CHAPTER 155 Pharmacologic and Molecular Therapies of the Cochlear and Vestibular Labyrinth
Round Window Membrane
Anatomy
The round and oval windows sit on the medial wall of the middle ear. The conduction apparatus of the middle ear converges on the oval window, an arrangement designed to transfer the mechanical energy of sound waves into fluid waves that pass through the cochlea. Although situated at the tail end of this apparatus, the round window membrane (RWM) plays an essential role in acoustic dynamics because the compliance of the membrane allows for this mechanical energy to be released from the cochlea; without this outlet, no waves could travel through the perilymph. The actual RWM sits in the round window niche (fossula fenestrae cochleae) just posteroinferior to the promontory. When viewed from the intact tympanic membrane, the round window niche can be found an average of 3.44 mm (±0.68 mm) from the umbo, at an average angle of 113.2 degrees (±9.8 degrees) from the long process of the malleus.1
The connective tissue core contains fibroblasts, collagen, and elastic fibers, and houses blood and lymph vessels. The connective tissue layer is divided roughly into thirds, differing by fiber type and cellular material. Closest to the middle ear epithelium are coarse, loosely arranged collagen fibers, devoid of elastic fibers. In the middle of this layer, these fibers are joined by fibroblasts and ground substance, with occasional blood vessels and elastic fibers. Bordering the inner ear epithelium, there is a gradual increase in fibroblasts, collagen, and elastic fibers. As a whole, the connective tissue layer is responsible for providing compliance to the RWM. A discontinuous inner epithelial layer bathes in the perilymph of the scala tympani. Cells in this layer house pinocytotic vesicles and amorphous intracellular components, and feature long lateral extensions that bathe in the perilymph, suggesting that the RWM participates in some form of active transport.2 Figure 155-1 depicts the histologic complexity of the RWM.
Physiology
The RWM is a dynamic biologic membrane. All three layers of the RWM participate in a defensive response to pathogen insult. In the context of otitis media, the outer epithelial cells become hyperplastic, whereas blood vessels within the connective tissue layer become edematous and dilated, permitting extravasation of neutrophils and macrophages. Fibroblasts also become hyperplastic, displaying an increased volume of basophilic cytoplasm.2 Yoon and Hellstrom3 found that although all layers of the RWM are involved in the defense response, the most dramatic changes are seen in the subepithelial space close to the basement membrane (Fig. 155-2). Certain toxins can also initiate these metaplastic changes, resulting in a thicker RWM. The thickness of the RWM doubles after exposure to Pseudomonas exotoxin.4 Streptolysin O has been shown to cause breakdown of the RWM, increasing its permeability to substances in the middle ear space.5
Another dynamic aspect of the RWM is its ability to transport macromolecules. This process seems to be receptor mediated.6 Transport starts at the outer layer, where molecules are taken up by pinocytosis and are brought into the connective tissue layer. From there, substances either are absorbed by blood or lymphatic vessels or are mobilized further to the inner epithelial layer, where they are released by pinocytosis into the perilymph. The RWM may also participate in the absorption of perilymph because experimental evidence has shown the passage of tracer substances from the perilymph compartment into the RWM.2
The RWM displays dynamic changes as it ages. Although there is no change in the thickness of the RWM with aging, changes in cellular density and elastic fiber patterns can be seen. These changes may decrease the compliance of the membrane and compromise the overall function of the auditory system.7
Permeability
A large range of materials are able to cross the RWM, including various antimicrobials, steroids, anesthetics, tracers, albumin, horseradish peroxidase, latex spheres, germicidal solutions, water, ions, and macromolecules (including bacterial toxins).8 Several factors contribute to the permeability of the RWM, including size, charge, the morphology of the compound, and the thickness of the RWM. Size has proved to be a factor in permeability because 1-µm microspheres cross the RWM, but 3-µm microspheres do not.9 Substances with a molecular weight of less than 1000 kD diffuse across the RWM rapidly, whereas substances greater than 1000 kD are transported by pinocytosis.10 Charge of the molecule can also affect its ability to traverse the RWM: cationic ferritin crosses the RWM, but anionic ferritin does not.9 The implication is that liposolubility is a factor in RWM permeability, a feature that is important in the design of liposome vectors for gene therapy.8 The morphologic features of the compound can stimulate pinocytosis, presumably by a receptor-mediated mechanism.8,10 Increased thickness of the RWM decreases permeability of substances.8 Although the average thickness of the human RWM is 10 to 30 µm, this thickness can double in inflammatory conditions.11,12
RWM permeability can be altered with the use of exogenous adjuvants. Chandrasekhar and colleagues6 compared the ability of three exogenous adjuvants to increase the perfusion of dexamethasone applied to the round window niche. These compounds included histamine (for its vasodilatory effects), hyaluronic acid (for its proposed osmotic effect), and dimethyl sulfoxide (for its ability to increase medication solubility in perilymph). Histamine adjuvant with dexamethasone resulted in significantly higher perilymph steroid levels than all other combinations, whereas hyaluronic acid and dimethyl sulfoxide had no significant effect. Although this study failed to show an increase in steroid perfusion with the use of hyaluronic acid, several practitioners advocate use of hyaluronic acid in their intratympanic steroid protocols.1,13
Kinetics
Delivery of therapeutic agents across the RWM displays a nonuniform distribution in the perilymph. Concentrations of the delivered agent are usually high in the basal turns in close proximity to the RWM, and are low at the apical turns. The permeability characteristics of the substance across the RWM and the rate of its clearance from the perilymph are the two major factors that determine the dispersal characteristics of substances in perilymph; this can include a combination of clearance to blood, clearance to any other scala, uptake or binding by cells, or metabolism by any of the cochlear tissues.14 Knowing the RWM permeability and clearance rates of a given agent permits the simulation of its perilymphatic distribution with reasonable accuracy.
With these observations, Salt and Ma14 described the development of a computer-simulated model of drug distribution in the perilymphatic space (Fig. 155-3). Their model, the Washington University Cochlear Fluids Simulator, is a public-domain program that is available on the Internet at http://otowustl.edu/cochlea/. An example of the power of this program is provided in the study by Plontke and coworkers,15 in which a model of gentamicin kinetics in the perilymph was closely approximated to published in vivo kinetics data by adjusting input parameters defining RWM permeability, clearance, and interscala drug exchange. These investigators were able to establish that intratympanically administered gentamicin spreads from the RWM to the vestibule by communication through the scala, rather than by diffusion through the helicotrema. The study also suggested that drug concentrations and distribution in the perilymph were substantially influenced by the delivery method and the duration of exposure of the drug to the RWM. These computer-generated simulations are useful because they permit the optimization of different treatment protocols in humans, without resorting to simple trial and error or costly animal trials.
