of saliva during mastication.97 The stimulation of receptors in the PDL may be one source for reflex salivation. In both rabbits and humans, there is a high correlation between parotid flow and mandibular movement, especially on the working, ipsilateral side. In humans, selective anesthetization of the nerves innervating the PDL significantly reduced the amount of saliva elicited from crushing a food stimulus held between the teeth.98
Both location and stimulus modality influence the release of saliva.97,99 Stimulating the anterior part of the tongue is most effective for evoking salivation from the sublingual and submandibular glands, but posterior tongue stimulation is more effective for producing parotid gland flow. Aversive gustatory stimuli such as (sour) acids or (bitter) quinine hydrochloride are more effective for eliciting saliva than stimulation with weak salt or sucrose solutions. In animal experiments, sweet stimuli were the most effective stimuli for the release of the enzyme amylase from the parotid gland.100
Mechanical and chemical stimulation of the oral cavity also initiate the release of digestive enzymes. These cephalic phase responses include the release of gastric acid, insulin, glucagon, and pancreatic polypeptide.101,102 An increase in gastric motility and emptying of the gallbladder also occurs. Although cephalic phase insulin release (CPIR) is a highly variable response not occurring in all individuals, insulin levels rise, on average, 25% above baseline within 2 minutes of oral stimulation. This release is neurally mediated because it does not occur in the absence of an intact vagus nerve.103 In animal studies, sweet stimuli—particularly glucose—are most effective in triggering CPIR,104,105 but are somewhat less effective in humans (discussed by Teff102). Rather, “palatable” stimuli appear more effective in general. Although cephalic phase insulin release accounts for perhaps only 1% of the total insulin release associated with a meal, this amount underestimates its potential importance in glucose metabolism. Experimental studies in which CPIR is bypassed by intragastric infusions can result in both hyperinsulinemia and hyperglycemia.106,107 One possible mechanism for this is that vagally mediated insulin release acts as a signal on hepatic receptors to further regulate glucose metabolism; that is, it is acting as a signal to initiate metabolic events rather than simply to convert glucose.102 Other cephalic phase responses represent larger fractions of total meal responses. In humans, cephalic phase gastric acid secretion can reach 50% of total meal release, antral motor activity can reach 70% of that achieved during a meal, and gallbladder emptying can reach 50%.101
Although cognitive factors and other sensory stimuli such as sight and sound can elicit cephalic phase responses, oral stimuli are usually the most effective. Oral stimuli signals carried by the gustatory and trigeminal nerves influence preganglionic parasympathetic vagal neurons located in the dorsal motor nucleus of the vagus.108 Oropharyngeal receptors innervated by the superior laryngeal nerve may also influence digestive functions.108 There is increased diuresis in response to drinking a saline solution as compared with the intragastric infusion of the same volume of fluid.109
Mastication
Food consumption through the oral cavity can be characterized as a series of stages or phases (Fig. 89-6). Different stages of ingestion have been defined by placing small metal markers in the jaws, hyoid, and tongue. These markers can be detected with high-speed cine-fluorographic techniques, allowing the movements of the internal oral apparatus to be monitored during the entire ingestive sequence of the awake preparation. The division of feeding into five dynamic stages by Hiiemae and Crompton64 is indicated on the second tier of Figure 89-6. The first stage of putting food into the mouth (ingestion) is followed by intraoral transport and the positioning of food between the molars (second stage) for mastication (third stage). Intraoral transport to the back of the tongue (fourth stage) initiates deglutition (fifth stage). The duration of each stage of feeding is highly species specific and variable, depending on what is being ingested. Fluid consumption does not require mechanical breakdown by mastication and thus has only three stages. In humans, drinking uses the same muscles as mastication, but the coupling among the facial, trigeminal, and lingual muscles is different. The orbicularis oris muscle contracts to form a tight seal during human drinking (sucking) but relaxes during mastication.
The movements of mastication can be further subdivided. Kinematic measurements during mastication indicate that rhythmic masticatory movements of solid food typically involve several distinct components.110,111 Beginning the masticatory cycle with an open mandible, the jaw closes rapidly and then more slowly. The transition from fast closure to slow closure occurs when the teeth make contact with solid food and is thought to involve sensory feedback from the PDL. More detailed analysis of the opening phase of mastication indicates additional complexity. After the slow-closure phase, when the teeth make maximal intercuspation, the masticatory cycle continues with a slow-opening phase followed by a fast-opening phase.
Feeding sequences analyzed using combined sirognathography and electromyography or video-fluorography present a modified picture of the human ingestive sequence.112,113 Human jaw movements associated with eating natural foods do not show obvious changes in the rate of opening and closing during rhythmic mastication. Thus the stages of fast closing, slow closing, and slow opening were not evident as in animal studies. Nevertheless, the ingestive sequence could be divided into three stages, beginning with biting and transport of the bolus to the molars, chewing, and “clearance” (swallowing). A distinct stage from chewing to bolus formation for swallowing was not obvious, and swallowing occurred during mastication and as a terminal event.
Although mastication involves coordinated activity of the jaws, hyoid apparatus, and tongue,64 the majority of electromyographic studies of mastication have focused on the jaw musculature. Jaw opening during mastication is associated with activity in the anterior digastric muscles and the inferior head of the lateral pterygoid muscle.111,114 The closing phase of mastication begins with contraction of the masseter muscle, followed by the temporalis, medial pterygoid, and superior head of the pterygoid, which are recruited during the power stroke (slow closure). Food is typically chewed unilaterally. Although the trigeminal musculature is bilaterally activated during mastication, the ipsilateral (working) side is active earlier.
Food consistency is one factor affecting the masticatory rhythm. In a study of the effects of hardness on chewing, Plesh and colleagues115 observed that most subjects chewed hard gum at a slightly slower rate than soft gum. The decreased frequency of chewing was associated with significantly longer opening and occlusal phases of chewing rather than with the closing phase, despite the significantly greater EMG activity in the masseter muscle. Age is another factor that affects the masticatory rhythm.116 Older subjects chewed at the same frequency as younger subjects (approximately 1.4 Hz), but the structure of the rhythm was different. The older subjects opened and closed their mouths at a slower velocity but achieved the same overall chewing rate by not opening their mouths as far. Movement irregularities during chewing were also observed during the jaw-opening and jaw-closing phases of mastication in patients diagnosed with temporomandibular pain.117 Unlike the smooth, uninterrupted alteration between opening and closing seen in healthy persons, patients with temporomandibular pain frequently started reopening their mouths during the closing phase of mastication or reclosed during the opening phase.
Experimental studies indicate that the masticatory rhythm is centrally programmed; that is, a peripheral stimulus is not necessary to initiate the masticatory rhythm, nor is feedback from the active muscles necessary to sustain the response.114 Fictive mastication evoked by central stimulation in a paralyzed experimental preparation indicates that neither the afferent limb of the jaw-opening reflex nor that of the jaw-closing reflex is necessary to generate the masticatory rhythm. Thus the alternating activation of a jaw-opening reflex followed by a jaw-closing reflex does not explain the origins of the masticatory rhythm.
Nevertheless, both the jaw-opening and jaw-closing reflexes are functionally entwined in rhythmic oral behavior, and the excitability of these reflexes varies as a function of jaw position during rhythmic opening and closing.118 In general, the jaw-opening reflex is attenuated during rhythmic masticatory movements as compared with a stationary mandible. In particular, low-threshold mechanical stimuli are less effective than high-threshold stimuli in producing a jaw-opening reflex when applied during rhythmic masticatory movements. Thus during the occlusal phase of mastication, a protective jaw-opening reflex can be initiated in the presence of unexpected mechanical forces directed against the teeth or soft tissues, but innocuous mechanical stimulation associated with chewing will not interrupt the masticatory rhythm.
Transection studies relying on electrical stimulation to induce fictive jaw movements localized the central pattern generator for mastication to the medial core of the reticular formation. More recent studies using reversible pharmacologic lesion techniques in awake, freely moving (feeding) animal preparations indicate that a necessary substrate for rhythmic lingual/masticatory movements is in the lateral reticular formation in a region overlapping with substantial populations of preoromotor interneurons.119,120 This region of the brainstem reticular formation is also the target of descending projections from metabolic integrative substrates in the hypothalamus and from the motor cortex.61,121
Although the basic neural circuitry necessary for the rhythmic alternating contraction of jaw-opening and jaw-closing muscles does not require sensory input, intraoral sensory receptors are critical for regulating bite force during mastication. Efficient eating requires that food be reduced in size for swallowing. This requires determining both the hardness and size of the food and correctly positioning food between the occlusal surfaces of teeth. Psychophysical studies in humans indicate that receptors in both the PDL and the temporomandibular joint contribute to the interdental discrimination required during eating.2 The loss of PDL receptors associated with complete dentures results in impaired interdental discrimination, as does anesthetization of the dentition in individuals with natural teeth. Receptors in the temporomandibular joint also contribute to size discrimination in the mouth. When the temporomandibular joint is anesthetized, interdental discrimination decreases.
Oral Phase of Deglutition
After mastication and the intraoral transport of food to the back of the tongue, deglutition is initiated. The oral phase of deglutition consists of an upward movement of the tongue against the soft palate to force the bolus in the direction of the pharynx.3,4 The precise nature of the stimulus that triggers the pharyngeal stage of deglutition is unknown. Both the volume and the rate of bolus accumulation interact to trigger swallows in experimental animals.122 When the rate of licking (intraoral transport) increased in response to increased stimulus delivery, the volume per swallow also increased. Moreover, the physical nature of the bolus can influence the sequence and recruitment of individual muscles involved in the buccal phase of swallowing. In monkeys, the masseter muscle was recruited with the suprahyoid muscles (the anterior digastric, geniohyoid, and mylohyoid) during swallows of solid food, in contrast to fluid swallows.123 Similarly, there is individual variation in the activation sequence of the suprahyoid muscles and the genioglossus muscle during voluntary swallows in humans.124 In summary, the overall movement of a bolus from the dorsal surface of the posterior tongue to the pharynx characterizes the oral phase of swallowing. The precise motor sequence of individual muscles during the oral phase of deglutition can vary, depending on both the individual and sensory characteristics of the bolus. Contact of the bolus with sensory receptors in the oropharynx triggers peristaltic contractions of the pharyngeal musculature.
Like mastication, swallowing can be evoked from electrical stimulation of central structures in the absence of peripheral (muscular) feedback and is thus thought to be controlled by a central pattern generator.3,4 The location of the central pattern generator for swallowing involves the caudal region of the nucleus of the solitary tract and the medullary reticular formation adjacent to the nucleus ambiguus. Cortical pathways that reach these medullary regions through descending pathways mediate voluntary swallowing.
Swallowing takes precedence over both respiration and mastication, causing a brief disruption of rhythm. Respiratory apnea associated with swallowing shows considerable variation across individuals, in one study ranging from 0.61 to 3.83 seconds.125 For some individuals, the apneic period increases with the volume swallowed; for others it decreases. Spontaneous swallows tend to be associated with a shorter apneic period.126 Swallows do not occur randomly throughout respiration. Rather, the majority of swallows occur during expiration or late inspiration and subsequently reset the respiratory rhythm (i.e., there is no modification of the postswallow rhythm to compensate for the swallow-induced apnea).126 Sensory information from the oropharynx could reach the central pattern generator for respiration via the nucleus of the solitary tract to mediate adaptability of the respiratory rhythm to bolus size.125 Alternatively, oropharyngeal afferents could influence the central deglutition substrate to modify respiration126; that is, there could be an interaction between central pattern generators for respiration and swallowing.127
Although swallowing affects the masticatory and licking rhythm in animals, it has only a minimal effect on human mastication.128,129 Swallows most often occur during the early jaw opening phase of mastication and significantly prolong the masticatory cycle. In rodents, the prolonged lick cycle associated with a swallow equals the increased duration of tongue protrudor and retractor muscle contractions; that is, the increased cycle duration was used to accommodate the participation of the tongue in swallowing.79 In addition to interactions between swallowing and mastication, there are also interactions between respiration and mastication. In general, mastication increases respiratory rate while at the same time decreases inspiratory and expiratory time.129 In addition to possible metabolic demands of mastication on respiration (i.e., physical exertion), mastication can increase upper airway resistance by more closely apposing the tongue with the palate. In humans, there is a small but significant tendency for inspiration to occur during masticatory jaw opening.128
Specialized Sensory Systems: Taste
Gustatory Sensitivity
In contrast to the common chemical sense, taste sensations are evoked by relatively low concentrations of chemical stimuli when applied to the specialized gustatory receptor cells. Most investigators agree that there are a discrete number of taste sensations; the most common and easily recognizable are sweet, salty, sour, and bitter. Some contend that there is a fifth taste, umami (heavenly), associated with the taste of monosodium glutamate.130 The sensations of flavor while eating are more diverse than those of pure taste and result from the interaction of taste with the smell and texture of food. The confusion between taste and flavor is well documented in taste and smell clinics. Self-reports of chemosensory dysfunction are highly unreliable; on testing, many individuals reporting loss of taste are frequently found to have impaired olfactory function with no loss in taste sensitivity.131
In addition to a sensory-quality dimension with four distinct tastes, taste stimuli can be categorized on a hedonic dimension with stimuli divided into those that are preferred and those that are disliked. The hedonic attribute of taste is concentration dependent and spans the different submodalities of sweet, sour, salty, and bitter. Low and medium concentrations of salt are preferred, but salt becomes aversive at high concentrations. Although there is a strong genetic component to the hedonic values associated with gustatory stimuli, taste preferences are clearly modifiable by experience.132 Human neonates find bitter solutions strongly aversive, but adults learn to enjoy coffee, alcohol, and other bitter-tasting substances. The hedonic attributes of taste are also subject to metabolic state.
