Physiology of the Cardiovascular System
A Primary vehicle of transport of substances in the body.
1. Respiratory gases (e.g., oxygen and carbon dioxide)
2. Circulating antibodies and leukocytes involved in the body’s defense mechanisms
3. Platelets and clotting factors involved in hemostasis
4. Cellular nutrients to all of the cells
5. Cellular waste products away from the cells
6. Electrolytes, proteins, water, and hormones, all of which contribute to the numerous complex functions of blood.
II Anatomic Classification of the Vascular Bed (Figure 10-1)
A The typical vascular bed begins with the aorta or pulmonary artery.
B Branches from either of these main arteries are called large arteries.
C The larger arteries continue to branch to medium arteries.
D The medium arteries branch further to the arterioles.
E The end of the arteriolar bed is marked by a thick band of smooth muscle called the precapillary sphincter, which marks the initial portion of the microcirculation.
F The arterioles branch to metarterioles or directly to capillaries.
G Distal to the precapillary sphincter are the capillaries.
H Many capillaries join to form venules.
I Numerous venules join to form small veins, which in turn join to form large veins.
J Large veins join the major veins of the body, either the superior vena cava, inferior vena cava, or pulmonary veins.
III Functional Divisions of the Vascular Bed
A Distribution, resistance, exchange, and capacitance vessels
1. Distribution vessels begin with the major arteries and include the large and medium arteries.
a. These vessels distribute the cardiac output (CO) to the various organ systems.
b. These vessels typically are under an elevated pressure, contain a relatively small percentage of total blood volume, and are highly elastic.
2. Resistance vessels begin with the arterioles and end with the precapillary sphincter.
a. These vessels have the largest proportion of smooth muscle constituting the vascular wall of any of the blood vessels.
b. Through contraction and relaxation of the smooth muscle, the resistance vessels can regulate the distribution of blood to the various capillary beds.
c. The resistance vessels are the major source of peripheral resistance and predominantly function in arterial blood pressure regulation.
3. The exchange vessels are the capillaries.
a. Fluid, gas, nutrient, and waste exchange occurs in these vessels.
b. Exchange of these substances occurs between capillary blood and interstitial fluid. Exchange then occurs from the interstitial fluid to the cells that make up the tissue.
c. The major process underlying exchange in the capillaries is diffusion.
d. Because of the vast distribution of capillary beds, the process of diffusion is sufficiently fast enough to maintain cellular metabolism.
4. Capacitance vessels include the venules through the large veins and encompass the total venous system.
a. Capacitance vessels serve as channels for blood return to the heart from the various capillary beds.
b. These vessels are called capacitance, or reservoir, vessels because they contain most (70% to 75%) of the total blood volume.
c. The capacitance vessels are typically under low pressure, contain a large blood volume, and are relatively inelastic compared with their arterial counterparts.
5. Distribution (volume) of blood in the components of the vascular system varies widely depending on the function of the component (Table 10-1).
TABLE 10-1
Estimated Distribution of Blood in Vascular System of the Hypothetical Adult Man
Volume | ||
Region | ml | % |
Heart (diastole) | 360 | 7.2 |
Pulmonary | ||
Arteries | 130 | 2.6 |
Capillaries | 110 | 2.2 |
Veins | 200 | 4.0 |
Subtotal | 440 | 8.8 |
Systemic | ||
Aorta and large arteries | 300 | 6.0 |
Small arteries | 400 | 8.0 |
Capillaries | 300 | 6.0 |
Small veins | 2300 | 46.0 |
Large veins | 900 | 18.0 |
Subtotal | 4200 | 84.0 |
Grand total | 5000 | 100 |
Age, 40 years; weight, 75 kg; surface area, 1.85 m2.
From Mountcastle VB: Medical Physiology, ed 14. St. Louis, Mosby, 1980.
IV Vascular System: Systemic and Pulmonary Circulations (Figure 10-2)
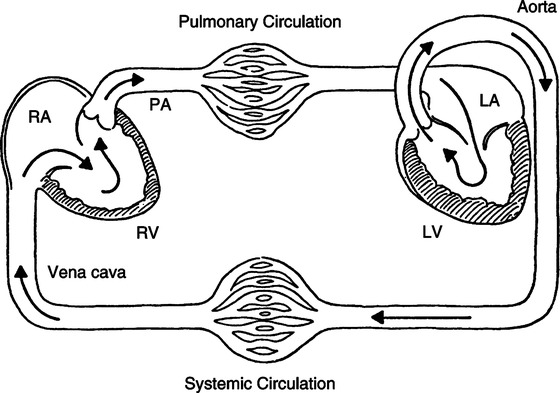
1. Systemic circulation begins with the systemic pump, the left ventricle, and continues to a typical vascular bed, ending with the right atrium.
a. Functions of systemic circulation:
(1) To distribute left ventricular CO so that each region of the body receives an adequate volume of blood per unit time.
(2) To perfuse individual tissues so that cellular metabolism is maintained.
(3) To return venous blood to the right side of the heart to maintain right ventricular output.
b. The velocity of the blood flow varies inversely with the total cross-sectional area through which blood flows at a given time (Figure 10-3). This physical law, coupled with the architecture of the vascular system, nicely accomplishes the three functions of the systemic circulation.
(1) The velocity of the blood flowing through the aorta is fast.
(2) The velocity of blood flow progressively slows from the arteries to arterioles.
(3) As the extraordinarily large cross-sectional area of the systemic capillaries is encountered, the velocity of blood flow attains it slowest rate.