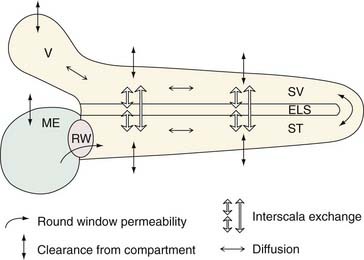
Figure 155-3. Schematic of the physical processes incorporated into the simulation program (the Washington University Cochlear Fluids Simulator, version 1.6, a public-domain computer program available at http://oto.wustl.edu/cochlea/). The compartments shown include the middle ear (ME), scala tympani (ST), cochlear endolymphatic space (ELS), scala vestibuli (SV), and vestibule (V). Drug enters through the round window (RW) in an amount depending on the permeability, and spreads longitudinally by diffusion. Local interscala exchange allows drug to spread to the endolymphatic space and the scala vestibuli, and from there to the vestibule. Drug clearance (losses to other compartments such as to blood) occurs from each compartment. Diffusion, clearance, and interscala exchange are calculated for each 0.1-mm segment of the fluid space.
(From Plontke SKR, Wood AW, Salt AN. Analysis of gentamicin kinetics in fluids of the inner ear with round window administration. Otol Neurotol. 2002;23:967.)
Another important finding from this simulated research is that the distribution of substances in perilymph is dramatically altered after perforation of the otic capsule. This finding is important because many previous pharmacokinetic studies have perforated the otic capsule to access perilymph for drug concentration sampling; their findings may be distorted by this phenomenon.14
Adhesions
As noted previously, the epithelium that neighbors the round window niche sometimes can create a veil that separates the RWM from the middle ear. Other reactive changes, such as scarring from repeated middle ear infections or from prior middle ear surgery, may also lead to adhesions that obstruct the RWM.16 In a cadaveric study of 202 ears to determine the rate and nature of RWM obstruction, Alzamil and Linthicum17 identified RWM obstruction in one third of ears. In their series, 21% had false RWMs, 10% had fibrous plugs, and 1.5% had fatty plugs. In cases in which temporal bones came from the same cadaver, 57% had no obstruction, 22% had bilateral obstruction, and 21% had unilateral obstruction. These investigators noted that the plugs filling the round window niche are 1 mm (in contrast to the RWM, which is approximately 20 µm thick).
Silverstein and coworkers16 provided further evidence of RWM obstruction. In a series of 41 patients, they found that 17% of ears had partial obstruction and 12% had total obstruction of the round window niche. Because these adhesions may cause significant variability in the pharmacokinetics of intratympanically applied medications, these authors recommended that endoscopic removal of these adhesions should be performed before any intratympanic drug treatments. In their practice, they use a 1.7-mm endoscope with 0-degree and 30-degree viewing angles. Adhesions, if identified, are removed with a small right-angle pick. The clinical and therapeutic advantages of lysing adhesions before intratympanic drug therapy have yet to be shown in controlled clinical trials.
Delivery Method
Following is a description of how typical intratympanic injections are performed. The patient lies flat with the affected ear facing the ceiling. The external ear canal is cleaned of debris, and the tympanic membrane is visualized with an operating microscope. The round window niche can be found an average of 3.44 mm (±0.68 mm) from the umbo, at an average angle of 113.2 degrees (±9.8 degrees) from the long process of the malleus (Fig. 155-4).18 Local anesthetic is applied to the tympanic membrane. Anesthetic preparations include topical tetracaine with alcohol, topical 15% phenol preparation, or ear canal injection of 1% lidocaine with 1 : 100,000 epinephrine.19 If endoscopy is to be performed, a generous myringotomy incision is made from the umbo posteriorly to the anulus, large enough to pass the endoscope (with scope diameters of approximately 1.7 to 2.4 mm). If simple injection is performed, myringotomy may still be necessary because there needs to be a vent for air to escape the middle ear space as it is filled with fluid.
Some protocols call for multiple injections over a short time. In these cases, it is useful to place a tympanostomy tube after the initial myringotomy. Montandon and colleagues20 found that placement of tympanostomy tubes resulted in resolution of vertigo in 71% of patients with Meniere’s disease in their study; if tubes are going to be used in a study, they should be acknowledged as a confounding variable.
Advances in microendoscopes may significantly increase the ease and accuracy of intratympanic drug delivery. Plontke and associates21 described the development of a 1.2-mm endoscope that incorporates a thin fiberoptic, a working/laser channel (0.3 mm), and a suction/irrigation channel (0.27 mm). This new device would allow for several manipulations to occur at once, including direct observation of the RWM, lysis of adhesions (if present), and application of medications directly to the RWM.
Intratympanic injection is inherently inaccurate because the injected medication can leak down the eustachian tube, escape out of the external canal, or be sequestered in the middle ear. The amount of medication delivered potentially changes with each patient and each dose.22 In an attempt to address this problem, several static sustained-release vehicles have been developed. A dry 2 mm × 3 mm Gelfoam (Upjohn, Kalamazoo, MI) pledget can be placed directly in the round window niche against the RWM. The treatment compound can be injected directly onto the Gelfoam pad. Because of the slow dissipation of Gelfoam, this injection can be repeated several times (as in titration protocols for gentamicin treatment of Meniere’s disease).19 Gelfoam slurry can also be used to suspend the medicine. This slurry can be directly injected into the middle ear space. This method has the advantage of the Gelfoam being easily removed from the middle ear in the case of an adverse reaction to the applied medicine.23
A two-component fibrin glue system also can be used (developed by Red Cross–Holland Lab, College Park, MD). The first component of the glue is deposited in the round window niche. The other component is mixed with the medicine and is added to the first (which is already in the round window niche). The two are mixed in situ and subsequently solidified, allowing the medicine to be slowly released from the glue onto the RWM.23
The ultimate degree of pharmacokinetic control is achieved with the use of mechanical sustained-release devices. These devices allow researchers to manipulate inner ear kinetic curves reliably by changing the rate and amount of dose delivered to the RWM.22 Two devices are currently approved for use in humans and have been studied in clinical trials: the Silverstein MicroWick (Micromedics, Eaton, MN) and the IntraEar Microcatheter (Durect, Cupertino, CA). The Silverstein MicroWick (Fig. 155-5) is made from polyvinyl acetate and measures 1 mm × 9 mm long, small enough to fit through a tympanostomy tube. The wick absorbs medication (which can be administered by the patient at home) that has been applied to the external ear canal and delivers it to the RWM. The advantage of this system is that fitting the device is a simple, minimally invasive procedure (only nominally more invasive than tympanostomy tube placement), and the device can be removed without anesthesia. Long-term use is not advised because the wick material may become adherent to the mucosa of the round window niche. This device has been used to deliver steroids and gentamicin treatments in human clinical trials.19
The IntraEar Microcatheter (Fig. 155-6) consists of an electronic pump (Disetronics, Minneapolis, MN) connected to a catheter tip that is placed directly on the RWM. Implantation of the microcatheter is more invasive and requires the elevation of a tympanomeatal flap. Several sizes are available to secure a good fit of the catheter tip into the round window niche. The rate and dose of drug delivery are set by the practitioner at the time of implantation, but can be adjusted in the middle of the treatment period. This device has also been extensively tested in human subjects for steroid and gentamicin therapy to the inner ear.24
Several new therapeutic agents (e.g., neurotrophins) require long-term or continuous application, which presents a serious challenge from a delivery point of view. In an attempt to address the need for a long-term delivery device, Praetorius and colleagues25 developed a fully implantable micropump system. The device is made from pure titanium, polyethylene, and silicone, and is designed for lifelong implantation in humans. To place the device, a cavity is drilled out of the mastoid bone to house the pump and reservoir system, a procedure that is similar to the one used to place cochlear implants or implantable hearing devices. The catheter tip is placed into the round window niche. The design allows for variation in kinetics, enabling bolus and continuous infusions. The device is designed so that it can be refilled by a simple procedure without the need for reimplantation.