Gustatory Structures
Approximately 7900 gustatory receptors in the human mouth are grouped into distinct subpopulations, defined by their intraoral location, gross morphology, and innervation.133 Gustatory subpopulations differ in sensitivity to chemical stimuli; however, the overall morphology of the taste bud structure within each subpopulation is similar. Each taste bud contains 50 to 150 neuroepithelial cells arranged in spindle-like clusters. Some of the cells within the taste bud extend microvilli into a nonkeratinized “pore” region on the apical surface of the bud. Taste bud cells without microvilli occur both on the periphery of the bud (these are termed marginal cells) and at the base of the taste bud (designated basal cells).134 Receptor cells die and are replaced over a 10- to 14-day period.135 Marginal and basal cells may constitute replacement cells, but the lineage of replacement receptor cells within the taste bud remains controversial. Because taste cells undergo continuous differentiation, disruption of cell division by radiation or other agents can disrupt the sense of taste.
The chorda tympani branch of the facial nerve innervates two to five taste buds on each of approximately 400 fungiform papillae on the anterior aspect of the tongue.136 Fungiform papillae density is greatest at the tip of the tongue and decreases along the dorsal and dorsolateral edges of the tongue. No fungiform papillae are found along the midline. Taste buds on the posterior aspect of the tongue are innervated by the glossopharyngeal nerve and located either in tightly packed clusters distributed along the walls of the trenches surrounding 7 to 10 circumvallate papillae or in the inner folds of the foliate papillae located along the lateral edges of the posterior part of the tongue. The 2400 taste buds in the circumvallate papillae and the 1300 taste buds in the foliate papillae constitute the largest percentage in the human oral cavity. A third large subpopulation of gustatory receptors located in the pharynx and larynx numbers approximately 2400 in humans. These taste buds are not associated with distinct papillae; however, the bud morphology is similar to that found on the tongue. Taste buds of the pharynx are innervated by the glossopharyngeal nerve, and the superior laryngeal nerve branch of the vagus innervates those in the larynx. A smaller subpopulation of taste buds (approximately 400 in humans) is found on the soft palate. These taste receptors, also not associated with distinct papillae, are innervated by the greater superficial petrosal nerve branch of the facial nerve. In rodent species, small populations of taste buds are also found on the buccal wall and the sublingual organ, but these have yet to be characterized in humans.137
The specific pattern of innervation of taste buds by a peripheral nerve has been characterized for the fungiform papillae on the front of the tongue. Single fibers of the chorda tympani nerve synapse on multiple receptor cells within a single taste bud and on receptor cells in adjacent taste buds.138 Likewise, each receptor cell is innervated by more than one fiber of the chorda tympani nerve. Each fiber of the chorda tympani thus receives input from multiple receptor cells, and each bud is innervated by more than one fiber. This pattern of convergence of multiple receptor cells from adjacent taste buds onto a single afferent fiber provides an anatomic substrate for spatial interactions between adjacent taste buds. Successively lower perceptual thresholds in humans can be reached by stimulating multiple adjacent papillae with gustatory stimuli.139
Gustatory Physiology
A common observation in neurophysiologic studies of the gustatory system is that individual neural elements are usually sensitive to a variety of chemical stimuli. Receptor cells, afferent nerve fibers, and central neurons are often responsive to diverse chemical stimuli that elicit qualitatively different sensations in humans. The central issue in gustatory coding has been to determine how broadly responsive neurons code for such distinct sensations as sweet, salty, sour, and bitter. Recent work has focused on organizing gustatory neurons at different levels of the sensory pathway into neuron types.99 Although many neurons are multiply sensitive to different-tasting stimuli, these sensitivities are not random. Neurons are not specifically tuned to a single stimulus, but typically respond best to one of the stimuli representing the four basic taste qualities. The representation (coding) of quality is thought to be mediated by comparison of activity across these classes of neurons.140
Gustatory Transduction
Discoveries made over the past 20 years and, in particular, in the brief time that has elapsed since the beginning of the 21st century, have shed remarkable light on the process by which chemical energy inherent in gustatory stimuli is transformed into cellular changes in the taste receptor cell. These studies on gustatory transduction have been the topic of several recent reviews.8,141–144 Highlights and outstanding issues are emphasized here. Until the mid-1980s, hypotheses regarding gustatory transduction revolved around ill-defined interactions of taste stimuli with membrane-bound receptors. Molecular biologic studies of the past few years have revealed the chromosomal location and specific genetic sequence of receptors that transduce information about carbohydrates, artificial sweeteners, amino acids, and the diverse array of chemicals that humans describe as “bitter.” All these receptors can be classified as G-protein-coupled receptors. Discoveries about these seven-transmembrane segment-spanning proteins have occurred rapidly, and though conforming in some ways to previous ideas, have revealed surprises and ignited controversies. However, well before molecular biologic investigations transformed the field, classic physiologic techniques revealed that one important ionic stimulus, Na+, was transduced in part by transmembrane transport of the stimulus into the cell,145 an event that would be expected to lead directly to depolarization.
The discovery of this mechanism was in conflict with prevailing ideas of stimulus-membrane interactions and the presumed impermeability of the receptor cell to its ligand.146 Nevertheless, on the basis of the suppression of the peripheral nerve response to sodium salts after lingual application of amiloride across a variety of species, ranging from rodents (rat, gerbil, some mice) to primates (rhesus monkey, chimpanzee), a significant portion of the gustatory response to sodium salts appears to be due to entry of the sodium ions through amiloride-sensitive sodium channels located on the apical microvilli of taste receptor cells (reviewed by Halpern147). These sodium channels, which are epithelial Na+ channels (EnaCs), resemble those found in a number of sodium-transporting epithelia such as kidney, lung, and colon (reviewed in Halpern147 and Gilbertson and Margolskee.8 In rodents, gustatory sodium transduction via ENaCs is reflected in the responses of particular subsets of primary afferent neurons, cells with relatively specific responses to sodium salts, as opposed to those cells with a broader sensitivity that extends to nonsodium salts and acids.148
In the rat, the amiloride-sensitive sodium channel has been demonstrated to be essential for behavioral discrimination of sodium from other salts,149–151 but in the human, the significance of this transduction mechanism is not as clear. Although the overall intensity of NaCl is reduced by amiloride,152 when subjects are asked to ascribe intensity ratings of NaCl to particular qualities, the main reductions in intensity are not in the “salty” taste per se but rather in the weaker “sour” taste that this stimulus elicits.153,154 Thus although the ENaC channel is almost certainly present and operational in humans, it may have a more general function in detecting ionic stimuli than in rodents. Indeed, although sodium transduction via the amiloride-sensitive channel is widespread phylogenetically, in addition to the human there are other notable exceptions in which this mechanism appears less important (some mouse strains) or specific (dog).96
Further, although transduction via amiloride-sensitive channels plays an important role, not all sodium responsiveness, even in rodents, relies on this mechanism. A significant portion of the response to NaCl remains after blockade with amiloride, and therefore different transduction mechanisms must mediate the residual, amiloride-insensitive response. However, these mechanisms are not as well understood. Interaction with other apically located cation channels, as well as transport through the tight junctions of the taste bud to interact with basolateral ion channels, has been proposed. Importantly, the amiloride-insensitive mechanism(s) for sodium transduction is (are) not as specific for the sodium cation as transduction via ENaC channels and thus is (are) generally believed to also explain responsiveness to nonsodium salts such as ammonium and potassium chloride (reviewed in Gilbertson and Margolskee8).
Transduction of the class of stimuli that taste sour to humans, namely organic and inorganic acids, is also not as well understood as the amiloride-sensitive mechanism for sodium. A variety of channels that exhibit responsiveness to or modulation by acids have been characterized and are suspected to have some function in taste tissue including members of the ENaC/degenerin superfamily, the acid-sensing channels, the mammalian degenerin-1 channel, and a group of hyperpolarization-activated channels that can be gated by cyclic nucleotides (HCN1 and HCN4) (reviewed in Gilbertson and Margolskee8). However, the contribution that these channels make to the transduction of acid stimuli in the taste system is not clear. An intriguing hypothesis regarding the “proximate” stimulus for acid transduction, however, has recently emerged. It has been proposed that taste cell responses to acids are a result of changes in intracellular pH caused directly by the entry of the acid stimulus into the receptor cell. Measurements of intracellular pH after stimulation with acids show systematic changes in intracellular pH that track concentration for a given acid. These changes are small, serving to keep the cell at a physiologic pH, but are highly reliable and explain a perplexing aspect of the perceptual characteristics of acids. Low pH is clearly the chemical feature that sour stimuli have in common and, for a given acid, sourness is a positive function of concentration and therefore pH. However, it has long been puzzling that, across acids, sourness is not correlated with pH. Rather, at a given pH, organic acids are much sourer. However, the intracellular measurements seem to solve this puzzle: sourness (and the magnitude of the chorda tympani response) is directly correlated with the intracellular drop in pH elicited by different acids. In the case of inorganic acids, hydrogen ions are proposed to enter the cell via ion channels, but organic acids enter the cells as electroneutral molecules by diffusing across the lipid bilayer and then are broken down by intracellular mechanisms.155,156
In contrast to simple ionic stimuli and acids, transduction of carbohydrates, amino acids, and bitter-tasting chemicals occurs via interactions of stimuli with distinct classes of G-protein-coupled receptors. In parallel with the diverse chemical substances that evoke the bitter quality, which include “… amino acids and peptides, flavonoids and terpenes, methylxanthines (caffeine), sulfimides (saccharin), ureas and thioureas (PTC, PROP), and organic and inorganic salts”7 (referencing Rouseff157), transduction of bitter stimuli involves interaction with a large family of receptors, called T2Rs.158,159 The identification of these receptors was aided by the sequencing of the human genome and genetic linkage studies in humans that localized variations in the threshold for a particular bitter substance, propylthiouracil, to a locus in chromosome 5.160 The region identified by the linkage studies was screened, and this resulted in the identification of a family of candidate genes, arranged in groups in chromosome 5 and also in chromosomes 7 and 12, that encoded novel G-protein-coupled receptors. Homologous genes were identified in rodents. Additional investigation revealed that these genes showed a distant resemblance to chemosensory G-protein-coupled receptors found in the vomeronasal organ, as well as metabotropic receptors for the neurotransmitter, glutamate. Significantly, they are expressed specifically in taste buds.158 Furthermore, the various T2R genes colocalized with each other and with a specific G-protein, “gustducin,”158 which was already known to contribute to the ability of rodents to sense bitter stimuli.161 The identification of naturally occurring variations in one T2R family member that correlated with the ability of different mouse strains to taste the bitter stimulus cycloheximide, along with heterologous expression experiments that conferred bitter responsiveness, provided compelling evidence that these genes code bitter receptors.159 The large size of the gene family, the colocalization of the genes, and the specificity of responsiveness when heterologously expressed, prompted a hypothesis about the peripheral neural coding of the bitter quality—namely, the wide array of stimuli that humans perceive as bitter are likewise detected by a large number of distinct bitter receptors, each specifically tuned to particular substances but activating the same set of receptor cells.159 However, fast on the heels of this proposal, a physiologic study using calcium imaging demonstrated that many individual taste receptor cells showed a high degree of specificity for particular bitter stimuli, suggesting that this simple hypothesis might not be adequate.162
Sweet stimuli and L-amino acids also are detected by a group of G-protein coupled receptors, termed T1Rs, although fewer distinct receptors appear to be involved. Both naturally sweet carbohydrates (i.e., mono- and disaccharides) and artificial sweeteners can be detected by a single receptor, a G-protein-coupled dimer of the subunits T1R2 and T1R3.163 Interestingly, one mechanism for sensing amino acids involves one of these same subunits, T1R3, paired with a different one, T1R1.164 A second amino acid taste receptor is a variant of the metabotropic glutamate receptor.165,166 All of these receptors have been shown to be specifically localized to taste buds and to respond to appropriate ligands in heterologous expression systems, providing strong evidence for their gustatory function.