(4) The velocity of the blood flow progressively increases from venules to veins as the cross-sectional area markedly decreases.
(5) As a result blood is quickly distributed (arteries), spends the greatest amount of time in the parenchymal tissue of the circulation (capillaries) serving the metabolic needs of the tissues, and then is quickly returned (veins) for recirculation.
2. Control of systemic circulation is governed by four major mechanisms: autonomic control, hormonal control, local control, and mechanical factors.
a. The arterial portion of the systemic circulation is basically governed by three mechanisms: autonomic nervous system, hormonal control, and local control.
(1) Arteries and arterioles are innervated extensively and virtually exclusively by postganglionic fibers of the sympathetic nervous system.
(2) The arterial vasculature of different tissues varies in the degree of sympathetic innervation.
(a) The largest degree of sympathetic innervation is to the arterial vasculature perfusing the skin.
(b) The degree of sympathetic innervation steadily decreases through the arterial vasculature perfusing spleen, mesenteric vessels, and kidneys.
(c) A smaller degree of sympathetic innervation exists in the muscles.
(d) The least degree of sympathetic innervation exists in the vessels perfusing the heart and brain. Furthermore, these vessels have a small degree of parasympathetic innervation.
(3) Sympathetic stimulation of blood vessels results in smooth muscle contraction and vasoconstriction.
(a) This principally affects the resistance vessels because of their large component of smooth muscle.
(b) Tonic sympathetic stimulation of arterial blood vessels results in a given arteriolar caliber.
(i) Increased sympathetic stimulation above this tonic level results in vasoconstriction and an increase in resistance to flow through these vessels.
(ii) Decreased sympathetic stimulation below this tonic level results in vasodilation and a decrease in resistance to flow through these vessels.
(iii) Because of differing degrees of sympathetic innervation in the different tissues, general sympathetic stimulation results in varying degrees of vasoconstriction and varying vascular resistance from tissue to tissue and hence a corresponding varying amount of blood flow from tissue to tissue (Table 10-2).
TABLE 10-2
Estimated Distribution of Cardiac Output and Oxygen Consumption in Normal Human Subject* at Rest Under Usual Indoor Conditions
Blood Flow | Oxygen Uptake | ||||
Circulation | ml/min | % Total | Arteriovenous Oxygen Difference (vol%) | ml/min | % Total |
Splanchnic | 1400 | 24 | 4.1 | 58 | 25 |
Renal | 1100 | 19 | 1.3 | 16 | 7 |
Cerebral | 750 | 13 | 6.3 | 46 | 20 |
Coronary | 250 | 4 | 11.4 | 27 | 11 |
Skeletal muscle | 1200 | 21 | 8.0 | 70 | 30 |
Skin | 500 | 9 | 1.0 | 5 | 2 |
Other organs | 600 | 10 | 3.0 | 12 | 5 |
Total | 5800 | 100 | 4.0* | 234 | 100 |
From Mountcastle VB: Medical Physiology, ed 14. St. Louis, Mosby, 1980.
(4) Parasympathetic stimulation of the arterial vasculature of the brain and heart results in smooth muscle relaxation and vasodilation. This phenomenon results in a decrease in resistance to blood flow.
(5) The adrenomedullary hormones norepinephrine and epinephrine stimulate the α (alpha) receptors and produce vasoconstriction.
(6) Acidosis, hypoxemia, hypercarbia, and increased temperature produce local relaxation of smooth muscle in resistance vessels and resultant vasodilation.
b. The capillary bed of the systemic circulation is governed almost exclusively by local factors.
(1) In tissues where capillary blood flow is limited by arteriolar constriction, there is local accumulation of acid and carbon dioxide and a deficiency of oxygen.
(2) These local factors result in relaxation of the smooth muscle and local arteriolar dilation, which reestablishes blood flow.
(3) Blood flow removes the local accumulation of waste products and replenishes oxygen and nutrient supply, resulting in arteriolar constriction, which in turn limits blood flow.
(4) Thus the cycle repeats itself, providing blood flow to tissues intermittently to maintain cellular metabolism.
c. The veins of the systemic circulation are governed by the autonomic nervous system, hormonal factors, and mechanical factors.
(1) The veins are exclusively innervated by postganglionic fibers of the sympathetic nervous system.
(2) The veins have a less extensive innervation than do their arterial counterparts. However, unlike that of the arteries, sympathetic innervation of the venous vasculature does not vary from one tissue to the next.
(a) Thus sympathetic stimulation causes venoconstriction of all veins of the body.
(b) Generalized venoconstriction decreases the venous vascular space, resulting in increased venous return to the heart.
(c) Conversely, decreased sympathetic stimulation results in a decrease in venous tone and venodilation.
(d) Generalized venodilation increases the venous vascular space and decreases venous return to the heart.
(3) Adrenomedullary hormones epinephrine and norepinephrine mimic sympathetic stimulation and produce venoconstriction.
(4) Mechanical factors that affect the veins of the systemic venous system are the thoracoabdominal pump, skeletal muscle pump, and semilunar valves.
(a) The thoracoabdominal pump affects the veins by aiding venous return. This is accomplished by exposing the intrathoracic veins to the fluctuating subatmospheric pressure produced by spontaneous ventilation. Coupled with the fact that extrathoracic veins are surrounded by atmospheric or supraatmospheric pressure, venous return is enhanced.
(b) The veins in the limbs contain semilunar valves that prevent retrograde flow of blood. When skeletal muscle contracts, it compresses the veins, increasing venous pressure. Because these veins have valves, compression of vessels can squeeze blood in only one direction. This mechanism also is responsible for enhancing venous return.