Steroids
Mechanism of Action
Steroids mitigate the destructive processes caused by the immune response by decreasing the number of circulating blood leukocytes and inhibiting the formation and liberation of inflammatory mediators.26 They also inhibit the release of chemoattractive and vasoactive factors, decrease the secretion of lipolytic and proteolytic enzymes, and inhibit the release of proinflammatory cytokines, such as interferon-γ, granulocyte/monocyte colony-stimulating factor, interleukins, and tumor necrosis factor-α.27 These actions decrease the damage from an inflammatory response, whether the insult is secondary to mechanic, hypoxic, ischemic, infectious, or autoimmunologic causes.28
Several studies have established how steroids attenuate pathogen-induced immune responses in the ear. On exposure to lipopolysaccharide, cultured endothelial modiolar cells and tissue exhibit a generic response and release proinflammatory cytokines.29 These proinflammatory cytokines cause vasculitis, vascular leakage syndrome, entry of immunocompetent cells, and perivasculitis, ultimately leading to cochlear ischemia, intracochlear tissue damage, and hearing loss. Administration of dexamethasone can inhibit this cytokine immune response, and can potentially interrupt the beginnings of the inflammatory cascade at the level of cytokine expression.
Several other studies support the role of steroids in ion homeostasis in the inner ear. Serum glucocorticoid levels are directly correlated with activity and concentration of Na+,K+-ATPase in the inner ear.30 Lee and Marcus31 found that potassium secretion by marginal cells is immediately increased after the administration of steroids. Because the kinetics are too rapid for transcriptional activation to cause this change (it takes at least 30 minutes for RNA polymerase to be activated), a nongenomic mechanism is implicated. Modern theories of steroid pharmacology include not only nongenomic and genomic pathways of steroid hormone action, but also a nongenomic modulation of the genomic effects.32 These relationships in the inner ear are just beginning to be understood.
Pharmacokinetics
Intratympanic administration yields much higher concentrations of steroids in the inner ear than either intravenous or oral administration.6,33 Parnes and colleagues33 compared intravenous and intratympanic administration of hydrocortisone, methylprednisolone, and dexamethasone (short-acting, intermediate-acting, and long-acting steroids). Although all three steroids successfully penetrated the blood-labyrinthine barrier, there was a much higher concentration of steroids in inner ear tissues with intratympanic administration. Other investigators have shown that the metabolism of steroids, including uptake and elimination, is different in cochlear tissues compared with other organs.34 Methylprednisolone had the highest concentration and longest duration in perilymph and endolymph of the three compounds.33 Similar concentrations of steroids were found in the scala tympani and scala vestibuli. The authors argue that the concentration of steroids in the endolymph implies some form of active transport through the membranous labyrinth.
The findings regarding the superior concentrations of methylprednisolone are controversial. First, other forms of steroids have been tolerated much better by middle ear tissues. Dexamethasone seems to be better tolerated and less irritative to middle ear tissues. Second, higher concentrations have not led to superior clinical results. It may be that higher perilymph and endolymph concentrations do not translate into greater efficacy. In addition, although high methylprednisolone concentrations and high anti-inflammatory activity associated with attainable levels are observed with methylprednisolone, therapeutic efficacy may rely on other mechanisms of action. One possibility relates to Na+-K+ channel activity. The mineralocorticoid and glucocorticoid classes of steroids induce markedly different responses in Na+-K+ channel activity.31 At this point, it is reasonable to use the less morbid middle ear therapeutic agents (i.e., dexamethasone) until more definitive studies can determine whether the higher concentrations in the study by Parnes and colleagues33 translate into better clinical results, despite decreased tolerability.
Systemic Steroids
Currently, systemic steroids are the treatment of choice for sudden SNHL35,36 and acute vestibular vertigo.37 The most frequently used protocol of oral steroids for inner ear disease is 60 mg of prednisone (or 1 mg/kg/day for adults) taken for 10 to 14 days in idiopathic sudden SNHL or for 1 month in suspected autoimmune inner ear disease.38 Both indications call for a gradual taper after the initial treatment period is finished. If hearing loss returns during the taper, a higher dose of prednisone is restarted. Relapse of hearing loss is often preceded by tinnitus.39 Shea40 recommended that in addition to oral steroids, 16 mg of intravenous dexamethasone should be perfused over 3 hours. The value of adjunctive intravenous delivery of steroids in addition to oral therapy remains to be established.
Meniere’s Disease
Meniere’s disease may be due in some cases to immune dysfunction. Steroids are often used in Meniere’s disease treatment protocols.1 Itoh and Sakata41 reported the first intratympanic steroid protocol in 1987, in which four to five weekly injections of 2 mg of dexamethasone were administered to 61 patients with unilateral Meniere’s disease. This protocol resulted in relief of vertigo in 80% of patients and reduction in tinnitus in 74% of patients. Subsequently, additional studies have used intratympanic steroids to treat Meniere’s disease, some with more promising results than others.
Sennaroglu and colleagues42 placed tympanostomy tubes in 24 patients with Meniere’s disease with intractable vertigo and applied 5 drops of 1 mg/mL dexamethasone solution into the middle ear space every other day for 3 months (administered at home by the patient). This protocol resulted in a vertigo control rate of 72%, improved hearing in 17%, decreased tinnitus in 75%, and reduced aural fullness in 75%. This protocol is attractive because the drug is self-administered, the entire procedure can be accomplished under local anesthesia, and the delivery method provides flexibility to titrate the dose. The authors acknowledge that their results may be confounded by a placebo effect secondary to the tympanostomy tubes. They cite a study by Montandon and associates20 that reported that tympanostomy tube placement prevented vertigo attacks in 71% of patients.
Barrs and coworkers43 also used tympanostomy tubes to administer intratympanic dexamethasone. They used a dose of 0.3 to 0.5 mL of 4 mg/mL dexamethasone. Injections were given daily for the first 2 days and weekly thereafter for a total of 1 month and five treatments. The vertigo control responses were reported in time intervals, with an 86% response at less than 3 months, a 52% response at 3 months, and a 43% response at 6 months. There was an average of 2.7-dB hearing loss, but one patient had a 35-dB hearing loss. The authors propose that intratympanic steroid treatment is effective for short-term management of vertigo, but is less successful for long-term vertigo control.