These discoveries about the molecular basis of taste transduction are all exciting, but the definition of receptors for amino acid stimuli may have a special significance for understanding taste function in mammals. Although it is widely agreed that the salty, sour, sweet, and bitter sensations represent distinct, fundamental gustatory qualities, the status of the amino acid taste has been more uncertain. Some have proposed that the taste evoked by certain amino acids, namely glutamate, especially in combination with nucleotide compounds like inosine monophosphate (IMP), evoke a unique umami sensation,130 whereas others have considered the taste of amino acids to be a complex amalgam of the other qualities. The discovery of receptors for these stimuli has reawakened interest in this debate and led to more widespread acceptance of a “fifth basic quality.”83,164,167 However, it should be noted that even though specific receptors may detect such stimuli, this does not assure their independent status at higher levels of the gustatory system or as a perceptual quality. This issue is discussed later in “Central Gustatory Pathways and Function.”
Although the T1, T2, and metabotropic glutamate receptors cover a broad spectrum of gustatory responsiveness, it is entirely possible that other receptors for bitter, sweet, and amino acid stimuli are yet to be discovered. There are currently no genetic knockouts for any of these receptors, which could assess the degree of their contribution to gustatory perception. In fact, other mechanisms have been proposed to contribute to bitter transduction. For example, quinine can directly block K+ channels in taste receptor cells.168 In addition, discrepancies between recent molecular findings and the much larger body of physiologic studies make it obvious that some of the seductively simple hypotheses about peripheral taste processing are probably not adequate. Questions about bitter processing were mentioned earlier. In addition, in situ hybridization studies demonstrate that T1 and T2 receptors are not colocalized within individual receptor cells (Fig. 89-7). These molecular findings suggest that entirely separate “labeled-lines” code for sweet, bitter, and amino acid stimuli at the most peripheral level of the taste system.163 In contrast, physiologic studies of taste receptor cells using intracellular (reviewed by Herness169) and patch-clamp recording170 or Ca2+ imaging162 all suggest that, although individual taste bud cells are not equivalently responsive across taste qualities, they do exhibit at least some degree of broad tuning; in other words, they respond to more than a single quality (Fig. 89-8). The reason for the inconsistencies between the molecular biologic and the physiologic investigations is unclear, but the two types of studies give different pictures of taste quality coding by the most peripheral elements of the taste system.
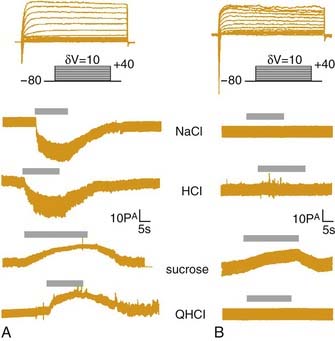
Figure 89-7. Patch-clamp recordings from two taste cells stimulated with four qualitatively different stimuli. The cell on the right (B) responds to just a single stimulus, but the cell on the left (A) responds to all four stimuli. The molecular data such as those presented in Figure 89-8, would not predict the coexistence of responses to sucrose (sweet) and QHCl (bitter) within a single cell.
(From Gilbertson TA, Boughter JD Jr, Zhang H, Smith DV. Distribution of gustatory sensitivities in rat taste cells: whole-cell responses to apical chemical stimulation. J Neurosci. 2001;21:4931-4941.)
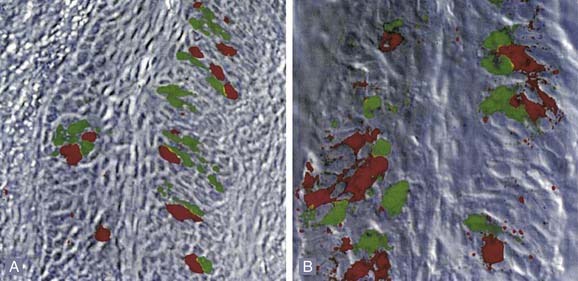
Figure 89-8. Photomicrographs of in situ labeling of taste receptor cells in the circumvallate (A) and foliate (B) papillae. The red labeling denotes staining with probes for the T2R class of receptors which transduce bitter stimuli; green with the T1R class that transduces sweet or amino acid stimuli. Note the lack of overlap of these classes of receptors within individual cells. These data are in some conflict with physiologic data, such as that shown in Figure 89-7.
(From Nelson G, Hoon MA, Chandrashekar J, et al. Mammalian sweet taste receptors. Cell. 2001;106:381-390.)
Part of what complicates the interpretation of data on taste bud cells is how little is understood about processing within the bud itself. Aside from the obvious requirement that these cells must exhibit some degree of differential responsiveness across qualities, they also differ in several other characteristics. Importantly, only a fraction of the receptor cells have classic synapses onto the primary afferent nerve (reviewed by Witt and colleagues134). Because cells in the taste bud, like other epithelial cells, are in a constant state of turnover, it might be thought that the small number of cells with synapses merely reflect a small number of mature cells in the bud (i.e., cells without synapses are in the process of differentiating and thus can neither respond to taste stimuli nor transmit information centrally). However, this simple explanation appears not to be true. None of the cells that express gustducin, a known element in bitter transduction, have classic synapses.171 Thus the mechanism by which information from gustducin-containing, bitter-responsive cells reaches primary afferent neurons is mysterious. Perhaps these receptor cells communicate directly with primary afferent neurons using nonclassical synapses or first send information to other cells in the taste bud, which in turn, synapse with the primary afferent neuron. The possibility of intra-bud communication is bolstered by recent observations that taste bud cells contain and respond to a variety of neurotransmitters and peptides.172,173 A recent molecular study174 suggests that after receptor binding, downstream signaling events for bitter, sweet, and amino acid transduction all require the opening of a specific type of ion channel, the TRP channel. Animals in which the TRPm5 gene has been deleted show no evidence of responses to sweet, bitter, or amino acid stimuli, based either on recordings from the primary afferent nerves or behavioral responses to these stimuli.174 These results are surprising because many other studies suggest that the downstream signaling events are much more heterogeneous (reviewed by Gilbertson and Margolskee8) and provide only limited evidence of TRP-mediated currents in response to taste stimuli in taste receptor cells.175 A true appreciation of the logic of taste coding at the level of the receptor cell will clearly require a better understanding of the reasons for the discrepancies between the physiologic and molecular work and the nature of integrative processing in the taste bud–primary afferent nerve circuit.
Peripheral Sensitivity
REGIONAL DIFFERENCES
Regardless of the controversy surrounding the specificity of responsiveness of individual receptor cells in experimental animals, it is notable that single human fungiform papillae are broadly tuned across taste qualities. In humans, single fungiform papillae have an average of only about four taste buds,139,176 and thus although there is certainly more than a single receptor cell in a given papillae, at the same time there is quite a limited number. Initial observations that single fungiform papillae were sensitive to a single taste quality resulted from stimulus concentrations that were too low.177 In taste, as in other sensory systems, there is a trade-off between the area stimulated and the threshold concentration. The lingual threshold for a given gustatory stimulus requires progressively higher concentrations for progressively smaller areas. In one study, when single papillae were stimulated with sufficiently high concentrations, the majority of fungiform papillae mediated multiple taste sensations. Sixty-six percent of the fungiform papillae tested elicited recognition of at least three of the four standard taste qualities.177 Similar results have been obtained by other investigators.178,179
Gustatory receptors sample food or fluid as it is ingested, masticated, and transported to the back of the mouth for swallowing. Receptor densities appear greatest at critical junctures of the ingestive sequence, which are outlined in Figure 89-6. Gustatory receptors at the tip of the tongue are contacted immediately as food enters the mouth and are optimally situated to determine whether to continue or abort the ingestive sequence. A second population on the back and sides of the tongue and on the opposing palate is probably stimulated during mastication when food is crushed between the molars. In addition to differences in location, the varied sensitivity of the various subpopulations of gustatory receptors to chemical stimuli suggests a differential contribution to gustatory function. However, it needs to be kept in mind that sensitivity differences are relative and not always related in a simple manner to variations in function.
The chorda tympani nerve in many animal species is highly sensitive to a variety of salts (e.g., NaCl). This sensitivity is consistent with human psychophysical studies that show a low threshold to NaCl on the anterior aspect of the tongue.180 Studies in rats indicate that many individual chorda tympani fibers are sensitive to both NaCl and hydrochloric acid (which tastes sour to humans) but that a subset of peripheral nerve fibers are responsive exclusively to sodium salts.148 When the sodium channel blocker amiloride was applied to the surface of the tongue, only the sodium-specific fibers lost their responsiveness to NaCl. Those chorda tympani fibers sensitive to both salts and hydrochloric acid maintained their sensitivity to NaCl stimuli in the presence of amiloride, implying that the sodium-specific neurons are particularly important for coding the salty quality of NaCl. Moreover, the recognition of NaCl decreases after chorda tympani nerve section in rats, further indicating a specialized role for this nerve in sodium recognition.181
Many mammals including humans have a high sensitivity to sugars and other sweet-tasting compounds on the anterior tongue. A common subject in gustatory research, the rat seems to be an exception because of its poor anterior tongue sensitivity to sweet stimuli. However, even in this species, a robust responsiveness to sweet stimuli is apparent in the anterior oral cavity, specifically in the nasoincisor ducts on the hard palate that lie in apposition to the anterior tongue.182 The greater superficial petrosal branch of the facial nerve innervates the nasoincisor duct and soft palate receptors. In fact, the soft palate is also highly responsive to sweet stimuli.183,184 Another rodent, the hamster, also exhibits a relatively greater responsiveness to sweet stimuli applied to the palate vs the tongue, but the hamster tongue also responds well to sweet stimuli.185 Whether other mammals including humans are relatively more responsive to sweet stimuli applied to the palate is an open question. However, in humans, taste buds have only been described on the soft186 but not the hard palate. Across a variety of species (e.g., rat,187 sheep,188 gerbil,189 mouse,190 monkey191), gustatory receptors on the posterior tongue are relatively more responsive to aversive, particularly bitter, stimuli than are receptors in the anterior oral cavity. Stimulation of taste receptors with bitter tastants initiates powerful rejection responses.192–194 Consistent with this differential sensitivity, sectioning the glossopharyngeal nerves in rats attenuates the rejection response to quinine monohydrochloride to a greater degree than does sectioning the chorda tympani nerves.195,196 However, surprisingly, section of the chorda tympani nerve actually has a greater effect on quinine discrimination.197 These consequences of lesions and other considerations have led to the suggestion that the anterior oral cavity is more important in gustatory discrimination per se (regardless of quality), whereas the posterior oral cavity is more important in providing input for gustatory-guided reflexes.187,197
A specific function is particularly apparent for the superior laryngeal nerve. Chemoresponsive fibers in the superior laryngeal branch of the vagus nerve differ greatly from facial and glossopharyngeal nerve sensitivities. In general, superior laryngeal nerve fibers are insensitive to NaCl and sucrose but respond well to stimulation with potassium chloride, ammonium chloride, acids, and interestingly, water.198,199 In fact, individual nerve fibers in the superior laryngeal nerve are not divisible into groups on the basis of their optimal sensitivity, as are fibers in the chorda tympani and glossopharyngeal nerves, but rather compose a single broadly responsive group.200 The location of superior laryngeal nerve-innervated taste buds in the larynx and on the epiglottis indicates a protective-reflex role for these receptors, rather than contributing to gustatory quality perception. The superior laryngeal nerve is a particularly low-threshold nerve for eliciting swallows that could protect the airway.201
In addition to these neurophysiologic studies in animals, human psychophysical studies show clear regional variation in the recognition threshold to different gustatory stimuli.180 The front of the tongue had the lowest threshold for both salty and sweet stimuli; sour stimuli had the lowest threshold when applied to the foliate papillae. Although the front of the tongue also had the lowest threshold for bitter stimuli such as quinine, stimulation of the circumvallate papillae produced a steeper intensity function than obtained by stimulating the front of the tongue. The psychophysical scaling results for quinine monohydrochloride are consistent with the frequent observation that bitter sensations are more intense in the back of the mouth. With the exception of the high sensitivity to sour stimuli on the sides of the tongue, the gradient for threshold to chemical stimuli on the tongue follows the gradient for thresholds to mechanical stimuli, with the anterior region the most sensitive.
Despite these regional variations in threshold and concentration response functions, sensations of sweet, sour, salty, and bitter can be elicited from each oral region where taste buds are present. Moreover, the loss of a single gustatory nerve may not be apparent to the individual and can often be ascertained only by specific psychophysical procedures.202 In general, the high degree of specialization among the different gustatory nerves of experimental animals is not as obvious in humans. Destruction of the chorda tympani from middle ear surgery destroys taste sensitivity from the front of the tongue,203,204 but there have been no reports of a disruption of salt intake. However, following third molar surgery, which has the potential to damage the chorda tympani nerve, patients incorrectly identified the taste of NaCl slightly more frequently after surgery.205 It is also interesting to note that humans with laryngectomies reported thirst less often and were less able to localize thirst compared with a control group, suggestive of a role for the superior laryngeal nerve.206 Although deficits after damage of single taste nerves are neither profound nor impressively specific, these data need to be interpreted cautiously due to their limited nature. Thus only a few studies of taste responsivity after chorda tympani damage have been carried out; no studies of the gustatory consequences of glossopharyngeal damage have been reported. In addition, perceptual testing has been limited and may not have detected subtle but significant effects on gustatory discrimination. Nevertheless, the regional intraoral variation of taste sensitivity of humans may represent only a vestigial form of reflex organization, superseded by a wider distribution of gustatory sensitivities within the oral cavity and by an increase in the voluntary neural control of ingestion.