3. Specific regional systemic circulations
a. Coronary circulation is most influenced by local and mechanical factors.
(1) Local metabolites are major determinants of perfusion.
(2) Seventy-five percent of coronary perfusion takes place during ventricular diastole when the myocardium is in a state of mechanical relaxation. Hence diastolic arterial pressure is the value most often monitored to assess the perfusion pressure at the openings of the coronary arteries. Diastolic pressures <60 mm Hg directly reduce coronary blood flow.
b. Cerebral circulation is most influenced by local factors.
c. Gastrointestinal/splanchnic/pancreatic/hepatic circulations
(1) Sympathetic influences are dominant.
(2) Venous systems of these circulations contain significant blood volumes and respond by increasing and decreasing venous return directly as a result of the amount of venomotor tone exerted by sympathetic input.
d. Renal and epidermal circulation are principally under significant sympathetic influence.
B Pulmonary circulation (see Figure 10-2)
1. Pulmonary circulation begins with the pulmonary pump (the right ventricle) and continues to a typical vascular bed, ending with the left atrium.
a. Functions of pulmonary circulation:
(1) To distribute right ventricular output to pulmonary capillaries, matching the alveolar ventilation with an adequate volume of blood per unit time (external respiration).
(2) To perfuse the cells of the lung parenchyma with nutrients and rid them of waste products.
(3) To return blood to the left side of the heart to maintain left ventricular output.
b. The velocity of the blood flow varies inversely with the total cross-sectional area through which blood flows at a given time. This physical law, coupled with the architecture of the vascular system, nicely accomplishes the three functions of the pulmonary circulation (see Figure 10-3).
(1) The velocity of the blood flowing through the pulmonary artery is fast.
(2) The velocity of blood flow progressively slows from the arteries to arterioles.
(3) As the extraordinarily large cross-sectional area of the pulmonary capillaries is encountered, the velocity of blood flow attains it slowest rate.
(4) The velocity of the blood flow progressively increases from venules to veins as the cross-sectional area markedly decreases.
(5) As a result blood is quickly distributed (arteries), spends the greatest amount of time in the parenchymal tissue of the pulmonary circulation (capillaries) performing external respiration, and then is quickly returned (veins) to the heart for recirculation.
2. Control of pulmonary circulation is governed by the same four mechanisms that affect systemic circulation.
a. The pulmonary vasculature generally has less smooth muscle and thinner walls than its counterpart in the systemic circulation.
b. This makes pulmonary circulation susceptible to mechanical factors (e.g., intrathoracic and alveolar pressures) and the effects of gravity (secondary to alterations in bodily position) on the distribution of blood flow.
c. Pulmonary vasculature responds to sympathetic stimulation just as does the systemic circulation but to a much lesser extent.
d. Three local factors that have profound effects on pulmonary resistance vessels are decreased alveolar Po2, hypoxemia, and acidemia. All three cause pulmonary vasoconstriction, with increased resistance to blood flow.
e. Adrenomedullary hormones produce pulmonary vasoconstriction but to a milder degree than in systemic circulation.
f. Thus most of the control of pulmonary circulation depends on passive response to mechanical factors and on local factors. This is in contrast to the dominance that the sympathetic nervous system displays in controlling systemic circulation.
3. Systemic vascular resistance is normally 6 to 10 times pulmonary vascular resistance.
V Basic Functions of the Heart (Figure 10-4)
A To impart sufficient energy to the blood to provide circulation through the vascular system.
1. As has been discussed, the vascular resistance of systemic circulation is much greater than that of pulmonary circulation. Therefore, the left side of the heart must create greater pressures than the right side to bring about a given flow.
2. The major principle of circulation (blood flow) is that for circulation to exist, there must be a pressure gradient. The heart must create the pressure head (P1) for the pressure gradient (P1 − P2) across the respective parts of the vasculature. Therefore for a given vascular resistance, blood flow is a direct function of the pressure head generated by the heart.
VI Mechanical Events of the Cardiac Cycle (Figure 10-5)
A Electrical events of the heart are precursors to the mechanical events of the heart.
1. If the heart is normal, depolarization of the atria causes atrial contraction (systole).
2. Depolarization of the ventricles causes ventricular contraction (systole).
3. Repolarization of the atria causes atrial relaxation (diastole).
4. Repolarization of the ventricles causes ventricular relaxation (diastole).
B Atrial systole (Figure 10-6).
1. Mechanical left and right atrial systole begins at the peak of the P wave of the electrocardiogram (ECG).
2. The decrease in size of the respective atria causes left atrial pressure to increase approximately 7 to 8 mm Hg and right atrial pressure to increase 5 to 6 mm Hg concurrently. The pressure differential from atria to ventricles causes blood to flow from the atria through the respective atrioventricular (AV) orifices to the ventricles.
3. Normal mean right and left atrial pressures are 0 to 8 mm Hg and 2 to 12 mm Hg, respectively.
4. Atrial systole accounts for 20% to 40% of the total ventricular volume. This figure depends on heart rate (HR) and atrial contractility. The remaining 60% to 80% of ventricular volume is a result of passive filling by venous return, highlighting its critical importance in maintaining adequate CO.
5. The atria are weak pumps compared with the ventricles and should be thought of as thin-walled blood reservoirs for the respective ventricles. The 20% to 40% of ventricular volume added by atrial systole is simply a priming of the ventricles before ventricular systole. Atrial systole is not essential for adequate ventricular filling, as can be demonstrated by atrial fibrillation or complete heart block.