Shea40 reported on a protocol that uses a combination of intravenous, intratympanic, and oral dexamethasone to treat patients with Meniere’s disease. A mixture of 0.5 mL hyaluronan containing 16 mg/mL of dexamethasone is injected into the middle ear after argon laser myringotomy and removal of RWM adhesions. The patient sits with the injected ear up for 3 hours while receiving 16 mg of intravenous dexamethasone. This treatment is performed for 3 consecutive days. Then 0.25 mg of oral dexamethasone is taken for 30 to 90 days, depending on the response to treatment. This protocol resulted in a vertigo control rate of 77%, hearing improvement in 35.4%, and hearing loss in 6.3%. With the 6-point functional level score recommended by the American Academy of Otolaryngology–Head and Neck Surgeons (AAO-HNS) guidelines,44 61.3% of patients were improved, 32.3% were unchanged, and 6.4% were worse after treatment.
In a rare controlled trial, Silverstein and colleagues1 conducted a prospective, randomized, double-blind, crossover trial of intratympanic dexamethasone and placebo in 17 patients with Meniere’s disease. All patients had stage IV Meniere’s disease by the Shea classification (they no longer had vertigo, had poor hearing, and had significant fullness and tinnitus). Patients received intratympanic injection of either placebo (0.2 to 0.3 mL of 1 : 1 normal saline and sodium hyaluronate) or 0.2 to 0.3 mL of a 1 : 1 mixture of 16 mg/mL dexamethasone and sodium hyaluronate. This treatment was performed for 3 consecutive days. Three weeks after the initial treatment, the groups received the crossover treatment (the placebo group received intratympanic steroids and vice versa). The parameters recorded were audiometric data, electronystagmography recordings, and tinnitus evaluations; several questionnaires and telephone interviews were included. Intratympanic steroids provided no significant benefit over placebo in any of the parameters recorded, and patients could not guess which arm of the study they were in. This study would seem to confirm the lack of benefit of intratympanic steroid use for Meniere’s disease. The severely diseased patient population (all stage IV) may be a selection bias not present in other studies, however, leaving open the possibility that intratympanic steroids may be useful in less severe cases. Also, most successful intratympanic steroid protocols involve steroid treatments that last longer than 3 days.
In another well-designed study, Garduño-Anaya and associates45 conducted a prospective, placebo-controlled, double-blind, randomized study with 2-year follow-up, and found dramatically different results than Silverstein and colleagues.1 A regimen of 5 consecutive days of intratympanic dexamethasone (4 mg/mL) versus saline for placebo control was administered to 11 study patients and 11 controls, all having unilateral disease as outlined by the 1995 AAO-HNS Committee on Hearing and Equilibrium. In the dexamethasone group at 2-year follow-up, complete control of vertigo (class A) was achieved in 9 of 11 patients (82%), and substantial control of vertigo (class B) was achieved in the remaining 2 patients. In the control group, only 7 of 11 patients finished the trial, and of these, 4 patients (57%) achieved class A vertigo control, 2 patients (29%) achieved class C, and 1 patient (14%) achieved class F. These results were statistically significant. There were several other outcome measures with variable results, with significant improvement in AAO-HNS 6-point functional level; Dizziness Handicap Inventory; and subjective vertigo, tinnitus, and aural fullness scores in the treatment group, but no significant changes in the Tinnitus Handicap Score, pure-tone average, speech discrimination score, electronystagmography, or electrocochleography tests between the two groups. The final verdict on intratympanic steroid use for Meniere’s disease is still pending, and more data on optimal dosing and protocols are needed.
Sudden Sensorineural Hearing Loss
Systemic and intratympanic steroid therapy has also been used for treatment of sudden SNHL. The major prognostic factors predicting response to treatment for sudden SNHL are initial severity of hearing loss and time between onset and treatment.6,46 There is a high spontaneous recovery rate of 30% to 60%; treatment efficacy of any intervention has to be greater than the spontaneous recovery rate. Oral steroid therapy within the first 2 weeks has shown recovery rates approaching 80% and decreasing thereafter.46,47 Because of the high initial response to oral steroids, few practitioners have attempted to use intratympanic steroids, and most intratympanic steroid trials enroll patients who had oral treatment fail. That being said, numerous studies, mostly retrospective, have shown that intratympanic steroids do provide an excellent method for salvage of hearing in the case of systemic steroid treatment failure.
Gianoli and Li35 reported results of a trial of intratympanic steroids for patients with sudden SNHL who had failed to improve after high-dose systemic steroids (1 mg/kg/day of prednisone for a minimum of 1 week). The protocol consisted of tympanostomy tube placement followed by instillation of 0.5 mL of steroid solution consisting of either 25 mg/mL of dexamethasone or 62.5 mg/mL of methylprednisolone. Four treatments were administered over 10 to 14 days, and audiometric data were recorded 1 to 2 weeks after treatment. The results showed a pure-tone average improvement of 10 dB or greater in 44% of patients. The authors argued that although this improvement seems modest, this is in a cohort of patients who would otherwise be considered refractory to steroid treatment. Although there was a trend toward better outcomes for methylprednisolone, there was no significant difference between the two steroid solutions.
Kopke and colleagues46 reported results of the use of methylprednisolone perfusion by means of an RWM microcatheter in patients with sudden SNHL who failed oral prednisone therapy. The catheter delivered 62.5 mg/mL of methylprednisolone at a continuous rate of 10 µL/hour for 14 days with an electronic pump. Audiometric changes were the main outcome measures recorded. Of the six patients who had catheter placement 6 weeks or less after the onset of hearing loss, five had improvements of 10 dB or more in pure-tone averages, and four of these had a return to baseline pure-tone averages. Results were less promising for patients receiving treatment more than 6 weeks after onset of symptoms; in one patient, there was additional hearing loss associated with vertigo.
Several other, smaller reports have been published. Chandrasekhar47 reported results from a series of 10 patients treated with intratympanic dexamethasone. The dexamethasone concentration and number of intratympanic injections varied among patients, and several patients were taking oral medications in addition to intratympanically administered steroids, making outcomes difficult to assess. Of the 10 patients treated, 6 experienced hearing improvements greater than 10 dB, however. Parnes and colleagues33 reported results from a similar series of 13 patients with sudden SNHL treated with intratympanic steroids. Because there was considerable variation in the number of treatments applied and the drug administered (dexamethasone vs. methylprednisolone), the results are difficult to assess. Of the 13 patients treated, 6 showed hearing improvements of 10 dB or more.