INDIVIDUAL DIFFERENCES
Although there are regional differences in taste sensitivity within an individual, an equally salient feature of human gustatory perception is wide variation from person to person. Several studies have demonstrated as much as a 100-fold variation in detection threshold for both sucrose and NaCl across a wide age span.207 The longest studied and perhaps clearest example of individual differences is the variation in both threshold and responsiveness to the chemically related compounds propothiouracil (PROP) and phenylthiocarbamide (PTC). Decades before anything was known of the molecular basis of bitter transduction, a fortuitous discovery by a chemist synthesizing PTC revealed that some persons found relatively low concentrations of this substance intensely bitter, whereas others found it nearly tasteless.208 Genetic studies have found this variability to be a heritable trait, but there is still disagreement on the mode of inheritance.209 Although thresholds for PTC and PROP show a bimodal distribution, there is a continuum of sensitivity in the middle range, suggesting that PTC/PROP sensitivity is not transmitted as a simple Mendelian dominant trait. Rather, partial dominance or multilocus models appear more appropriate.209 In a recent study, Reed and colleagues160,209 found a strong linkage to chromosome 5 and a suggestion of a linkage on chromosome 7 near sites where the molecular studies have identified G-protein-coupled receptors for bitter stimuli. Thus it might be tempting to speculate that PROP sensitivity is a consequence of phenotypic variation in the inheritance of partially dominant bitter receptors strongly responsive to PROP and related compounds. However, in some studies, persons who exhibit high or low responsiveness to PROP show similar differences to other stimuli including other bitter-210,211 and sweet-tasting211 compounds, as well as differences in responsiveness to a nongustatory stimulus, capsaicin, which produces a burning sensation.211 Thus it seems possible that some of the variability in PROP sensitivity reflects a more general variation in oral sensory sensitivity.
Indeed, differences in the number of fungiform papillae and pores have been identified in groups of people with high, moderate, and low sensitivity to PROP. “Supertasters” have the most fungiform papillae and highest sensitivity, “nontasters” have the fewest fungiform papillae and lowest sensitivity, and “medium-tasters” are in between on both measures.212 Other studies have demonstrated a similar relationship between papillae number and sensitivity to gustatory stimuli including sucrose, NaCl, and PROP.176 However, the relationship between fungiform papillae number and taste sensitivity, whether to PROP or other compounds, exhibits a high degree of variability, such that individuals assigned to various groups often overlap in their anatomic characteristics.176,210,212 Thus the factors underlying individual differences in taste sensitivity are undoubtedly multifactorial and include not only multiple genetic factors (some yet to be discovered), but environmental factors such as nerve damage caused by otitis media,213 as well as a decline in taste sensitivity with age.214,215
Whatever the underlying basis, it is interesting that a number of studies have made the suggestion that variation in taste sensitivity has the potential to affect health. Thus although there is not universal agreement, several studies have reported that people with a high PROP sensitivity claim less preference for certain vegetables (reviewed by Drewnowski7), and this appears to affect their intake.216 Preliminary evidence suggests that these preferences could affect gastrointestinal health. A recent abstract217 reported a positive relationship between colon polyps and PROP taster status. A relationship between oral health and PROP status has also been claimed, with individuals sensitive to PROP having fewer dental caries.218–220 It has been speculated that this is due to a greater sensitivity to sweet, as well as bitter, substances, so highly sensitive individuals can consume lower amounts of sugar to achieve the same amount of satisfaction. However, given the lack of unanimous agreement on the positive relationship between PROP and general gustatory sensitivity, as well as the lack of any direct evidence regarding dietary habits in these individuals, more work is necessary to validate these claims and to understand their basis.
Central Gustatory Pathways and Function
Afferent gustatory fibers in the facial, glossopharyngeal, and vagus nerves synapse in the nucleus of the solitary tract (NST) of the medulla with a rostral to caudal organization.221,222 In primates, taste information projects directly to the gustatory thalamus, which is situated medial to the oral somatosensory representation in the ventroposteromedial nucleus.223 From the thalamus, taste information projects to the insular-opercular region of the cortex,224 which comprises the primary gustatory cortex and then to a secondary gustatory cortical area located immediately anterior in the caudolateral orbitofrontal cortex.225 The secondary gustatory cortex projects to several regions of the ventral forebrain including the hypothalamus and amygdala.10 Positron emission tomography and functional MRI studies in humans have demonstrated that cortical regions analogous to those demonstrated on the basis of anatomic and physiologic studies in nonhuman primates most likely also comprise the primary and secondary gustatory cortical areas in people (Fig. 89-9).226
The gustatory pathway in rodents is organized somewhat differently. Taste information from the NST reaches an analogous thalamic relay in the ventro-posteromedial nucleus, but only after an additional synapse in the parabrachial nucleus of the pons.227,228 The region identified as the primary gustatory cortex in rodents is also the insular cortex,229,230 but a secondary taste cortex has not yet been identified. Instead, in rodents, taste information reaches limbic areas more directly, via a projection from the parabrachial nucleus.231 However, regardless of species and the route the information takes, it has been hypothesized that the thalamocortical pathway can be specialized for perceptual/discriminative gustatory functions; the limbic projections may be more involved in the hedonic/motivational attributes of taste.232 Local brainstem gustatory pathways, however, have the capacity to mediate basic gustatory discriminative functions. Decerebrate animals233 and anencephalic human infants193 discriminate palatable from unpalatable gustatory stimuli.
Gustatory pathways are in close anatomic proximity with central pathways controlling the autonomic nervous system function. This proximity provides a substrate for interactions between gustatory and autonomic afferent information,234 as do the numerous connections between limbic structures and the nuclei in the taste pathway.140 In rodents, an early study demonstrated changes in the firing pattern of gustatory-responsive neurons in the nucleus of the solitary tract in response to gut distension that were indicative of interaction between the autonomic and gustatory systems.235 More recent studies have extended these findings to the parabrachial nucleus. Indeed, not only does gut distention affect parabrachial taste neurons,236 but another manipulation that mimics satiety, intraduodenal lipid infusion, also affects gustatory firing rates.237 Specifically, lipid infusions depress taste responses, and furthermore this occurs mainly for responses to normally preferred gustatory stimuli. Thus lipid infusions most profoundly depress responses in neurons preferentially responsive to NaCl, and especially those responsive to sucrose. This is in contrast to a lack of effect on responses evoked by HCl and QHCl, stimuli that animals do not normally voluntarily ingest.237 These effects of gastric and duodenal stimuli suggest a simple mechanism for the reduction in intake that occurs with the visceral sequelae of intake—namely, that neurons responsive to preferred taste stimuli, which normally drive ingestion, reduce their input into feeding circuits when the animal is satiated. Other changes in body state that affect ingestion have also been shown to have effects on early stages of taste processing in rodents. Both sodium deprivation and conditioned taste aversion have stimulus-specific effects in the NST.238–240 In fact, different levels of dietary sodium even affect responses at the level of the peripheral nerve.241,242 However, the nature of these neural changes induced by sodium appetite or conditioned taste aversion can be rather complex, and taken together they are not correlated in a straightforward way with the behavioral changes these state changes produce.240 It is also of interest that these modulations observed at the early stages of gustatory processing in rodents appear only to occur at higher stages of processing in primates, although this has been evaluated only after inducing a state of satiety. In monkeys, recordings from the NST from awake, behaving animals showed that gustatory responses did not change as the animal was fed to a state of satiety.243 In fact, taste responses were likewise stable in the primary insular-opercular cortex, regardless of whether the animal was hungry or sated.244 However, in secondary, orbitofrontal gustatory cortex,245 as well as in the hypothalamus246 and amygdala,247 taste responses become progressively reduced during the course of feeding. A particularly interesting aspect of the primate phenomena is that the effect on taste responses exhibits what is known as “sensory-specific” satiety. That is, even within the realm of preferred stimuli, taste responses are most reduced for the stimulus that actually produces the satiety. For example, neurons responsive to both glucose and a complex stimulus, black currant juice, mainly exhibited reduced glucose responses if glucose was used to feed the monkey until he refused this stimulus. Neural and behavioral responsiveness to black currant juice, however, were relatively unaltered.10
Analogous to these effects demonstrated in experimental animals, changes in body state also affect perception of taste stimuli in humans. Such a phenomenon is readily demonstrated in short-term experiments, when subjects rate the pleasantness of a sweet taste before and after the induction of satiety. A classic study by Cabanac and colleagues248 showed that ratings of the pleasantness of sucrose declined over time when subjects ingested the samples, drank an equivalent quantity of sucrose as a bolus, or received intragastric sucrose. Interestingly, ratings were unaffected by simple gastric distention, infusion of hypertonic saline, or intravenous glucose infusion. Later studies found similar effects.9 Further, similar to the primate experiments just discussed, these changes in pleasantness exhibit sensory-specific satiety249 and, accordingly, people will eat larger meals when the meals are composed of more varied foods.250
It has been more difficult to document preference of differences among populations of persons with different chronic conditions. For example, preferences for sweet stimuli in obese versus nonobese persons have been reported to be enhanced in some studies, but in other studies have been reported to be depressed or the same.9 It seems likely that these equivocal results reflect the heterogeneity of the obese population in terms of etiology, chronicity, and dietary status, as well as the complexities involved in obtaining accurate preference ratings, particularly in a population of people who may feel self-conscious about their eating habits. Studies of diabetic patients are more consistent in reporting elevated psychophysical thresholds to glucose.251,252 Loss of gustatory sensitivity in diabetics may result from a systemic lack of glucose receptors and from general neuropathy. However, even diabetes can be associated with multiple effects. An animal model of this disease, performed with the db/db mouse, shows elevated peripheral nerve responses to sugars.253 Thus conditions that affect carbohydrate metabolism and body weight obviously have the potential to influence taste responsiveness, but the relationship is complicated and requires further study.
With regard to umami taste, evidence from cortex is in some disagreement. An initial study254 suggested a unique status for this quality. Recordings from primary and secondary gustatory cortex demonstrated that the prototypic umami stimulus, monosodium glutamate (MSG), was just as effective as four standard gustatory stimuli—glucose (sweet), NaCl (salty), HCl (sour), and QHCl (bitter)—measured either by average firing rates or with regard to the number of neurons that responded optimally to a given stimulus. Furthermore, the neural representation of MSG relative to the other stimuli was just as distinct as the above four stimuli were from each other on the basis of correlations between responses to the stimuli evoked across the populations of neurons. Although the stimulus array used in that study was limited and did not allow a ready comparison between stimuli that are known to be the same and those known to be different, in a follow-up study with the four standard taste stimuli plus two umami stimuli,255 it was indeed shown that the representation of MSG and the other umami stimulus, IMP, was more similar than any other stimulus pair. However, these data are not entirely compelling because the correlation between MSG and IMP (0.77) was actually only nominally greater than the correlation between MSG and the sour stimulus, HCl (0.71). Furthermore, in another study that used a large array of amino acids, MSG was not shown to be distinctly represented relative to the four standard qualities but was instead highly correlated with NaCl,256 an observation that has been made frequently at lower levels of the rodent gustatory system.257 Thus although amino acid stimuli activate a special set of gustatory receptors and are effective for stimulating taste neurons throughout the neuraxis, whether or not they represent a unique taste or a complex conglomerate of the classic four is still unresolved.
The neural representation of dietary fats has long been a mystery. Although the presumption had been that fat stimuli were represented not on the basis of their ability to stimulate the gustatory system but rather due to their olfactory and somatosensory properties, interest in a possible gustatory efficacy was reawakened by the discovery that certain free fatty acids were capable of stimulating taste receptor cells.258 This neural representation of fat has recently been studied in the monkey orbitofrontal cortex.259,260 This is a particularly appropriate brain region in which to study these stimuli because it receives not only gustatory but also olfactory and somatosensory inputs. Fatty stimuli (e.g., cream) were shown to activate a small subset of neurons in the orbitofrontal cortex. Occasionally, neurons were observed that responded to fat stimuli but not to standard taste stimuli, but more frequently fat-responsive cells were also taste responsive. There was not an obvious relationship between the gustatory sensitivity spectrum of a neuron and whether it responded to fat stimuli. Significantly, the chemical properties of the fats were not important in their effectiveness because nonfat stimuli including silicone and mineral oil were just as effective as dietary fats such as cream and vegetable oil. Thus at least in this sample of neurons, neither the olfactory nor the gustatory properties of the fats appeared to be responsible for driving the cells. Furthermore, the free-fatty acids specifically shown to stimulate taste receptor cells, linoleic and lauric, were poor stimuli for these cells, being no more effective than water. Indeed, this was true even though coconut oil, which contains high amounts of these fatty acids, was a highly effective stimulus. Thus it was concluded that the somatosensory properties of the fats were the determining factor. Interestingly, a parametric investigation of the responses of these cells to viscosity revealed that the responses of only a subset of these cells to fat could be predicted by their responses to viscosity. Thus a different, unidentified somatosensory property must be a critical variable in determining the effectiveness of fats as a stimulus.