6. Atrial systole increases the end-diastolic volume of each ventricle to approximately 145 ml. It also increases the end-diastolic pressure of the right and left ventricles to 2 to 8 mm Hg and 4 to 12 mm Hg, respectively. The ventricles are now prepared (loaded) for their subsequent contraction.
C Ventricular systole and atrial diastole (see Figure 10-6).
1. Mechanical left and right ventricular systole begins at the peak of the R wave of the ECG and coincides with atrial diastole.
2. Ventricular contraction increases intraventricular pressure. When pressure in the respective ventricles exceeds atrial pressure, the tricuspid and mitral valves close, producing the first, or S1, heart sound.
3. Aortic and pulmonic semilunar valves have been closed during ventricular diastole because pressure in the aorta and pulmonary artery has exceeded left and right ventricular pressure.
4. With AV and semilunar valves closed, the ventricles are functionally closed chambers. Contraction of the ventricle decreases their size and rapidly increases intraventricular pressure.
5. The first portion of ventricular systole is called isovolumetric contraction. It is characterized by the AV and semilunar valves being closed and by a rapid increase in intraventricular pressure without a concomitant change in intraventricular blood volume.
6. The second portion of ventricular systole is called the period of ejection. Ejection begins when left and right intraventricular pressure exceeds the pressure in the aorta and pulmonary artery, respectively. It should be noted that this point is the diastolic pulmonary artery and aortic pressure. Previous to ventricular systole, blood has been steadily leaving the pulmonary and systemic (aortic) arterial systems, and intraarterial pressures have been steadily decreasing in both. The lowest intraarterial pressure is attained just before actual ventricular ejection and is called diastolic pressure of the respective arteries. Normal diastolic pressure for the aorta and pulmonary artery is 60 to 90 mm Hg and 5 to 16 mm Hg, respectively.
7. The period of ejection is characterized by opening of the semilunar valves.
a. During this time intraventricular pressure steadily increases above intraarterial pressure, causing blood to leave the ventricles.
b. Intraventricular pressure attains its maximum value (ventricular systolic pressure), followed by a resultant increase in intraarterial pressure to its maximum value (arterial systolic pressure).
c. Normal right and left ventricular systolic pressure is 15 to 28 mm Hg and 90 to 140 mm Hg, respectively.
d. Normal pulmonary artery and aortic systolic pressure is 15 to 28 mm Hg and 90 to 140 mm Hg, respectively.
e. When ejection is complete, intraarterial pressure and retrograde blood flow cause closing of aortic and pulmonic semilunar valves. This produces the second, or S2, heart sound.
8. The total period of ejection causes a stroke volume (SV) of 70 ml to be added to each arterial system by the respective ventricles.
9. It should be noted that the end-diastolic volume of each ventricle is 145 ml and the SV is 70 ml. This results in a residual blood volume of each ventricle equal to 75 ml. The residual volume is called the end-systolic volume. The percentage of the end-diastolic volume that is ejected as the SV is termed the ejection fraction (EF). Expressed as an equation:
< ?xml:namespace prefix = "mml" />
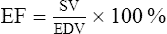
10. All previous blood pressure and volume values are based on the normal resting heart.
11. Closure of aortic and pulmonic semilunar valves marks the beginning of ventricular diastole.
D Ventricular diastole (see Figure 10-6).
1. Mechanical left and right ventricular diastole begins after completion of the T wave of the ECG.
2. Ventricular diastole begins with closure of the pulmonic and aortic semilunar valves and ends with onset of atrial systole.
3. Tricuspid and mitral valves have remained closed through the preceding ventricular systole and remain closed in early ventricular diastole. This is because intraventricular pressure exceeds intraatrial pressure.
4. The ventricles are functionally closed chambers with all cardiac valves remaining closed. Relaxation of the ventricular myocardium precipitates a large decrease in intraventricular pressure without a change in intraventricular blood volume, called isovolumetric relaxation.
5. When right and left intraventricular pressures decrease below the respective intraatrial pressures, the tricuspid and mitral valves open. This results in a rapid filling of each ventricle by intraatrial blood, followed by passive distention of the ventricles by blood returning from the lung and periphery.
6. It should be noted that blood will continue to passively fill the ventricles through the AV valves, which remain open until the onset of ventricular systole. This slow but steady addition to ventricular volume is evidenced by a small increase in intraatrial and intraventricular pressures.
7. The ventricular filling occurring during ventricular diastole accounts for 60% to 80% of the end-diastolic volume.
8. The entire myocardium remains relaxed until the onset of the P wave and atrial systole initiate another cardiac cycle.
E Summary of mechanical events of the cardiac cycle (Figure 10-7).
1. After the P wave of the ECG, the atria contract, propelling blood through the open AV valves to the ventricles.
2. During the height of the subsequent QRS complex, the ventricles contract in unison. It is during this same time that atrial relaxation occurs.
3. Intraventricular pressure soon increases above atrial pressure and causes the AV valves to close. This prevents retrograde flow of the blood from the ventricles to atria. Closure of the AV valves produces the S1 heart sound.
4. Intraventricular pressure continues to increase rapidly and soon exceeds intraarterial pressure. This causes the semilunar valves to open and provides blood flow from the ventricles to the arteries.
5. Relaxation of the ventricles occurs after completion of the T wave of the ECG.
6. As the ventricles relax, intraventricular pressure decreases below the respective intraarterial pressures. This causes the semilunar valves to close, preventing retrograde flow of blood from arteries to respective ventricles. Closure of the semilunar valves produces the S2 heart sound.