One point of consensus about these studies is that they show that the longer between the insult and the administration of intratympanic steroid treatment after oral steroid failure, the lower are the chances of salvaging hearing. If intratympanic steroids are to be used, they should be used as soon as possible after it becomes clear that oral steroids are not improving hearing, preferably within the first 2 weeks of the original insult.48
Dosing
The most widely used steroid for intratympanic protocols is dexamethasone, followed by methylprednisolone. Intratympanic dexamethasone preparations vary from 1 to 25 mg/mL.49,50 Some studies use a hyaluronic acid preparation consisting of a 1 : 1 mixture of 16 mg/mL of dexamethasone and 0.5 mg/mL of hyaluronate sodium.1,40 Most intratympanic methylprednisolone studies use a solution of 62.5 mg/mL.22,46 The amount of solution injected in each protocol is designed to fill the middle ear space (which is 0.3 to 0.5 mL). The interval of dosing depends mostly on the instillation method. Protocols that include self-administration through tympanostomy tubes have every-other-day dosing.49 Intratympanic injection protocols are much less frequent, and often include “shotgun” dosing with multiple injections over the first 2 weeks of treatment.1,51
Side Effects
The side effects of long-term systemic steroid use are well known and include compromise of the immune system leading to infections, osteoporosis, peptic ulcers, hypertension, myopathy, ocular effects, impaired healing, psychologic effects, and avascular necrosis.52 In contrast, intratympanic steroids are characterized by minor local morbidities. Several preclinical studies have documented that intratympanic steroids cause no morphologic or functional compromise in animal models.27,53 Human clinical trials have reported benign side-effect profiles, even after multiple and long-term treatments.33 There are several reports of decreased hearing in human clinical trials of patients with Meniere’s disease,40,43 but it is unclear whether this is a side effect of treatment or part of the natural course of the disease.51,54 There are some reports of tympanic membrane perforations and otitis media secondary to the perfusion process.35 Some patients experience a mild burning sensation in the ear after injection of methylprednisolone. This side effect has been avoided by combining 0.1 mL of 1% lidocaine with 0.9 mL of standard intravenous methylprednisolone solution (40 mg/mL).33
Gentamicin
Fowler55 first described the use of aminoglycosides for chemical ablation of the labyrinth in 1948, using systemic streptomycin to treat patients with Meniere’s disease with intractable vertigo. Bilateral cochlear damage led to the abandonment of this effort, but in 1957, Schuknecht56 revived interest in chemical ablation with the introduction of intratympanic administration of aminoglycosides. Although loss of hearing was almost as common as the resolution of vertigo, his work set the stage for the development of modern intratympanic chemical ablation protocols. In the mid-1970s, Beck and Schmidt57 described a low-dose strategy that departed from the goal of total vestibular ablation. They compared a high-dose ablative protocol with a low-dose, low-injection-frequency protocol, and found that although vertigo control was essentially the same, the hearing loss rate decreased from 58% to 15%. This improvement rekindled interest in intratympanic gentamicin therapy, and led to the development of strategies that maximize vertigo control, while minimizing hearing loss.
Mechanism of Action
Despite the widespread belief that gentamicin is selectively toxic to vestibular hair cells, this assertion is not fully supported by the literature. Several researchers have shown that gentamicin and streptomycin cause parallel and dose-dependent damage to vestibular and cochlear hair cells.58,59 Wanamaker and colleagues59 stated, “When the vestibular system was severely damaged, the cochlea was severely damaged; when loss in the vestibular system was mild, loss in the cochlea was mild. We did not observe any dose-related selectivity for the vestibular system over the cochlea.” The clinical finding of significant correlation between loss of caloric response and hearing loss after gentamicin exposure further betrays the notion of selective vestibular toxicity.60,61
If there is no selective vestibular toxicity, how is it that clinicians have achieved good vertigo control with minimal hearing loss? In part, the answer may lie in pharmacokinetics.22,62,63 Hoffer and colleagues22 compared the kinetic profiles of intratympanic injection versus microcatheter delivery of gentamicin, and correlated these data with the functional and morphologic changes observed in the inner ear. Despite the fact that the total dose in both methods was roughly equal, the resulting morphologic changes were quite different. Intratympanic injection led to erratic changes, sometimes causing obliteration of auditory functioning within 4 hours, and in other cases showing significantly delayed ototoxic effects. In contrast, controlled perfusion by microcatheter caused predictable and uniform damage.
Hoffer and colleagues22 explained that these different morphologic changes may be due to two different patterns of hair cell loss, patterns that correlate with the timing and concentration of aminoglycoside delivery. These patterns include a necrotic pattern that is associated with rapid and high-dose perfusion, and an apoptotic pattern that is associated with slower or chronic perfusion.53,64 This complicated relationship may be secondary to saturation of anionic binding sites on the cell membrane or to modifiable active uptake patterns that depend on gentamicin kinetics.65 In addition to the amount of gentamicin that accumulates in the perilymph over time, the nature of the distribution curve, timing of the peak, and total dose determine morphologic and functional consequences of aminoglycoside exposure.
What is the histologic and physiologic correlate of partial ablation? It is known that gentamicin damages type I hair cells more rapidly and severely than type II hair cells.66 In the chinchilla, investigators showed that type II hair cells regenerated to approximately 55% of the baseline population, whereas type I hair cells showed no regeneration at all. It may be that these patterns of hair cell destruction and regeneration describe the partial vestibular ablation seen in intratympanic gentamicin clinical trials. Carey and associates67 examined the changes in the angular vestibulo-ocular reflex after a single dose of intratympanic gentamicin, and found that in all cases there was a decreased, but not abolished, angular vestibulo-ocular reflex gain. These authors concluded that this finding provides evidence of incomplete hair cell destruction, which may account for the phenomenon of “partial ablation.” Minor68 speculated that there may be a critical level of vestibular activity required to initiate an episode of vertigo, and that the regions of vestibular epithelium or groups of afferent nerve fibers responsible for vertigo may be more susceptible to gentamicin toxicity than others. Minor68 also speculated that hair cell regeneration may explain the phenomenon of recurrent vertigo after initial control with intratympanic gentamicin.
Although selective hair cell destruction and regeneration have yet to be established in humans, this may explain some of the more puzzling findings documented in clinical trials. Numerous trials have reported that there is an increase in hearing after gentamicin treatment.61,69,70 In addition, in the microdose infusion trials by Hoffer and colleagues,71 vestibular function improved in 78% of patients. These findings are hard to reconcile with traditional models of aminoglycoside toxicity.
The alternative explanation for how gentamicin achieves a selective vestibular effect is the proposal that gentamicin primarily affects secretory, rather than sensory, cells; this is known as the dark cell theory. Although cited in almost every article related to intratympanic gentamicin treatment, there is no firm documented scientific evidence showing how the effect actually occurs. Vestibular dark cells are important (but not essential) in creating and maintaining ion homeostasis in the inner ear.72–74 Several studies have documented that aminoglycosides induce structural and functional alteration of these dark cells.19,75 This damage is thought to alter the ionic homeostasis of the inner ear in such a way that it brings the ionic dysregulation in endolymphatic hydrops back into balance.76,77 This theory helps explain how the hearing restoration and vestibular improvement referenced earlier might occur; re-establishment of ionic homeostasis would restore baseline function in the cochlea and labyrinth. There is uncertainty, however, whether this dark cell damage occurs before damage to sensory cells. Chen and colleagues58 looked at secretory and sensory cells in response to aminoglycoside challenge, and found no significant changes in dark cells, despite extensive damage in cochlear and vestibular hair cells in the same animal. If dark cells are responsible for the selective effect of gentamicin, this has yet to be established in any preclinical models. Explanation of this mechanism, if it exists, would be a great advance in otology.