Interaction between Saliva and Taste
The presence of saliva in the mouth continually stimulates gustatory receptors with low levels of salt ions. Correspondingly, recognition thresholds for NaCl are somewhat raised when the tongue is adapted with a solution containing salivary levels of sodium (4.3 mm compared with recognition thresholds using distilled water rinses, 0.14 mm).261 By implication, the presence of other salivary constituents as a result of either disease or medication may affect gustatory sensitivity.262 Salivary concentrations of pirmenol in patients being treated for ventricular arrhythmias, for example, can produce the bitter taste reported by these patients.263 Increased salivary levels of glucose in diabetic patients provide one mechanism for the increased detection thresholds for glucose in this patient population.252
Saliva can also exert a trophic influence on gustatory receptors. Patients suffering long-term salivary loss as a result of Sjögren’s syndrome had increased detection and recognition threshold to many gustatory stimuli.264 Biopsy specimens of the circumvallate papillae from a subset of these patients indicated a profound loss of taste buds. The effects of desalivation on both taste bud morphology and gustatory sensitivity have also been explored in animals.265,266 Surgically removing the salivary glands was associated with increased keratosis of the lingual epithelium and shrinking of the circumvallate papillae. Correlated with these morphologic changes was the increased consumption of nonpreferred gustatory stimuli, indicative of a loss of gustatory sensitivity. Electron microscopic observation of the circumvallate papillae showed the infiltration of bacteria, suggesting that the loss of antibacterial agents in saliva permitted degenerative microbial action. Lack of salivation by acute pharmacologic manipulations in experimental human studies, however, had relatively little effect on gustatory sensitivity.267
The loss of taste acuity in humans after radiotherapy to the head and neck could result directly from the destruction of taste buds and indirectly from reduced salivary flow.268 Direct irradiation of gustatory structures in experimental animals produced a loss of taste buds.269 Radiotherapy can also influence the gustatory system through the formation of conditioned taste aversions.270,271 Clinical observations of hedonic changes in taste may result, in part, from the pairing of a conditioned stimulus (food) with an unconditioned stimulus, the gastrointestinal distress resulting from either chemotherapy or abdominal radiation. Animal experiments indicate that such pairings can have a profound impact on gustatory preferences.272 Animal studies also indicate that the formation of a conditioned taste aversion is a central phenomenon that requires an intact forebrain.
Boughter J, Bachmanov A. Behavioral genetics and taste. BMC Neuroscience. 2007;8(September (Suppl 3)):S3.
Breslin P, Spector A. Mammalian taste perception. Curr Biol. 2008;18(February (4)):R148-155.
Chandreshekar J, Hoon M, Ryba NJ, et al. The receptors and cells for mammalian taste. Nature. 2006;444(November 711):288-294.
Lemon C, Katz D. The neural processing of taste. BMC Neuroscience. 2007;8(September(Suppl 3)):S5.
Lindemann B, Ogiwara Y, Ninomiya Y. The discovery of umami. Chem Senses. 2002;27:843-844.
Lund JP, Kolta A. Brainstem circuits that control mastication: do they have anything to say during speech? J Commun Disord. 2006;39(September-October (5)):381-390.
Lund JP, Kolta A. Generation of the central masticatory pattern and its modification by sensory feedback. Dysphagia. 2006;3(July):167-174.
Matsuo K, Palmer J. Anatomy and physiology of feeding and swallowing: normal and abnormal. Phys Med Rehabil Clin N Am. 2008;19(November (4)):691-707.
Miles TS. Postural control of the human mandible. Arch Oral Biol. 2007;52(April (4)):347-352.
Mistry S, Hamdy S. Neural control of feeding and swallowing. Phys Med Rehabil Clin N Am. 2008;19(November (4)):709-728.
Sessle BJ. Mechanisms of oral somatosensory and motor functions and their clinical correlates. Jrl Oral Rehab. 2006;33(April):243-261.
Svensson P, Jadidi F, Arima T, et al. Relationships between craniofacial pain and bruxism. Jrl Oral Rehab. 2008;35(June):524-547.
Trulsson M. Sensory-motor function of human periodontal mechanoreceptors. Jrl Oral Rehab. 2006;33(April):262-273.
Turker K. Reflex control of human jaw muscles. Crit Rev Oral Biol Med. 2002;13(January (1)):85-104.
Turker K, Sowman PF, Tuncer M, et al. The role of peridontal mechanoreceptors in mastication. Arch Oral Biol. 2007;52(April (4)):361-364.
van der Bilt A, Engelen L, Pereira LJ, et al. Oral physiology and mastication. Phys Behav. 2006;89(August (1)):22-27.
van der Glas H, van der Bilt A, Abbink JH, et al. Functional roles of oral reflexes in chewing and biting: phase-, task-, and site-dependent reflex sensitivity. Arch Oral Biol. 2007;52(April (4)):365-369.
Zhang F, Klebansky B, Fine RM, et al. Molecular mechanism for the umami taste synergism. Proc Natl Acad Sci U S A. 2008;105(December (52)):20930-20934.
1. Darian-Smith I. The trigeminal system. In: Iggo A, editor. Handbook of Physiology. New York: Springer-Verlag, 1973.
2. Jacobs R, Bou Serhal C, van Steenberghe D. Oral stereognosis: a review of the literature. Clin Oral Investig. 1998;2:3-10.
3. Jean A. Brain stem control of swallowing: neuronal network and cellular mechanisms. Physiol Rev. 2001;81:929-969.
4. Miller AJ. The neuroscientific principles of swallowing and dysphagia. San Diego, California: Singular; 1999.
5. Byers MR. Dental sensory receptors. Int Rev Neurobiol. 1984;25:39-94.
6. Capra NF. Mechanisms of oral sensation. Dysphagia. 1995;10:235-247.
7. Drewnowski A. Genetics of human taste perception. Chapter 40. Doty RL, editor. Handbook of Olfaction and Gustation. New York: Marcel Dekker, 2003.
8. Gilbertson TA, Margolskee RF. Molecular physiology of gustatory transduction. Chapter 34. Doty RL, editor. Handbook of Olfaction and Gustation. New York: Marcel Dekker, 2003.
9. Mattes RD. Nutritional implications of taste and smell. Chapter 42. Doty RL, editor. Handbook of Olfaction and Gustation. New York: Marcel Dekker, 2003.
10. Rolls ET, Scott TR. Central taste anatomy and physiology. Chapter 33. Doty RL, editor. Handbook of Olfaction and Gustation. New York: Marcel Dekker, 2003.
11. Sessle BJ. Acute and chronic craniofacial pain: brainstem mechanisms of nociceptive transmission and neuroplasticity, and their clinical correlates. Crit Rev Oral Biol Med. 2000;11:57-91.
12. Sharav Y. Orofacial pain. In: Wall PD, Melzack R, editors. Textbook of Pain. London: Churchill Livingstone, 1999.
13. Bromley SM, Doty RL. Clinical disorders affecting taste: evaluation and management. In: Doty RL, editor. Handbook of Olfaction and Gustation. New York: Marcel Dekker, 2003.
14. Rath EM, Essick GK. Perioral somesthetic sensibility: do the skin of the lower face and the midface exhibit comparable sensitivity? J Oral Maxillofac Surg. 1990;48:1181-1190.
15. Manly RS, Pfaffman C, Lathrop DD, et al. Oral sensory thresholds of persons with natural and artificial dentitions. J Dent Res. 1952;31:305-312.
16. Green BG, Gelhard B. Perception of temperature on oral and facial skin. Somatosens Res. 1987;4:191-200.
17. Yamada M, Maruhashi J, Miyake N. The distribution of sensory spots on the oral mucous membrane. Jpn J Physiol. 1952;2:73-81.
18. Trulsson M, Essick GK. Low-threshold mechanoreceptive afferents in the human lingual nerve. J Neurophysiol. 1997;77:737-748.
19. Marlow CD. General sensory innervation of the human tongue. Anat Rec. 1965;152:503-512.
20. Gairns FW. The sensory nerve endings of the human palate. Q J Exp Physiol. 1955;40:40-48.
21. Dixon AD. Sensory nerve terminals in the oral mucosa. Arch Oral Biol. 1961;5:105-114.
22. Munger BL. The general somatic afferent terminals in oral mucosa. In: Simon SA, Roper SD, editors. Mechanisms of Taste Transduction. Boca Raton, Fla: CRC Press, 1993.
23. Tachibana T, Fujiwara N, Sato H, et al. A comparative electron microscopic analysis of mechanoreceptors in the hard palate of the mouse (Mus musculus; Rodentia) and the musk shrew (Suncus murinus; Insectivora). Arch Oral Biol. 1990;35:949-956.
24. Jacobs R, van Steenberghe D. Role of periodontal ligament receptors in the tactile function of teeth: a review. J Periodontal Res. 1994;29:153-167.
25. Maeda T, Ochi K, Nakakura-Oshima K, et al. The Ruffini ending as the primary mechanoreceptor in the periodontal ligament: its morphology, cytochemical features, regeneration, and development. Crit Rev Oral Biol Med. 1999;10:307-327.
26. Byers MR. Sensory innervation of periodontal ligament of rat molars consists of unencapsulated Ruffini-like mechanoreceptors and free nerve endings. J Comp Neurol. 1985;231:500-518.
27. Byers MR, Dong WK. Comparison of trigeminal receptor location and structure in the periodontal ligament of different types of teeth from the rat, cat, and monkey. J Comp Neurol. 1989;279:117-127.
28. Jerge CR. Organization and function of the trigeminal mesencephalic nucleus. J Neurophysiol. 1963;26:379-392.
29. Byers MR, O’Connor TA, Martin RF, et al. Mesencephalic trigeminal sensory neurons of cat: axon pathways and structure of mechanoreceptive endings in periodontal ligament. J Comp Neurol. 1986;250:181-191.
30. Cash RM, Linden RW. The distribution of mechanoreceptors in the periodontal ligament of the mandibular canine tooth of the cat. J Physiol. 1982;330:439-447.
31. Trulsson M, Johansson RS. Orofacial mechanoreceptors in humans: encoding characteristics and responses during natural orofacial behaviors. Behav Brain Res. 2002;135:27-33.
32. Trulsson M. Multiple-tooth receptive fields of single human periodontal mechanoreceptive afferents. J Neurophysiol. 1993;69:474-481.
33. Kidokoro Y, Kubota K, Shuto S, et al. Possible interneurons responsible for reflex inhibition of motoneurons of jaw-closing muscles from the inferior dental nerve. J Neurophysiol. 1968;31:709-716.
34. van Steenberghe D, van den Bergh A, de Vries JH, et al. The influence of advanced periodontitis on the psychophysical threshold level of periodontal mechanoreceptors in man. J Periodontal Res. 1981;16:199-204.
35. Trulsson M, Gunne HS. Food-holding and -biting behavior in human subjects lacking periodontal receptors. J Dent Res. 1998;77:574-582.
36. Williams WN, Levin AC, LaPointe LL, et al. Bite force discrimination by individuals with complete dentures. J Prosthet Dent. 1985;54:146-150.
37. Bryant BP, Silver WL. Chemesthesis: the common chemical sense. In: Finger TE, Silver WL, Restrepo D, editors. The Neurobiology of Taste and Smell. New York: Wiley-Liss, 2000.
38. Caterina MJ, Julius D. The vanilloid receptor: a molecular gateway to the pain pathway. Annu Rev Neurosci. 2001;24:487-517.
39. Ahlquist M. Quality of pain sensations following application of algogenic agents on the exposed human tooth pulp: a psychophysical and neurophysiological study. In: Fields HL, Dubner R, Cervero F, editors. Advances in Pain Research and Therapy. New York: Raven Press, 1985.
40. Burgess J, Byers MR, Dworkin SF. Pain of dental and intraoral origin. In: Bonica JJ, editor. The Management of Pain. Philadelphia: Lea and Febiger, 1990.
41. Hargreaves KM, Swift JQ, Roszkowski MT, et al. Pharmacology of peripheral neuropeptide and inflammatory mediator release. Oral Surg Oral Med Oral Pathol. 1994;78:503-510.
42. Bowles WR, Withrow JC, Lepinski AM, et al. Tissue levels of immunoreactive substance P are increased in patients with irreversible pulpitis. J Endod. 2003;29:265-267.
43. Byers MR, Taylor PE. Effect of sensory denervation on the response of rat molar pulp to exposure injury. J Dent Res. 1993;72:613-618.