7. Intraventricular pressure continues to decrease until intraventricular pressure decreases below the intraatrial pressure. This causes the respective AV valves to open and provides blood flow from atria to ventricles.
8. Blood returning from pulmonary and systemic circulation continues to flow through the atria and open AV valves passively, filling the relaxed ventricles. This passive filling continues until the onset of the subsequent atrial contraction, which begins the next cardiac cycle.
A CO is the amount of blood pumped out of each ventricle over time.
B The CO of the right and left ventricles is equal and identical for a given period of time.
C The CO is equal to the SV times the HR:

1. The CO is conventionally expressed in liters per minute.
a. The normal range of CO in a resting individual is 4 to 8 L/min.
b. With stress or exercise, the CO can increase five to six times its normal resting value.
2. The SV is the amount of blood ejected from the ventricle with each ventricular systole.
a. The SV is expressed in milliliters per contraction.
b. The normal range for the SV of a resting individual is 60 to 130 ml per contraction.
3. The HR is the number of times the heart contracts per minute. The normal range for the HR of a resting individual is 60 to 100 contractions per minute.

5. It should be clear that increases in CO are brought about by increases in the HR and/or SV and that decreases in CO are brought about by decreases in HR and/or SV.
1. The pacemaker of the heart (SA node) sets the HR. The number of times per minute that the SA node depolarizes is largely governed by neural and chemical factors.
2. The neural factors that affect HR are mediated through the two divisions of the autonomic nervous system, namely, the parasympathetic and sympathetic nervous systems.
a. Parasympathetic impulses are conducted to the SA node through cranial nerve X (vagus nerve).
(1) Parasympathetic effects on the SA node are inhibitory and decrease the HR.
(2) Decreasing the HR is called negative chronotropism. Thus the parasympathetic nervous system exhibits negative chronotropic effects.
b. Sympathetic impulses are conducted to the SA node through sympathetic nerve fibers originating from the upper thoracic (T1 to T5) segment of the spinal cord.
(1) Sympathetic effects on the SA node are excitatory and increase the HR.
(2) Increasing the HR is called positive chronotropism. Thus, the sympathetic nervous system exhibits positive chronotropic effects.
c. The sympathetic and parasympathetic nervous systems are generally considered antagonists. However, in bringing about changes in HR, the two divisions of the autonomic nervous system complement each other.
(1) However, each nervous system can perform positive and negative chronotropism. Under certain clinical conditions, HR is altered by selective activities of one division of the autonomic nervous system.
(2) The parasympathetic nervous system generally is the dominant division of the neural input into the SA node. This is evidenced by total autonomic blockade, resulting in mild tachycardia.
d. Neural control of HR also is mediated through higher brain centers, such as the cerebral cortex and hypothalamus.
3. Major chemical factors that affect HR: electrolytes, exogenously administered drugs, and hormones.
a. The three major electrolytes affecting the HR are potassium, sodium, and calcium.
(1) Excess potassium and sodium decrease the HR.
(2) Excess calcium increases the HR.
(3) Potassium and sodium imbalance can alter cardiac membrane permeability and thus slow or speed the rate of electric conduction through the myocardium. However, only electrolyte imbalance brings about such changes in HR.
b. Classes of drugs that affect HR by either mimicking or inhibiting the activity of the sympathetic or parasympathetic nervous system:
(1) Sympathomimetics, such as isoproterenol, epinephrine, dobutamine, and dopamine, mimic the activity of the sympathetic nervous system and cause positive chronotropism.
(2) Sympatholytics, such as propranolol and metoprolol, inhibit the activity of the sympathetic nervous system and cause negative chronotropism.
(3) Parasympathomimetics, such as methacholine, pilocarpine, and neostigmine, mimic the activity of the parasympathetic nervous system and cause negative chronotropism.
(4) Parasympatholytics, such as atropine, inhibit the activity of the parasympathetic nervous system and cause positive chronotropism.
c. The major hormones that affect the HR are the adrenomedullary hormones.
4. HR is under many influences, ranging from conscious control by the cerebral cortex to exogenously administered pharmacologic agents. However, the most important regulatory control of HR is mediated through the autonomic nervous system.
1. Size of SV: Governed by preload, afterload, and state of contractility of ventricles.
a. Preload: Degree of ventricular diastolic filling before ejection begins, or the presystolic ventricular loading force.
(1) The Frank-Starling law states that the more the heart is filled during diastole, the greater the subsequent force of contraction. This results in increased SV (Figure 10-8), all other variables being equal.
(2) This relationship is related primarily to presystolic myocardial fiber length.
(3) Presystolic myocardial fiber length is directly related to end-diastolic volume because it is the actual intraventricular blood volume coupled with the compliance characteristics of the ventricle that results in myocardial fiber stretch.
(4) Because myocardial fiber length is virtually impossible to measure in the intact heart, it would seem that end-diastolic volume is an appropriate parameter to assess preload.
(a) The first factor affecting end-diastolic volume is the presence of a total blood volume sufficient for an effective vascular volume to vascular space relationship. There must be an adequate blood volume within the vascular space for the heart to circulate blood effectively. It is the relationship between vascular volume to vascular space that is crucial, not the absolute values of either vascular volume or vascular space.
(b) The state of the venous tone is the second factor affecting end-diastolic volume. The relationship between vascular volume and vascular space is essential to ensure adequate venous return and ventricular filling. Sixty percent to 80% of ventricular filling is accomplished by passive return of blood from the veins. The state of venous tone regulates venous vascular space, and it is therefore as crucial as blood volume in determining adequacy of venous return.