Pharmacokinetics
Intratympanic gentamicin kinetics in the perilymph follow a one-compartment model.22,78 Gentamicin rapidly diffuses across the RWM; the kinetics of gentamicin in perilymph are largely determined by the method of delivery.22,78,79 Intratympanic injection has a faster absorption phase, shows higher peak concentrations, and exhibits significantly more variability in all measurements than sustained-release delivery methods.22,80 In pharmacokinetic studies, large value ranges and standard deviations are characteristic of intratympanic injection delivery (Fig. 155-7).22
Elimination of gentamicin from the perilymph compartment is rapid and dose-dependent.78 Because of this rapid elimination, a sustained-release vehicle for drug delivery, such as a fibrin-based glue80 or delivery by microcatheter, is the only way to maintain high concentrations of gentamicin in perilymph.22 Although elimination in perilymph is rapid, there seems to be significant uptake of gentamicin in inner ear tissues, essentially constituting a second, “deeper” compartment. Access of aminoglycosides to the organ of Corti is probably directly from the perilymph, rather than from the endolymph.81 That gentamicin appears in the endolymph at all seems to be a function of it being slowly released from inner ear tissues. This delayed release may explain why aminoglycosides are not immediately toxic when applied into the perilymph, whereas toxicity in vitro (without compartments) is seen immediately.82 Hiel and coworkers83 showed that gentamicin is rapidly and specifically captured by hair cells, such that there is significant uptake of gentamicin before morphologic ototoxic changes occur. Hair cells store aminoglycosides in lysosomes, essentially constituting the “deep” intracellular compartment referred to previously. This compartment provides prolonged exposure of these cells to aminoglycosides, and may be the basis for the delayed morphologic and functional effects seen clinically.
The pharmacokinetic data generated in animal models seem to fit what occurs in humans. Becvarovski and colleagues79 devised an in vivo human pharmacokinetic study by recruiting patients who were undergoing either translabyrinthine surgery or labyrinthectomy and exposing them to gentamicin intraoperatively through the facial recess. In these patients, there was rapid diffusion of gentamicin into the perilymph that peaked after 30 minutes. After this peak, concentrations were in a stable range until the limit of the experiment at 110 minutes. Although there was no evidence of gentamicin in the cerebrospinal fluid, gentamicin was detected in serum shortly after administration (1 to 2 hours). This study confirms that rapid diffusion of gentamicin across the RWM occurs in humans. This study indicates that the elimination of gentamicin involves crossing the blood-labyrinthine barrier because gentamicin was detected in the serum shortly after administration.
As mentioned previously, progress in understanding gentamicin pharmacokinetics has been made with computer simulations. Plontke and colleagues15 approximated published in vivo kinetics data by adjusting input parameters defining RWM permeability, clearance, and interscala drug exchange. The results are shown in Figure 155-8. They were able to establish that intratympanically administered gentamicin spreads from the RWM to the vestibule by communication through the scala, rather than by diffusion through the helicotrema. The study also suggested that drug concentrations and distribution in the perilymph were substantially influenced by the delivery method and the duration of exposure of the drug to the RWM.
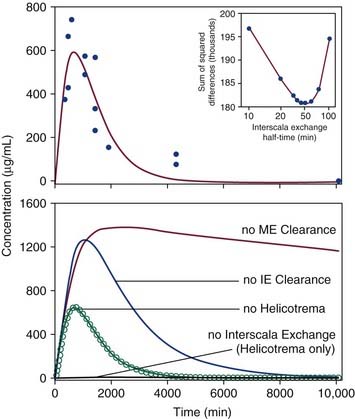
Figure 155-8. Top, Experimental measurements of gentamicin levels in perilymph samples taken from the vestibule after application to the round window (solid circles). Data are replotted from Hoffer and colleagues and Balough.23,80,169 Solid line shows best fit of the concentration time course established by the simulator (the Washington University Cochlear Fluids Simulator, version 1.6) based on physical processes of solute movement. The curve represents the calculated concentration of a simulated 15-µL sample taken from the vestibule (as described in the text). Inset graph, A unique best fit (using methods described in the text) was found to occur with an interscala exchange half-time of 45 minutes. Bottom, Calculated gentamicin time course in the vestibule with best-fit parameters (open circles) and with specific processes in the simulator disabled (solid lines).
(From Plontke SKR, Wood AW, Salt AN. Analysis of gentamicin kinetics in fluids of the inner ear with round window administration. Otol Neurotol. 2002;23:967.)
Clinical Protocols
Although many studies that used intratympanic gentamicin have been published, because of extreme differences in the quality of data, comparison of the different intratympanic gentamicin protocols is exceedingly difficult. Instead, several studies representing various strategies are discussed, and their results are summarized. There are three basic strategies used in intratympanic gentamicin therapy: fixed protocols, titrated protocols, and sustained-release protocols. Fixed protocols use a specific dose and number of injections in all patients. Titration protocols adjust the total dose delivered to a predetermined end point, such as paralytic nystagmus, decreased tandem gait, subjective dysequilibrium, or, more simply (and more commonly), relief from vertigo.76 Sustained-release protocols work on various principles, depending on the delivery mechanism.
There is little difference between fixed and titration protocols because most fixed protocols can be repeated if no clinical response is seen, and most titration protocols deliver a fixed number of injections in the beginning of the protocol, and then repeat them as necessary. The supposed advantage of fixed protocols is that each patient receives the same treatment, making outcomes easier to interpret across individuals. Fixed protocols can be more cost-effective because excessive testing and clinical visits are avoided.61 The flexible structure of titrated protocols supposedly allows for more control of the ototoxic effects of gentamicin, decreasing the risk of hearing loss.84 Close monitoring for ototoxic symptoms does not confer the ability to reverse these symptoms, however.85
Much of the controversy in intratympanic gentamicin therapy in the 1990s concerned a form of fixed protocol known as a “shotgun” protocol. The classic “shotgun” protocol was popularized by Nedzelski and colleagues in 1992.86 There have been periodic reports on this original “shotgun” protocol, and it is now one of the largest series in the literature, spanning a decade and including more than 90 patients. The protocol calls for three daily injections for 4 consecutive days of 26.7 mg/mL of gentamicin, and results in a vertigo control rate of 94% and hearing loss rate of 26%.60,61,86 The main argument against “shotgun” protocols is that they lead to higher rates of hearing loss,87 and that the hearing loss realized is profound.69 Toth and Parnes87 followed the classic “shotgun” protocol outlined previously, but encountered an unacceptable rate of hearing loss (57%) following 18 patients. After they changed to a titration protocol that involved weekly injections, the hearing loss rate decreased to 19%. Altlas and Parnes69 pointed out that in “shotgun” protocols, the proportion of patients who experience hearing loss greater than 30 dB (10% to 24%) is much higher than the proportion seen in titration protocols (0% to 4%).