44. Byers MR, Dong WK. Autoradiographic location of sensory nerve endings in dentin of monkey teeth. Anat Rec. 1983;205:441-454.
45. Narhi M, Jyväsjärvi E, Virtanen A, et al. Role of intradental A- and C-type nerve fibres in dental pain mechanisms. Proc Finn Dent Soc. 1992;88:S507-S516.
46. Ahlquist M, Franzen O, Coffey J, et al. Dental pain evoked by hydrostatic pressures applied to exposed dentin in man: a test of the hydrodynamic theory of dentin sensitivity. J Endod. 1994;20:130-134.
47. Kelly JP. The trigeminal system. In: Kandell ER, Schwartz JH, editors. Principles of Neural Science. New York: Elsevier Science Publishing, 1985.
48. Sessle BJ, Hu JW, Amano N, et al. Convergence of cutaneous, tooth pulp, visceral, neck and muscle afferents onto nociceptive and non-nociceptive neurones in trigeminal subnucleus caudalis (medullary dorsal horn) and its implications for referred pain. Pain. 1986;27:219-235.
49. Capra NF. Localization and central projections of primary afferent neurons that innervate the temporomandibular joint in cats. Somatosens Res. 1987;4:201-213.
50. Shigenaga Y, Chen IC, Suemune S, et al. Oral and facial representation within the medullary and upper cervical dorsal horns in the cat. J Comp Neurol. 1986;243:388-408.
51. Sharav Y, Leviner E, Tzukert A, et al. The spatial distribution, intensity and unpleasantness of acute dental pain. Pain. 1984;20:363-370.
52. Young RF. Effect of trigeminal tractotomy on dental sensation in humans. J Neurosurg. 1982;56:812-818.
53. Graham SH, Sharp FR, Dillon W. Intraoral sensation in patients with brainstem lesions: role of the rostral spinal trigeminal nuclei in pons. Neurology. 1988;38:1529-1533.
54. Woolf CJ, Doubell TP. The pathophysiology of chronic pain: increased sensitivity to low threshold A beta-fibre inputs. Curr Opin Neurobiol. 1994;4:525-534.
55. Chiang CY, Park SJ, Kwan CL, et al. NMDA receptor mechanisms contribute to neuroplasticity induced in caudalis nociceptive neurons by tooth pulp stimulation. J Neurophysiol. 1998;80:2621-2631.
56. Yu XM, Sessle BJ, Haas DA, et al. Involvement of NMDA receptor mechanisms in jaw electromyographic activity and plasma extravasation induced by inflammatory irritant application to temporo-mandibular joint region of rats. Pain. 1996;68:169-178.
57. Craig ADJr, Burton H. Spinal and medullary lamina I projection to nucleus submedius in medial thalamus: a possible pain center. J Neurophysiol. 1981;45:443-466.
58. Jain N, Qi HX, Catania KC, et al. Anatomic correlates of the face and oral cavity representations in the somatosensory cortical area 3b of monkeys. J Comp Neurol. 2001;429:455-468.
59. DaSilva AF, Becerra L, Makris N, et al. Somatotopic activation in the human trigeminal pain pathway. J Neurosci. 2002;22:8183-8192.
60. Pearson KG. Common principles of motor control in vertebrates and invertebrates. Annu Rev Neurosci. 1993;16:265-297.
61. Nakamura Y, Katakura N. Generation of masticatory rhythm in the brainstem. Neurosci Res. 1995;23:1-19.
62. Rekling JC, Feldman JL. PreBötzinger complex and pacemaker neurons: hypothesized site and kernel for respiratory rhythm generation. Annu Rev Physiol. 1998;60:385-405.
63. Lowe AA. The neural regulation of tongue movements. Prog Neurobiol. 1981;15:295-344.
64. Hiiemae KM, Crompton AW. Mastication, food transport, and swallowing. In: Hildebrand M, editor. Functional Vertebrate Morphology. Cambridge: Belknap Press, 1985.
65. Thexton A. Jaw, tongue and hyoid movement—a question of synchrony? [discussion]. J R Soc Med. 1984;77:1010-1019.
66. Koolstra JH. Dynamics of the human masticatory system. Crit Rev Oral Biol Med. 2002;13(4):366-376.
67. Miller AJ. Oral and pharyngeal reflexes in the mammalian nervous system: their diverse range in complexity and the pivotal role of the tongue. Crit Rev Oral Biol Med. 2002;13:409-425.
68. Orchardson R, Cadden SW. Mastication. In: Linden RWA, editor. The Scientific Basis of Eating. Basal: Karger, 1998.
69. Laboissiere R, Ostry DJ, Feldman AG. The control of multi-muscle systems: human jaw and hyoid movements. Biol Cybern. 1996;74:373-384.
70. Miller AJ. Craniomandibular muscles: their role in function and form. Boca Raton, Florida: CRC Press; 1991.
71. van Eijden TM, Turkawski SJ. Morphology and physiology of masticatory muscle motor units. Crit Rev Oral Biol Med. 2001;12:76-91.
72. Stephenson GM. Hybrid skeletal muscle fibres: a rare or common phenomenon? Clin Exp Pharmacol Physiol. 2001;28:692-702.
73. Korfage JA, Brugman P, Van Eijden TM. Intermuscular and intramuscular differences in myosin heavy chain composition of the human masticatory muscles. J Neurol Sci. 2000;178:95-106.
74. Dubner R, Sessle BJ, Storey AT. The Neural Basis of Oral and Facial Function. New York: Plenum Press; 1978.
75. Thexton AJ. Mastication and swallowing: an overview. Br Dent J. 1992;173:197-206.
76. Cadden SW, Newton JP, Yemm R. Electromyographic evidence for a digastric reflex evoked by perioral stimuli in humans. J Oral Rehabil. 1997;24:439-443.
77. Travers JB. Oromotor nuclei. In: Paxinos G, editor. The Rat Nervous System. San Diego, California: Academic Press, 2004.
78. Cunningham DP, Basmajian JV. Electromyography of genioglossus and geniohyoid muscles during deglutition. Anat Rec. 1969;165:401-409.
79. Travers JB, Jackson LM. Hypoglossal neural activity during licking and swallowing in the awake rat. J Neurophysiol. 1992;67:1171-1184.
80. Kier WM, Smith KK. Tongues, tentacles and trunks: the biomechanics of movement in muscular-hydrostats. Zool J Linnean Soc. 1985;83:307-396.
81. Korfage JA, Schueler YT, Brugman P, et al. Differences in myosin heavy-chain composition between human jaw-closing muscles and supra- and infrahyoid muscles. Arch Oral Biol. 2001;46:821-827.
82. Stal P, Marklund S, Thornell LE, et al. Fibre composition of human intrinsic tongue muscles. Cells Tissues Organs. 2003;173:147-161.
83. O’Reilly PM, FitzGerald MJ. Fibre composition of the hypoglossal nerve in the rat. J Anat. 1990;172:227-243.
84. Smith KK. Histological demonstration of muscle spindles in the tongue of the rat. Arch Oral Biol. 1989;34:529-534.
85. Tanaka T. Afferent projections in the hypoglossal nerve to the facial neurons of the cat. Brain Res. 1975;99:140-144.
86. Nakamura Y, Goldberg LJ, Mizuno N, et al. Effects of hypoglossal afferent stimulation on masseteric motoneurons in cats. Exp Neurol. 1978;61:1-14.
87. Lowe AA. Tongue movements: brainstem mechanisms and clinical postulates. Brain Behav Evol. 1984;25:128-137.
88. St John WM. Medullary regions for neurogenesis of gasping: noeud vital or noeuds vitals? J Appl Physiol. 1996;81:1865-1877.
89. St John WM. Rostral medullary respiratory neuronal activities of decerebrate cats in eupnea, apneusis and gasping. Respir Physiol. 1999;116:47-65.
90. Fregosi RF, Fuller DD. Respiratory-related control of extrinsic tongue muscle activity. Respir Physiol. 1997;110:295-306.
91. Fuller D, Mateika JH, Fregosi RF. Co-activation of tongue protrudor and retractor muscles during chemoreceptor stimulation in the rat. J Physiol. 1998;507:265-276.
92. Fuller DD, Williams JS, Janssen PL, et al. Effect of co-activation of tongue protrudor and retractor muscles on tongue movements and pharyngeal airflow mechanics in the rat. J Physiol. 1999;519:601-613.
93. Sauerland EK, Mizuno N. A protective mechanism for the tongue: suppression of genioglossal activity induced by stimulation of trigeminal proprioceptive afferents. Experientia. 1970;26:1226-1227.
94. Hellstrand E. Reflex control of cat extrinsic and intrinsic tongue muscles exerted by intraoral receptors. Acta Physiol Scand. 1982;115:245-256.
95. Ishiwata Y, Ono T, Kuroda T, et al. Jaw-tongue reflex: afferents, central pathways, and synaptic potentials in hypoglossal motoneurons in the cat. J Dent Res. 2000;79:1626-1634.
96. Ishiwata Y, Hiyama S, Igarashi K, et al. Human jaw-tongue reflex as revealed by intraoral surface recording. J Oral Rehabil. 1997;24:857-862.
97. Mattes RD. Nutritional implications of the cephalic-phase salivary response. Appetite. 2000;34:177-183.
98. Anderson DJ, Hector MP. Periodontal mechanoreceptors and parotid secretion in animals and man. J Dent Res. 1987;66:518-523.
99. Travers JB, Travers SP, Norgren R. Gustatory neural processing in the hindbrain. Annu Rev Neurosci. 1987;10:595-632.
100. Gjorstrup P. Taste and chewing as stimuli for the secretion of amylase from the parotid gland of the rabbit. Acta Physiol Scand. 1980;110:295-301.
101. Katschinski M. Nutritional implications of cephalic phase gastrointestinal responses. Appetite. 2000;34:189-196.
102. Teff K. Nutritional implications of the cephalic-phase reflexes: endocrine responses. Appetite. 2000;34:206-213.
103. Secchi A, Caldara R, Caumo A, et al. Cephalic-phase insulin and glucagon release in normal subjects and in patients receiving pancreas transplantation. Metabolism. 1995;44:1153-1158.
104. Goldfine ID, Ryan WG, Schwartz TB. The effect of glucola, diet cola and water ingestion on blood glucose and plasma insulin. Proc Soc Exp Biol Med. 1969;131:329-330.
105. Grill HJ, Berridge KC, Ganster DJ. Oral glucose is the prime elicitor of preabsorptive insulin secretion. Am J Physiol. 1984;246:R88-R95.
106. Lorentzen M, Madsbad S, Kehlet H, et al. Effect of sham-feeding on glucose tolerance and insulin secretion. Acta Endocrinol (Copenh). 1987;115:84-86.
107. Teff KL, Levin BE, Engelman K. Oral sensory stimulation in men: effects on insulin, C-peptide, and catecholamines. Am J Physiol. 1993;265:R1223-R1230.
108. Powley TL. Vagal circuitry mediating cephalic-phase responses to food. Appetite. 2000;34:184-188.
109. Gebruers EM, Hall WJ, O’Brien MH, et al. Signals from the oropharynx may contribute to the diuresis which occurs in man to drinking isotonic fluids. J Physiol. 1985;363:21-33.
110. Hiiemae KM. Masticatory movements in primitive mammals. In: Anderson DJ, Matthews B, editors. Mastication. Bristol, England: John Wright & Sons, 1976.
111. Luschei ES, Goldberg LJ. Neural mechanisms of mandibular control: mastication and voluntary biting. In: Handbook of Physiology. Baltimore: Williams & Wilkins; 1981.
112. Hiiemae K, Heath MR, Heath G, et al. Natural bites, food consistency and feeding behaviour in man. Arch Oral Biol. 1996;41:175-189.
113. Palmer JB, Hiiemae KM, Liu J. Tongue-jaw linkages in human feeding: a preliminary videofluorographic study. Arch Oral Biol. 1997;42:429-441.
114. Lund JP, Enomoto S. The generation of mastication by the mammalian central nervous system. In: Cohen A, Rossignol S, Grillner S, editors. Neural Control of Rhythmic Movements in Vertebrates. New York: John Wiley & Sons, 1988.
115. Plesh O, Bishop B, McCall W. Effect of gum hardness on chewing pattern. Exp Neurol. 1986;92:502-512.
116. Karlsson S, Carlsson GE. Characteristics of mandibular masticatory movement in young and elderly dentate subjects. J Dent Res. 1990;69:473-476.
117. Stohler CS, Ash MMJr. Demonstration of chewing motor disorder by recording peripheral correlates of mastication. J Oral Rehabil. 1985;12:49-57.
118. Lund JP. The importance of reflexes and their control during jaw movement. Trends Neurosci. 1983;6:458-463.
119. Chen Z, Travers SP, Travers JB. Muscimol infusions in the brain stem reticular formation reversibly block ingestion in the awake rat. Am J Physiol Regul Integr Comp Physiol. 2001;280:R1085-1094.