(c) The third major factor that affects end-diastolic volume is force of atrial systole. As mentioned, 20% to 40% of ventricular filling is accomplished by atrial systole. This is not of critical importance in the normal heart but becomes paramount in any cardiac dysfunction where ventricular compliance is decreased (i.e., ventricular hypertrophy or myocardial infarction).
(d) The fourth major factor that affects end-diastolic volume is compliance of the ventricle. As mentioned, this factor is not of importance in the normal heart, but decreases in ventricular compliance require a greater filling pressure per unit volume change. Thus increased filling pressure is necessary or the end-diastolic volume will have a reduced value.
(e) End-diastolic volume is an acceptable parameter for assessing preload; however, this too is difficult to measure with any accuracy in the intact heart. The most easily measured parameter that reflects preload is ventricular end-diastolic pressure.
(5) Given a constant ventricular compliance, it may be inferred that ventricular end-diastolic pressure should correlate well with ventricular end-diastolic volume. Accepting the latter as true, myocardial fiber length can be expressed as a function of end-diastolic pressure.
(a) In clinical practice, preload is assessed by measuring ventricular end-diastolic pressure.
(b) Right ventricular preload is assessed by end-diastolic pressure measurements taken from a central venous pressure (CVP) catheter as CVP (see Chapter 12).
(c) Left ventricular preload is assessed by end-diastolic pressure measurements taken from a pulmonary artery catheter as pulmonary wedge pressure (PWP; see Chapter 12).
(d) In general, the higher the end-diastolic pressure (preload), the greater the subsequent ventricular contraction and resulting SV.
(6) The Frank-Starling relationship is the basis for matching CO to venous return and balancing the output of right and left ventricles. For example, if venous return to the right atrium has suddenly increased because increased venous tone has altered the vascular volume/vascular space relationship:
(a) Increased venous return to the right ventricle would increase the right ventricular end-diastolic volume.
(b) Increased end-diastolic volume would result in an increased myocardial fiber length.
(c) Increased myocardial fiber length would in turn result in an increased force of contraction of the right ventricle.
(d) Increased force of contraction of the right ventricle would result in an increased right ventricular SV, all other factors remaining equal.
(e) Two phenomena have occurred. First venous blood has been mobilized back to the heart, and the increased venous return has been matched by an increase in right ventricular SV. This is one of the major ways that CO is increased. Second right ventricular output transiently exceeds left ventricular output.
(f) Increased right ventricular output will result in increased venous return to the left atrium and left ventricle.
(g) In similar fashion increased venous return to the left ventricle increases left ventricular end-diastolic volume.
(h) The increased end-diastolic volume would result in increasing the left ventricular myocardial fiber length. This in turn would result in an increase in the force of contraction of the left ventricle.
(i) The increased force of contraction of the left ventricle would result in an increased left ventricular SV.
(j) At this point venous blood has been mobilized from the venous reservoirs and has resulted in increased output from the right and left sides of the heart. Furthermore left ventricular output is in equilibrium with right ventricular output, all in accordance with the Frank-Starling relationship.
(k) The regulatory function of the Frank-Starling mechanism is frequently referred to as autoregulation because it is an intrinsic factor based on the architecture of the myocardial fibers, which automatically regulate CO to equal venous return. Thus it has led physiologists over the years to make the statement that within the physiologic limits of the heart, it will pump out all the blood it receives without allowing a backup of blood into the venous system.
b. Afterload: Resistance to flow from the ventricles.
(1) The work the heart must perform to pump blood out of the ventricles and into the circulation depends on three major factors: resistance of the semilunar valves, blood viscosity, and arterial blood pressure.
(a) As resistance of the semilunar valves (i.e., pulmonic or aortic stenosis) increases, afterload will be increased.
(b) As blood viscosity increases (i.e., hyperproteinemia or polycythemia), afterload will be increased.
(c) As arterial blood pressure increases (pulmonary or systemic hypertension), afterload will be increased.
(d) Decreases in any of these three parameters result in decreasing the afterload.
(2) Increases in afterload result in increases of ventricular work.
(a) The greater the resistance against which the ventricle must contract to eject blood, the more slowly it contracts.
(b) Increased afterload results in a decreased SV, which initially increases end-systolic volume. The normal venous return will then be added to the already increased end-systolic volume and increase the end-diastolic volume above normal. This allows a more forceful contraction against an increased afterload as a result of the Frank-Starling mechanism. This enables the ventricle to pump a given SV against an increased afterload. This compensation is at the expense of an increase in ventricular size. The larger the heart, the greater the work necessary to develop the myocardial tension required to produce a given intraventricular pressure (Laplace’s law).
(c) The slower rate of contraction and the increased ventricular size result in greater oxygen requirements to perform a given amount of work compared with that of the normal heart.
(3) Thus increases in afterload may or may not cause a decrease in SV but will cause increases in myocardial work.
(4) Decreases in afterload, commonly called afterload reduction, may or may not cause an increase in SV but will cause decreases in myocardial work (a cornerstone of post–myocardial infarction management).
(5) In general, the poorer the cardiac function, the more dependent the SV is on afterload.
(a) Increases in afterload tend to decrease SV in the patient with poor cardiac function.
(b) Increases in afterload do not cause decreases in SV in the patient with normal cardiac function.
(c) Decreases in afterload tend to increase SV in the patient with poor cardiac function.
(d) Decreases in afterload generally do not cause increases in SV in the patient with normal cardiac function.
(6) In the absence of valvular disease, afterload is clinically assessed by measuring mean arterial blood pressure.
c. State of ventricular contractility: Force with which the ventricles contract.