More recently, “hybrid” protocols have been introduced. Quaranta and associates88 used a fixed schedule of two 0.5-mL intratympanic injections of 20 mg/mL of gentamicin solution spaced 1 week apart. Patients who did not achieve vertigo control after the first two doses were offered additional doses to a total of four doses. This was a prospective study that included a control group of patients with Meniere’s disease who refused treatment. At the 2-year follow-up point, the vertigo control rate was 93% versus 47% for the untreated group. Hearing loss was 7% for the treated group versus 47% for the untreated group. Tinnitus rate and aural pressure relief were 20% and 40% for the treated group versus 27% and 27% for the untreated group. In the protocol, 47% of patients had residual vertigo after two doses and went on to receive a third dose, and 27% went on to receive a fourth dose. This study illustrates the paradoxic effect that intratympanic gentamicin actually improves hearing outcomes of patients with Meniere’s disease. Improvement in hearing from the baseline decline encountered in untreated Meniere’s disease is not a logical outcome if the mechanism of action of intratympanic gentamicin is merely the selective ablation of vestibular hair cells.
The microcatheter delivery method has been recommended to provide sustained gentamicin levels in the perilymph. Schoendorf and associates89 used a high-precision insulin pump and a microcatheter to deliver 40 mg/day of gentamicin to the RWM. The treatment was continued at this fixed rate until vestibular symptoms appeared (in essence, a titration protocol). Although vertigo control was achieved in most patients, 8 of 11 patients treated became deaf. The authors claim that the occurrence of deafness was not correlated with dose because one deafened patient received 720 mg and another received only 60 mg, and hearing was unchanged in a patient who received 240 mg. The authors noted that continuous perfusion protocols should not use titration strategies because the adequate dose for vertigo control may be quickly exceeded. This study highlights the notion that kinetics can have a larger impact on morphologic and functional outcomes than dose-related effects.
In contrast to the preceding study, most continuous-delivery protocols use microdose, slow-infusion strategies. Seidman and colleagues90 reported retrospective data from a multi-institutional trial that compared results of different microcatheter infusion rates. The vertigo control rate was 90% in the group receiving infusions of 1 µL/hr versus 79% in the group receiving infusions of greater than 10 µL/hr, a nonsignificant difference. The hearing loss rate was 23% in the group receiving 1 µL/hr versus 50% in the group receiving greater than 10 µL/hr, however, and this difference was statistically significant. The authors recommended the slow infusion rate and suggested that infusion rates less than 1 µL/hr might have even lower hearing loss rates, while maintaining equal clinical efficacy.
In a study that epitomizes the microdose, slow perfusion rate strategy, Hoffer and colleagues91 reported the 2-year results of 27 patients who underwent microcatheter gentamicin therapy. The study used a pediatric solution of 10 mg/mL, with the pump set at 1 µL/hr for 10 days, resulting in total dose of 2.4 mg. This strategy achieved vertigo control in 93% of patients, hearing loss in only 4%, tinnitus relief in 63%, and elimination of aural pressure in 74%. The protocol included a wide variety of vestibular tests, including vestibulo-ocular gain, phase, and symmetry; posturography score; and step velocity testing. Ninety-six percent of patients had no decrease in vestibular function; however, caloric testing was not performed. The investigators found that vestibular function was improved in 79% of patients.71 These results represent some of the most impressive results of intratympanic gentamicin therapy.
Chia and coworkers92 conducted a meta-analysis of intratympanic gentamicin trials. The study included seven studies (N = 218) that used multiple daily dosing technique (delivery three times per day for ≥4 days), two studies (N = 84) that used weekly dosing technique (weekly injections for four total doses), eight studies (N = 253) that used the low-dose technique (one to two injections with re-treatment for recurrent vertigo), four studies (N = 156) that used continuous microcatheter delivery, and six studies (N = 269) that used the titration technique (daily or weekly doses until onset of vestibular symptoms, change in vertigo, or hearing loss). The titration method of gentamicin delivery showed significantly better complete (81.7%; P = .001) and effective (96.3%; P < .05) vertigo control compared with other methods. The low-dose method of delivery showed significantly worse complete vertigo control (66.7%; P < .001) and trended toward worse effective vertigo control (86.8%; P = .05) compared with other methods. The weekly method of delivery trended toward less overall hearing loss (13.1%; P = .08), and the multiple daily method showed significantly more overall hearing loss (34.7%; P < .01) compared with other groups. No significant difference in profound hearing loss was found between groups, and the degree of vestibular ablation after gentamicin therapy was not significantly correlated with the resulting vertigo control or hearing loss status.
Practical Considerations
The argument for the use of intratympanic injection is that it is the simplest, most cost-effective, and least invasive method of delivery, and from published studies, it provides comparative vertigo control and hearing preservation rates to sustained-release protocols. Blakely93,94 argued that even though there are no head-to-head comparisons of these methods, the published results suggest that there is no reason to use anything but the simplest administration protocols. The argument for using sustained-release mechanisms, particularly continuous infusion devices, is that they seem to produce lower rates of hearing loss, allow for standardization across individuals, lead to more predictable and uniform results, and improve vestibular and auditory functioning.71
Dose
Eklund and coworkers95 found that frequency of deafness was dose-dependent in their intratympanic injection study. There is no minimum safe dose, however, because the lowest documented intratympanic gentamicin dose to produce hearing loss is 0.24 mg.96 The method of delivery is as important as dose in terms of ototoxicity; this was illustrated earlier in the trial by Schoendorf and colleagues,89 in which a microcatheter delivered a severely ototoxic dose of gentamicin, a dose that would be considered to be a “low dose” in intratympanic injection protocols. Per the pharmacokinetic studies of Hoffer and colleagues22 mentioned previously, functional and morphologic end organ damage depends less on the total dose delivered to the inner ear than on the kinetics of how the drug reaches inner ear tissues.
Concentration
The concentration of gentamicin used varies among different studies. Low-dose protocols use the pediatric formulation of gentamicin, which comes in a preparation of 10 mg/mL.96 The adult preparation of 40 mg/mL is often diluted to 30 mg/mL. Abou-Halawa and Poe76 compared outcomes between intratympanic gentamicin preparations of 30 mg/mL and 40 mg/mL, and found that although both cohorts had similar vertigo control and hearing loss rates, the cohort receiving 40 mg/mL required fewer injections to achieve these results. Several other studies have confirmed that with low-dose protocols, more periodic treatments are needed, and there is an increase in the recurrence rate.71
Interval
The injection interval depends on the end point chosen for the study. There is increasing support for spacing the injections over at least a week, primarily because of the delayed onset of gentamicin toxicity. The typical deafferentation syndrome occurs only 3 to 5 days after injection.88,97 Other advocates say that if a patient has Meniere’s disease with episodes that are only every other week, it is unreasonable to check for results on a weekly basis.98 In Blakely’s review of intratympanic gentamicin protocols,93,94 he argued that differences in injection intervals have little impact, if any, on outcomes.