120. Travers JB, Norgren R. Afferent projections to the oral motor nuclei in the rat. J Comp Neurol. 1983;220:280-298.
121. Travers JB, DiNardo LA, Karimnamazi H. Motor and premotor mechanisms of licking. Neurosci Biobehav Rev. 1997;21:631-647.
122. Weijnen JA, Wouters J, van Hest JM. Interaction between licking and swallowing in the drinking rat. Brain Behav Evol. 1984;25:117-127.
123. McNamara JAJr, Moyers RE. Electromyography of the oral phase of deglutition in the Rhesus monkey (Macaca mulatta). Arch Oral Biol. 1973;18:995-1002.
124. Hrycyshyn AW, Basmajian JV. Electromyography of the oral stage of swallowing in man. Am J Anat. 1972;133:333-340.
125. Preiksaitis HG, Mayrand S, Robins K, et al. Coordination of respiration and swallowing: effect of bolus volume in normal adults. Am J Physiol. 1992;263:R624-R630.
126. Paydarfar D, Gilbert RJ, Poppel CS, et al. Respiratory phase resetting and airflow changes induced by swallowing in humans. J Physiol. 1995;483:273-288.
127. Dick TE, Oku Y, Romaniuk JR, et al. Interaction between central pattern generators for breathing and swallowing in the cat. J Physiol. 1993;465:715-730.
128. McFarland DH, Lund JP. Modification of mastication and respiration during swallowing in the adult human. J Neurophysiol. 1995;74:1509-1517.
129. Fontana GA, Pantaleo T, Bongianni F, et al. Changes in respiratory activity induced by mastication in humans. J Appl Physiol. 1992;72:779-786.
130. Kawamura Y, Kare MR. Umami: a basic taste. New York: Marcel-Dekker; 1987.
131. Bartoshuk LM, Gent J, Catalanotto FA, et al. Clinical evaluation of taste. Am J Otolaryngol. 1983;4:257-260.
132. Cowart B, Beauchamp G. Factors affecting acceptance of salt by human infants and children. In: Kare MR, Brand JG, editors. Interaction of the Chemical Senses with Nutrition. Orlando, Fla: Academic Press, 1986.
133. Travers SP, Nicklas K. Taste bud distribution in the rat pharynx and larynx. Anat Rec. 1990;227:373-379.
134. Witt M, Reutter K, Miller IJJr. Morphology of the peripheral taste system. Chapter 32. Doty RL, editor. Handbook of Olfaction and Gustation. New York: Marcel Dekker, 2003.
135. Beidler LM, Smallman RL. Renewal of cells within taste buds. J Cell Biol. 1965;27:263-272.
136. Miller IJJr. Variation in human fungiform taste bud densities among regions and subjects. Anat Rec. 1986;216:474.
137. Miller IJ, Bartoshuk LM. Taste percerption, taste bud distribution, and spatial relationships. and others. Getchell TV, editor. Smell and Taste in Health and Disease. New York: Raven Press, 1991.
138. Miller IJJr. Peripheral interactions among single papilla inputs to gustatory nerve fibers. J Gen Physiol. 1971;57:1-25.
139. Miller IJJr, Reedy FEJr. Quantification of fungiform papillae and taste pores in living human subjects. Chem Senses. 1990;15:281-294.
140. Smith DV, Davis BJ. Neural representation of taste. In: Finger TE, Silver WL, Restrepo D, editors. The Neurobiology of Taste and Smell. New York: Wiley-Liss, 2000.
141. Glendinning JI, Chaudhari N, Kinnamon SC. Taste transduction and molecular biology. Chapter 13. Finger TE, Silver WL, Restrepo D, editors. The Neurobiology of Taste and Smell. New York: Wiley-Liss, 2000.
142. Kinnamon SC. A plethora of taste receptors. Neuron. 2000;25:507-510.
143. Margolskee RF. Molecular mechanisms of bitter and sweet taste transduction. J Biol Chem. 2002;277:1-4.
144. Montmayeur JP, Matsunami H. Receptors for bitter and sweet taste. Curr Opin Neurobiol. 2002;12:366-371.
145. Heck GL, Mierson S, DeSimone JA. Salt taste transduction occurs through an amiloride-sensitive sodium transport pathway. Science. 1984;223:403-405.
146. Beidler LM. A theory of taste stimulation. J Gen Physiol. 1954;38:133-139.
147. Halpern BP. Amiloride and vertebrate gustatory responses to NaCl. Neurosci Biobehav Rev. 1998;23:5-47.
148. Hettinger TP, Frank ME. Specificity of amiloride inhibition of hamster taste responses. Brain Res. 1990;513:24-34.
149. Formaker BK, Hill DL. Alterations of salt taste perception in the developing rat. Behav Neurosci. 1990;104:356-364.
150. Geran LC, Garcea M, Spector AC. Transecting the gustatory branches of the facial nerve impairs NH(4)Cl vs. KCl discrimination in rats. Am J Physiol Regul Integr Comp Physiol. 2002;283:R739-R747.
151. Spector AC, Guagliardo NA, St John SJ. Amiloride disrupts NaCl versus KCl discrimination performance: implications for salt taste coding in rats. J Neurosci. 1996;16:8115-8122.
152. Schiffman SS, Lockhead E, Maes FW. Amiloride reduces the taste intensity of Na+ and Li+ salts and sweeteners. Proc Natl Acad Sci U S A. 1983;80:6136-6140.
153. Ossebaard CA, Polet IA, Smith DV. Amiloride effects on taste quality: comparison of single and multiple response category procedures. Chem Senses. 1997;22:267-275.
154. Ossebaard CA, Smith DV. Amiloride suppresses the sourness of NaCl and LiCl. Physiol Behav. 1996;60:1317-1322.
155. Lyall V, Alam RI, Phan DQ, et al. Decrease in rat taste receptor cell intracellular pH is the proximate stimulus in sour taste transduction. Am J Physiol Cell Physiol. 2001;281:C1005-C1013.
156. Stewart RE, Lyall V, Feldman GM, et al. Acid-induced responses in hamster chorda tympani and intracellular pH tracking by taste receptor cells. Am J Physiol. 1998;275:C227-C238.
157. Rouseff RL. Bitterness in Foods and Beverages. Amsterdam: Elsevier; 1990.
158. Adler E, Hoon MA, Mueller KL, et al. A novel family of mammalian taste receptors. Cell. 2000;100:693-702.
159. Chandrashekar J, Mueller KL, Hoon MA, et al. T2Rs function as bitter taste receptors. Cell. 2000;100:703-711.
160. Reed DR, Nanthakumar E, North M, et al. Localization of a gene for bitter-taste perception to human chromosome 5p15. Am J Hum Genet. 1999;64:1478-1480.
161. Wong GT, Gannon KS, Margolskee RF. Transduction of bitter and sweet taste by gustducin. Nature. 1996;381:796-800.
162. Caicedo A, Kim KN, Roper SD. Individual mouse taste cells respond to multiple chemical stimuli. J Physiol. 2002;544:501-509.
163. Nelson G, Hoon MA, Chandrashekar J, et al. Mammalian sweet taste receptors. Cell. 2001;106:381-390.
164. Li X, Staszewski L, Xu H, et al. Human receptors for sweet and umami taste. Proc Natl Acad Sci U S A. 2002;99:4692-4696.
165. Chaudhari N, Landin AM, Roper SD. A metabotropic glutamate receptor variant functions as a taste receptor. Nat Neurosci. 2000;3:113-119.
166. Chaudhari N, Yang H, Lamp C, et al. The taste of monosodium glutamate: membrane receptors in taste buds. J Neurosci. 1996;16:3817-3826.
167. Breslin P. Human gestation. Chapter 16. Finger TE, Silver WL, Restrepo D, editors. The Neurobiology of Taste and Smell. New York: John Wiley & Sons, 2000.
168. Chen Y, Herness MS. Electrophysiological actions of quinine on voltage-dependent currents in dissociated rat taste cells. Pflugers Arch. 1997;434:215-226.
169. Herness S. Coding in taste receptor cells: the early years of intracellular recordings. Physiol Behav. 2000;69:17-27.
170. Gilbertson TA, Bought JDJr, Zhang H, et al. Distribution of gustatory sensitivities in rat taste cells: whole-cell responses to apical chemical stimulation. J Neurosci. 2001;21:4931-4941.
171. Yee CL, Yang R, Bottger B, et al. “Type III” cells of rat taste buds: immunohistochemical and ultrastructural studies of neuron-specific enolase, protein gene product 9.5, and serotonin. J Comp Neurol. 2001;440:97-108.
172. Herness S, Zhao FL, Kaya N, et al. Adrenergic signalling between rat taste receptor cells. J Physiol. 2002;543:601-614.
173. Herness S, Zhao FL, Lu SG, et al. Expression and physiological actions of cholecystokinin in rat taste receptor cells. J Neurosci. 2002;22:10018-10029.
174. Zhang Y, Hoon MA, Chandrashekar J, et al. Coding of sweet, bitter, and umami tastes: different receptor cells sharing similar signaling pathways. Cell. 2003;112:293-301.
175. Zhao F, Lu S, Herness S. Novel transduction pathway for the artificial sweetener acesulfame-K potentially utilizes a TRP mediated chloride current. Association for Chemoreception Sciences Abstracts. 2003.
176. Miller IJJr, Reedy FEJr. Variations in human taste bud density and taste intensity perception. Physiol Behav. 1990;47:1213-1219.
177. Bealer SL, Smith DV. Multiple sensitivity to chemical stimuli in single human taste papillae. Physiol Behav. 1975;14:795-799.
178. Arvidson K, Friberg U. Human taste: response and taste bud number in fungiform papillae. Science. 1980;209:807-808.
179. Harper HW, Jay JR, Erickson RP. Chemically evoked sensations from single human taste papillae. Physiol Behav. 1966;1:319-325.
180. Collings VB. Human taste responses as a function of locus of stimulation on the tongue and soft palate. Percept Psychophys. 1974;16:169-174.
181. Spector AC, Schwartz GJ, Grill HJ. Chemospecific deficits in taste detection after selective gustatory deafferentation in rats. Am J Physiol. 1990;258:R820-R826.
182. Travers SP, Pfaffmann C, Norgren R. Convergence of lingual and palatal gustatory neural activity in the nucleus of the solitary tract. Brain Res. 1986;365:305-320.
183. Harada S, Yamamoto T, Yamaguchi K, et al. Different characteristics of gustatory responses between the greater superficial petrosal and chorda tympani nerves in the rat. Chem Senses. 1997;22:133-140.
184. Nejad MS. The neural activities of the greater superficial petrosal rat in response to chemical stimulation of the palate. Chem Senses. 1986;11:283-293.
185. Harada S, Smith DV. Gustatory sensitivities of the hamster’s soft palate. Chem Senses. 1992;17:37-51.
186. Lalonde E, Eglitis J. Number and distribution of taste buds on the epiglottis, pharynx, larynx, soft palate, and uvula in a human newborn. Anat Rec. 1961;140:91-95.
187. Frank ME. Taste-responsive neurons of the glossopharyngeal nerve of the rat. J Neurophysiol. 1991;65:1452-1463.
188. Iggo A, Leek BF. The afferent innervation of the tongue of the sheep. In: Hayashi T, editor. Olfaction and Taste II. London: Pergamon Press, 1967.
189. Oakley B, Jones LB, Kaliszewski JM. Taste responses of the gerbil IXth nerve. Chem Senses. 1979;4:79-87.
190. Shingai T, Beidler LM. Response characteristics of three taste nerves in mice. Brain Res. 1985;335:245-249.
191. Hellekant G, Danilova V, Ninomiya Y. Primate sense of taste: behavioral and single chorda tympani and glossopharyngeal nerve fiber recordings in the rhesus monkey, Macaca mulatta. J Neurophysiol. 1997;77:978-993.
192. Grill HJ, Norgren R. The taste reactivity test: I. mimetic responses to gustatory stimuli in neurologically normal rats. Brain Res. 1978;143:263-279.
193. Steiner JE. The gustofacial response: observation on normal and anencephalic newborn infants. Symp Oral Sens Percept. 1973;4:254-278.
194. Steiner JE, Glaser D, Hawilo ME, et al. Comparative expression of hedonic impact: affective reactions to taste by human infants and other primates. Neurosci Biobehav Rev. 2001;25:53-74.
195. Grill HJ, Schwartz GJ, Travers JB. The contribution of gustatory nerve input to oral motor behavior and intake-based preference: I. effects of chorda tympani or glossopharyngeal nerve section in the rat. Brain Res. 1992;573:95-104.
196. Travers JB, Grill HJ, Norgren R. The effects of glossopharyngeal and chorda tympani nerve cuts on the ingestion and rejection of sapid stimuli: an electromyographic analysis in the rat. Behav Brain Res. 1987;25:233-246.
197. St John SJ, Spector AC. Behavioral discrimination between quinine and KCl is dependent on input from the seventh cranial nerve: implications for the functional roles of the gustatory nerves in rats. J Neurosci. 1998;18:4353-4362.