(1) The force of contraction of the ventricles at any given preload and afterload depends on the state of contractility (Figure 10-9).
(a) An increase in the state of ventricular contractility for a given preload and afterload is termed positive inotropism (see Figure 10-9, B).
(b) A decrease in the state of ventricular contractility for a given preload and afterload is termed negative inotropism (see Figure 10-9, C).
(c) The net effect of positive inotropism is generally a greater volume output per unit time.
(d) The net effect of negative inotropism is generally a smaller volume output per unit time.
(2) Ventricular contractility is altered by the sympathetic and parasympathetic nervous systems, blood gases (Po2, Pco2), pH, hormones, and exogenously administered drugs.
(3) The sympathetic nervous system extensively innervates the atrial and ventricular myocardium.
(a) Postganglionic nerve fibers of the sympathetic nervous system release norepinephrine to β (beta) receptor sites in the atrial and ventricular myocardium.
(b) This increases contractility of the myocardium (positive inotropism). Thus the sympathetic nervous system displays positive inotropic effects.
(c) Alterations in sympathetic discharge to the myocardium are believed to constitute the most important regulatory control of ventricular contractility.
(4) The parasympathetic nervous system only minutely innervates the atrial myocardium, and the ventricular myocardium is so innervated even more sparsely.
(a) Postganglionic nerve fibers of the parasympathetic nervous system release acetylcholine to the muscarinic receptor sites of the atrial and ventricular myocardium.
(b) As previously mentioned, because of the scantiness of parasympathetic innervation, the result is only a mild decrease in myocardial contractility or a slight negative inotropism. Thus the parasympathetic nervous system displays minor negative inotropic effects.
(5) Effects of blood gases on myocardial contractility:
(a) Mild decreases in Po2 result in increased contractility, whereas severe decreases in Po2 result in decreased myocardial contractility.
(b) Increases in Pco2 result in decreased contractility, whereas decreases in Pco2 result in increased myocardial contractility.
(c) Metabolic or respiratory acidosis results directly in decreased contractility, whereas metabolic and respiratory alkalosis may alter contractility through electric conduction dysfunction and arrhythmia.
(6) The most important hormones affecting myocardial contractility are the adrenomedullary hormones.
(a) The adrenal medulla secretes epinephrine and norepinephrine into the circulating blood.
(b) These two naturally occurring catecholamines have direct positive inotropic effects on the myocardium.
(c) The major differences between the effects of the catecholamines and direct sympathetic stimulation are that the effects of catecholamines take longer to establish but are longer lasting.
(d) The aforementioned statement is true of the effect of catecholamines on contractility and HR.
(7) The following exogenously administered drugs can alter myocardial contractility:
(a) The sympatholytics (e.g., propranolol and metoprolol) produce negative inotropic effects through β-receptor blockade.
(b) The sympathomimetics (e.g., isoproterenol, epinephrine, dobutamine, and dopamine) produce positive inotropic effects through β-receptor stimulation.
(c) Some antiarrhythmic agents (e.g., quinidine and procainamide) produce negative inotropic effects.
(d) Derivatives of digitalis produce positive inotropic effects.
(e) Calcium channel blockers (e.g., verapamil and nifedipine) produce negative inotropic effects.
(8) Myocardial contractility is an elusive parameter to assess clinically. However, controversial attempts have been made to quantitate it.
(9) It should be remembered that the SV is under a gamut of influences. However, any SV is determined by the interrelation of preload, afterload, and the state of ventricular contractility.
VIII Control of Arterial Blood Pressure
A Under normal circumstances, arterial blood volume exceeds arterial vascular space. This relationship results in an intravascular pressure dictated by the absolute arterial blood volume and the elastic properties of the arterial vasculature.
B The arterial system is continually receiving blood (inflow) from the left ventricle as CO and continually allowing blood to leave the arterial system (outflow) as arterial runoff.
C It is the balance or imbalance between CO (inflow) and arterial runoff (outflow) that results in any given arterial blood volume.
D The relationship between arterial blood volume and arterial vascular space is the primary determinant of arterial blood pressure.
E Thus by the equation P = () can be demonstrated that CO (
) and peripheral resistance (R) are directly related to arterial blood pressure (P). Arterial blood pressure depends on alteration of the blood volume to vascular space relationship by CO and/or peripheral resistance, as follows (Figure 10-10):
1. Increases in peripheral resistance (i.e., vasoconstriction) result in decreasing arterial runoff. If CO remains the same, inflow exceeds outflow from the arterial vasculature. This results in an increase in the arterial blood volume to vascular space relationship and a concomitant increase in arterial blood pressure.
2. Decreases in peripheral resistance (i.e., vasodilation) result in decreasing arterial blood pressure by the exact opposite mechanism.
3. Increases in CO result in increasing the rate of inflow to the arterial system. If peripheral resistance remains the same, inflow exceeds outflow from the arterial vasculature. This results in an increase in the arterial blood volume to vascular space relationship and an increase in arterial blood pressure.
4. Decreases in CO result in decreasing arterial blood pressure by the exact opposite mechanism.
5. It should be noted that in the previous four examples the imbalance between inflow and outflow is only temporary. It is by these mechanisms that arterial blood pressure can be increased or decreased. Once the desired arterial pressure is attained, the balance between inflow and outflow will maintain the pressure at that level.
F As has been described, CO and peripheral resistance are for a large part under neural control. Therefore it becomes apparent that regulation of arterial blood pressure is mediated through neural alterations in CO and peripheral resistance.
G Neural regulation of arterial blood pressure is mediated through autonomic fibers that originate from an area of the medulla oblongata. This area of the medulla is sometimes called the cardiovascular center.
H The cardiovascular center may be functionally divided into four subcenters: the vasomotor excitatory (vasoconstrictor) center, vasomotor inhibitory (vasodilator) center, cardiac excitatory center, and cardiac inhibitory center (Figure 10-11).
1. The vasomotor excitatory center influences the arterioles through the sympathetic nervous system. The degree of vasoconstriction or vasodilation is directly related to the amount of sympathetic stimulation.
2. The vasomotor inhibitory center does not influence the arterioles directly but acts by inhibiting the activity of the vasomotor excitatory center.
3. The cardiac excitatory center influences the heart through the sympathetic nervous system. Sympathetic stimulation originating from this center results in positive inotropic and chronotropic effects.
4. The cardiac inhibitory center influences the heart through the parasympathetic nervous system. Parasympathetic stimulation originating from this center results in a negative chronotropic effect and a mild negative inotropic effect.
I The cardiovascular center in the medulla receives sensory input from the entire body. The most important sources of sensory input are the exteroceptors, higher brain centers, local factors, peripheral chemoreceptors, and baroreceptors (see Figure 10-10).
1. Exteroceptors (e.g., proprioceptors, thermal receptors, and pain receptors) are sources of sensory input. Stimulation of the proprioceptors, pain receptors, and thermal receptors through muscular activation, pain, and heat, respectively, results in an increase in HR. This potentially will increase arterial blood pressure. Cold and muscular inactivity slow the HR and potentially decrease arterial blood pressure.
2. Higher brain centers (e.g., the cerebral cortex and hypothalamus) have medullary input.
a. Emotional factors alter blood pressure by mediation through the cerebral cortex. Fear or anger usually increases the blood pressure by stimulating the vasomotor and cardiac excitatory centers. This stimulation results in vasoconstriction and an increase in HR. However, decreases in blood pressure can be mediated through the cerebrum by stimulation of the vasomotor inhibitory center, as in fainting or blushing.
b. The hypothalamus mediates its control on the vasomotor inhibitory center in response to increases in body temperature. This causes vasodilation of the vessels of the skin and loss of body heat. A decrease in body temperature will result in vasoconstriction of the vessels of the skin with heat conservation as mediated through the hypothalamus.
c. Direct stimulation of the anterior portion of the hypothalamus produces bradycardia and a decrease in arterial blood pressure, whereas stimulation of the posterior portion of the hypothalamus produces tachycardia and an increase in arterial blood pressure.
3. The vasomotor inhibitory and excitatory centers are sensitive to local and direct effects of pH and Pco2 of arterial blood perfusing these centers.
a. Local increases in arterial pH and decreases in Pco2 cause depression of the vasomotor excitatory center by the vasomotor inhibitory center. This results in vasodilation, a decrease in peripheral resistance, and a decrease in arterial blood pressure.
b. Local decreases in arterial pH and increases in Pco2 cause direct excitation of the vasomotor excitatory center. This results in vasoconstriction, an increase in peripheral resistance, and an increase in arterial blood pressure.
c. A local decrease in Po2 of arterial blood potentiates the vasoconstrictor effect but alone has no local effect.
4. Peripheral chemoreceptors (aortic and carotid bodies) are responsible for initiating the vasomotor chemoreflex.
a. Hypoxemia, hypercapnia, and acidemia each stimulate the peripheral chemoreceptors.
b. The stimulated chemoreceptors in turn send an increased number of afferent impulses to the vasomotor excitatory center, with resultant vasoconstriction. This increases peripheral resistance and arterial blood pressure.
c. When hypoxemia and hypercapnia or hypoxemia and acidemia exist, the chemoreceptors display a synergistic effect (i.e., the stimulation originating from the chemoreceptors secondary to two simultaneous stimuli is greater than the mathematical sum of the two stimuli when they act alone). This results in a more profound vasoconstriction and an increase in blood pressure.
5. Baroreceptors are by far the most important short-acting regulator of arterial blood pressure.
a. Baroreceptors (pressoreceptors) are stretch receptors located in the arch of the aorta and carotid sinus.
b. They respond to changes in pressure, which stretch them to different degrees. The greater the pressure, the greater the number of impulses the baroreceptors will send.
c. With a decrease in arterial blood pressure, the number of impulses sent by the baroreceptors decreases. This decreased number of inhibitory impulses causes the vasomotor excitatory and cardiac excitatory centers to become more active. This results in increased CO and increased peripheral resistance, thus restoring arterial blood pressure to normal.
d. With an increase in arterial blood pressure, the number of impulses sent out by the baroreceptors increases. This increased number of inhibitory impulses causes the vasomotor excitatory center to become depressed directly and indirectly through increased activity of the vasomotor inhibitory center. The increased number of inhibitory impulses also depresses the cardiac excitatory center and stimulates the cardiac inhibitory center, resulting in a decreased HR. Thus peripheral resistance and CO decrease, restoring normal blood pressure.
J Long-term regulation of arterial blood pressure is accomplished by maintenance or alterations in the total blood volume.
1. Increases in total blood volume accomplished by renal fluid retention increase the venous vascular volume to space relationship and result in increased venous return.
a. Increased venous return increases end-diastolic volume and the resulting SV and CO.
b. The increased CO increases arterial blood volume and hence arterial blood pressure.
2. Conversely, decreases in total blood volume by renal fluid excretion decrease the venous vascular volume to space relationship and result in decreased venous return.