198. Bradley RM, Stedman HM, Mistretta CM. Superior laryngeal nerve response patterns to chemical stimulation of sheep epiglottis. Brain Res. 1983;276:81-93.
199. Storey AT, Johnson P. Laryngeal water receptors initiating apnea in the lamb. Exp Neurol. 1975;47:42-55.
200. Dickman JD, Smith DV. Response properties of fibers in the hamster superior laryngeal nerve. Brain Res. 1988;450:25-38.
201. Miller AJ. Deglutition. Physiol Rev. 1982;62:129-184.
202. Bartoshuk L. Clinical evaluation of the sense of taste. Ear Nose Throat J. 1989;68:331-337.
203. Bull TR. Taste and the chorda tympani. J Laryngol Otol. 1965;79:479-493.
204. Jeppson P-H, Hallen O. The taste after operation for otosclerosis. Prac Otolrhinololaryngol. 1971;33:215-221.
205. Shafer DM, Frank ME, Gent JF, et al. Gustatory function after third molar extraction. Oral Surg Oral Med Oral Pathol Oral Radiol Endod. 1999;87:419-428.
206. Miyaoka Y, Sawada M, Sakaguchi T, et al. Sensation of thirst in normal and laryngectomized man. Percept Mot Skills. 1987;64:239-242.
207. Bartoshuk LM, Rifkin B, Marks LE, et al. Taste and aging. J Gerontol. 1986;41:51-57.
208. Fox AF. The relationship between chemical constitution and taste. Proc Natl Acad Sci U S A. 1932;18:115-120.
209. Guo SW, Reed DR. The genetics of phenylthiocarbamide perception. Ann Hum Biol. 2001;28:111-142.
210. Delwiche JF, Buletic Z, Breslin PA. Covariation in individuals’ sensitivities to bitter compounds: evidence supporting multiple receptor/transduction mechanisms. Percept Psychophys. 2001;63:761-776.
211. Prutkin J, Fisher EM, Etter L, et al. Genetic variation and inferences about perceived taste intensity in mice and men. Physiol Behav. 2000;69:161-173.
212. Bartoshuk LM, Duffy VB, Miller IJ. PTC/PROP tasting: anatomy, psychophysics, and sex effects. Physiol Behav. 1994;56:1165-1171.
213. Bartoshuk LM, Duffy VB, Reed D, et al. Supertasting, earaches and head injury: genetics and pathology alter our taste worlds. Neurosci Biobehav Rev. 1996;20:79-87.
214. Murphy C. Taste and smell in the elderly. In: Meiselman HL, Rivlin RS, editors. Clinical Measurement of Taste and Smell. New York: MacMillan, 1986.
215. Schiffmann SS. Age-related changes in taste and smell and their possible causes. In: Meiselman HL, Rivlin RS, editors. Clinical Measurement of Taste and Smell. New York: MacMillan, 1986.
216. Drewnowski A, Henderson SA, Hann CD, et al. Genetic taste markers and preferences for vegetables and fruit of female breast care patients. J Am Diet Assoc. 2000;100:191-197.
217. Basson MD. Colon cancer and genetic variation in taste, Association for Chemoreception Sciences Abstracts No. 435. 2003.
218. Chung CS, Runck DW, Niswander JD, et al. Genetic and epidemiologic studies of oral characteristics in Hawaii’s schoolchildren. J Dent Res. 1970;49:1374-1385.
219. Chung CS, Witkop CJ, Henry JL. A genetic study of dental caries with special reference to PTC taste sensitivity. Am J Hum Genet. 1964;16:231-245.
220. Lin BP. Caries experience in children with various genetic sensitivity levels to the bitter taste of 6-propylthiouracil (PROP): a pilot study. Pediatr Dent. 2003;25:37-42.
221. Beckstead RM, Norgren R. An autoradiographic examination of the central distribution of the trigeminal, facial, glossopharyngeal, and vagal nerves in the monkey. J Comp Neurol. 1979;184:455-472.
222. Hamilton RB, Norgren R. Central projections of gustatory nerves in the rat. J Comp Neurol. 1984;222:560-577.
223. Beckstead RM, Morse JR, Norgren R. The nucleus of the solitary tract in the monkey: projections to the thalamus and brain stem nuclei. J Comp Neurol. 1980;190:259-282.
224. Pritchard TC, Hamilton RB, Morse JR, et al. Projections of thalamic gustatory and lingual areas in the monkey, Macaca fascicularis. J Comp Neurol. 1986;244:213-228.
225. Baylis LL, Rolls ET, Baylis GC. Afferent connections of the caudolateral orbitofrontal cortex taste area of the primate. Neuroscience. 1995;64:801-812.
226. Small DM, Zald DH, Jones-Gotman M, et al. Human cortical gustatory areas: a review of functional neuroimaging data. NeuroReport. 1999;10:7-14.
227. Norgren R, Leonard CM. Taste pathways in rat brainstem. Science. 1971;173:1136-1139.
228. Norgren R, Leonard CM. Ascending central gustatory pathways. J Comp Neurol. 1973;150:217-237.
229. Kosar E, Grill HJ, Norgren R. Gustatory cortex in the rat: I. physiological properties and cytoarchitecture. Brain Res. 1986;379:329-341.
230. Kosar E, Grill HJ, Norgren R. Gustatory cortex in the rat: II. thalamocortical projections. Brain Res. 1986;379:342-352.
231. Norgren R. Taste pathways to hypothalamus and amygdala. J Comp Neurol. 1976;166:17-30.
232. Pfaffmann C, Frank M, Norgren R. Neural mechanisms and behavioral aspects of taste. Annu Rev Psychol. 1979;30:283-325.
233. Grill HJ, Norgren R. The taste reactivity test: II. mimetic responses to gustatory stimuli in chronic thalamic and chronic decerebrate rats. Brain Res. 1978;143:281-297.
234. Norgren R. Taste and the autonomic nervous system. Chem Senses. 1984;10:143-161.
235. Glenn JF, Erickson RP. Gastric modulation of gustatory afferent activity. Physiol Behav. 1976;16:561-568.
236. Baird JP, Travers SP, Travers JB. Integration of gastric distension and gustatory responses in the parabrachial nucleus. Am J Physiol Regul Integr Comp Physiol. 2001;281:R1581-R1593.
237. Hajnal A, Takenouchi K, Norgren R. Effect of intraduodenal lipid on parabrachial gustatory coding in awake rats. J Neurosci. 1999;19:7182-7190.
238. Jacobs KM, Mark GP, Scott TR. Taste responses in the nucleus tractus solitarius of sodium-deprived rats. J Physiol (Lond). 1988;406:393-410.
239. McCaughey SA, Giza BK, Scott TR. Activity in rat nucleus tractus solitarius after recovery from sodium deprivation. Physiol Behav. 1996;60:501-506.
240. Tamura R, Norgren R. Intracranial renin alters gustatory neural responses in the nucleus of the solitary tract of rats. Am J Physiol Regul Integr Comp Physiol. 2003;284:R1108-R1118.
241. Contreras RJ. Changes in gustatory nerve discharges with sodium deficiency: a single unit analysis. Brain Res. 1977;121:373-378.
242. Pittman DW, Contreras RJ. Rearing on basal or high dietary NaCl modifies chorda tympani nerve responses in rats. Physiol Behav. 2002;77:277-289.
243. Yaxley S, Rolls ET, Sienkiewicz ZJ, et al. Satiety does not affect gustatory activity in the nucleus of the solitary tract of the alert monkey. Brain Res. 1985;347:85-93.
244. Rolls ET, Scott TR, Sienkiewicz ZJ, et al. The responsiveness of neurones in the frontal opercular gustatory cortex of the macaque monkey is independent of hunger. J Physiol (Lond). 1988;397:1-12.
245. Rolls ET, Sienkiewicz ZJ, Yaxley S. Hunger modulates the responses to gustatory stimuli of single neurons in the caudolateral orbitofrontal cortex of the macaque monkey. Eur J Neurosci. 1989;1:53-60.
246. Burton MJ, Rolls ET, Mora F. Effects of hunger on the responses of neurons in the lateral hypothalamus to the sight and taste of food. Exp Neurol. 1976;51:668-677.
247. Yan J, Scott TR. The effect of satiety on responses of gustatory neurons in the amygdala of alert cynomolgus macaques. Brain Res. 1996;740:193-200.
248. Cabanac M, Minaire Y, Adair ER. Influence of internal factors on the pleasantness of a gustative sweet sensation. Comm Behav Biol A. 1968;1:77-82.
249. Rolls BJ, Rolls ET, Rowe EA, et al. Sensory specific satiety in man. Physiol Behav. 1981;27:137-142.
250. Rolls BJ, Rowe EA, Rolls ET, et al. Variety in a meal enhances food intake in man. Physiol Behav. 1981;26:215-221.
251. Perros P, MacFarlane TW, Counsell C, et al. Altered taste sensation in newly-diagnosed NIDDM. Diabetes Care. 1996;19:768-770.
252. Settle RG. Diabetes mellitus and the chemical senses. In: Meiselman HL, Rivlin RS, editors. Clinical Measurement of Taste and Smell. New York: MacMillan, 1991.
253. Ninomiya Y, Sako N, Imai Y. Enhanced gustatory neural responses to sugars in the diabetic db/db mouse. Am J Physiol. 1995;269:R930-R937.
254. Baylis LL, Rolls ET. Responses of neurons in the primate taste cortex to glutamate. Physiol Behav. 1991;49:973-979.
255. Rolls ET, Critchley HD, Wakeman EA, et al. Responses of neurons in the primate taste cortex to the glutamate ion and to inosine 5′-monophosphate. Physiol Behav. 1996;59:991-1000.
256. Plata-Salaman CR, Scott TR, Smith-Swintosky VL. Gustatory neural coding in the monkey cortex: L-amino acids. J Neurophysiol. 1992;67:1552-1561.
257. Nishijo H, Ono T, Norgren R. Parabrachial gustatory neural responses to monosodium glutamate ingested by awake rats. Physiol Behav. 1991;49:965-971.
258. Gilbertson TA, Fontenot DT, Liu L, et al. Fatty acid modulation of K+ channels in taste receptor cells: gustatory cues for dietary fat. Am J Physiol. 1997;272:C1203-C1210.
259. Rolls ET, Critchly HD, Browning AS, et al. Responses to the sensory properties of fat of neurons in the primate orbitofrontal cortex. J Neurosci. 1999;19:1532-1540.
260. Verhagen JV, Rolls ET, Kadohisa M. Neurons in the primate orbitofrontal cortex respond to fat texture independently of viscosity. J Neurophysiol. 2003;90:1514-1525.
261. McBurney DH, Pfaffmann C. Gustatory adaptation to saliva and sodium chloride. J Exp Psychol. 1963;65:523-529.
262. Christensen CM. Role of saliva in human taste perception. In: Meiselman HL, Rivlin RS, editors. Clinical Measurement of Taste and Smell. New York: MacMillan, 1986.
263. Johnson BF, Shakar V, Woodman T, et al. Salivary concentrations of pirmenol as a possible cause of unpleasant taste. Br J Clin Pharmacol. 1986;22:613-615.
264. Henkin RI, Talal N, Larson AL, et al. Abnormalities of taste and smell in Sjögren’s syndrome. Ann Intern Med. 1972;76:375-383.
265. Cano J, Rodriguez-Echandia EL. Degenerating taste buds in sialectomized rats. Acta Anat (Basel). 1980;106:487-492.
266. Nanda R, Catalanotto FA. Long-term effects of surgical desalivation upon taste acuity, fluid intake, and taste buds in the rat. J Dent Res. 1981;60:69-76.
267. Christensen CM, Navazesh M, Brightman VJ. Effects of pharmacologic reductions in salivary flow on taste thresholds in man. Arch Oral Biol. 1984;29:17-23.
268. Mossman KL. Gustatory tissue injury in man. radiation dose response relationships and mechanisms of taste loss. Br J Cancer Suppl. 1986;7:9-11.
269. Conger AD, Wells MA. Radiation and aging effect on taste structure and function. Radiat Res. 1969;37:31-49.
270. Bartoshuk LM. Chemosensory alterations and cancer therapies. NCI Monogr. 1990;9:179-184.
271. Bernstein IL, Webster MM. Learned taste aversions in humans. Physiol Behav. 1980;25:363-366.
272. Chambers KC. A neural model for conditioned taste aversions. Annu Rev Neurosci. 1990;13:373-385.
273. Lund JP. Mastication and its control by the brain stem. Crit Rev Oral Biol Med. 1991;2:33-64.
274. Nakamura T, Ogawa H. Neural interaction between cortical taste neurons in rats: a cross-correlation analysis. Chem Senses. 1997;22:517-528.
275. Nakamura Y, Sessle BJ. Neurobiology of mastication: from molecular to systems approach, International congress series, No. 1186:xvii, 525. 1999.