Number of Injections
The general belief in the literature is that the fewer intratympanic gentamicin injections, the better. Youssef and Poe70 found that the chances of gentamicin being effective after three injections are significantly reduced. Other reasons to limit the number of injections include an increased risk of permanent perforation of the tympanic membrane with each injection (unless tympanostomy tubes are placed), the increased cost and inconvenience to the patient and the physician, and an increase in the risk of hearing loss (although this is not supported by all studies).87
Complications
The most notorious complication of intratympanic gentamicin treatment is hearing loss. Risk factors for hearing loss have been inconsistently identified across studies; this is partially due to the substantial differences in protocols between studies. Kaplan and associates60 found that the only factor related to hearing loss was poor hearing before treatment. Although some series have reported that the rate of hearing loss increases after multiple daily injections,87 others have found no correlation between the number of injections and hearing loss.70,72,95 There may be a genetic disposition to hearing loss after aminoglycoside treatment because of a maternally inherited 1555 A-to-G nucleotide substitution in the 12s mitochondrial ribosomal RNA.99 Several patients who experienced hearing loss secondary to intratympanic gentamicin have been tested for this substitution, and, to date, not one has been positive for this substitution.60,93,94,100
One issue that has been raised in assessing hearing loss across studies is the extent of the hearing loss. Most studies document hearing loss as being greater than 10-dB pure-tone average loss or greater than 15% decrease in speech discrimination scores (1995 AAO-HNS guidelines44). This may be a poor reporting format, however, because it does not indicate whether hearing loss is serviceable or profound. As noted previously, Altlas and Parnes69 argued that “shotgun” protocols result in substantial rates of hearing loss greater than 30 dB (range 10% to 24%84,86,97,101), whereas titration methods report low rates of substantial hearing loss (range 0% to 3.5%69,96,98,102). Although scientifically valid comparisons of these studies are impossible because of substantial difference in protocols, this trend is a troubling feature of “shotgun” protocols.
Reporting of hearing loss n gentamicin trials does not distinguish whether the deficit is due to drug-induced toxicity or the natural consequence of Meniere’s disease. The long-term hearing outcome for untreated Meniere’s disease has been reported to be approximately 30% with medical therapy.68 This figure is close to the hearing loss rates reported by most intratympanic gentamicin trials. The implication is that any intratympanic gentamicin trial that reports rates significantly better than 30% hearing loss rate of untreated patients may represent an actual prevention of hearing loss.
Determination of when hearing loss occurs in the course of therapy may be just as important as if hearing loss occurs. Several studies reported that if severe hearing loss occurs, it is noticed within 24 hours of gentamicin administration.96 Kaplan and associates60 reported updated data from patients who underwent the classic “shotgun” protocol, including statistics on the hearing loss rates at 1 month and 2 years. Of the 22 patients with worse hearing at 2 years, 80% realized this loss in the first month after treatment. Similarly, 91% of patients whose hearing was unchanged at the 1-month checkup had unchanged hearing at the 2-year checkup. Of the patients who experienced early hearing loss, 14 of 17 had profound hearing loss (defined as >50 dB or <50% word discrimination score), whereas of the patients who experienced late hearing loss, 4 of 5 had nonprofound hearing loss. These findings imply that the 1995 AAO-HNS guidelines44 might be improved if they asked clinicians to report hearing results immediately after treatment and at the 2-year interval. This information also supports efforts to use otoprotective agents to salvage hearing after toxic gentamicin treatment. Finally, although no formal data have been published to date, Jackson and Silverstein19 described initial efforts to use steroids as a salvage treatment for hearing loss secondary to ototoxic side effects of gentamicin treatment in patients with Meniere’s disease.
Acute vestibular deafferentation syndrome should be an anticipated outcome of treatment, and in this sense it is not strictly a complication. Acute vestibular deafferentation syndrome, also known as acute chemical labyrinthine upset, is the consequence of unilaterally insulting the vestibular apparatus.103 This phenomenon usually occurs 3 to 5 days after the injection. Symptoms include vertigo, nausea, oscillopsia, and dysequilibrium. Patients can readily distinguish these symptoms from symptoms typically related to Meniere’s disease. These symptoms become progressively worse until they peak 1 week after onset. During this peak, patients usually require 2 to 3 days of bed rest. Gradual resolution is achieved in 2 to 4 weeks in most patients.88,97 Because acute vestibular deafferentation syndrome is an expected outcome of therapy, some authors recommend that a vestibular rehabilitation team be available to work with severely affected patients.104
Other vestibular-related side effects include constant unsteadiness and dizziness, insecure gait in dark rooms, instability of the field of vision when turning the head, and very short episodes of losing control over balance or gait (or so-called Tumarkin attacks, which characterize the symptoms of some patients with Meniere’s disease). Tumarkin attacks may not depend on the vestibular function because these attacks have been reported in patients with no caloric response after treatment.89 Visual vestibular mismatch is currently being investigated as a possible side effect of gentamicin therapy. Visual vestibular mismatch is a syndrome in which visual and vestibular information is poorly coordinated in the brain, resulting in complaints of malaise and “dizziness.” Classic complaints include feeling uneasy, nauseous, or unsteady in supermarket aisles or in shopping malls, new-onset motion sickness, a dislike of fluorescent lights, or an aversion to elevator and escalator rides. This syndrome is believed to result from an overreliance on visual signals. Longridge and colleagues105 found that 17 of 28 patients with Meniere’s disease who had previous gentamicin therapy had visual vestibular mismatch. Currently, visual vestibular mismatch is a clinical diagnosis, does not correlate with posturography or caloric tests, and is a relatively new diagnostic category; conclusions about visual vestibular mismatch and gentamicin therapy should be interpreted with caution.
Indications and Contraindications
Intratympanic gentamicin treatment is indicated in patients with Meniere’s disease who have had conservative therapy fail and have recurrent vertigo. Conservative therapy consists of a diuretic and diet restrictions (salt, caffeine), and is effective in 70% of cases. Some patients try additional medical treatments, including vasodilators, vestibular suppressants, tranquilizers, calcium antagonists, betahistine, antidepressants, histamine, and steroids.72 Most practitioners consider intratympanic gentamicin therapy only after conservative therapy has failed after being tried for at least 1 year. At this junction, intratympanic steroids, endolymphatic sac surgery, and intratympanic gentamicin therapy are considered.
Sennaroglu and coworkers42 argued that it is reasonable to try to use intratympanic steroids before using intratympanic gentamicin. In a prospective study that compared intratympanic dexamethasone (n = 24) and gentamicin (n = 16), they found that vertigo control rates were 72% and 75%. Intratympanic gentamicin was considerably more cochleotoxic than intratympanic steroids, however. Vestibular nerve section and labyrinthectomy are reserved for only the most severe and refractory cases with and without residual hearing.
Some practitioners recommend that elderly patients should be treated with caution because central compensation for peripheral vestibular loss is less effective in elderly patients.104 Bilateral Meniere’s disease (incidence of 15% to 30%) is considered by some practitioners to be a contraindication for unilateral intratympanic gentamicin treatment because if hearing loss results from treatment the remaining ear may be vulnerable to hearing loss as a natural course of the disease.106 If bilateral disease is treated, some authors advocate that an intramuscular streptomycin protocol, rather than an intratympanic protocol, be used.107 Patients with no serviceable hearing in the problematic ear are generally considered candidates for labyrinthectomy, but some authors recommend that the low-morbidity, low-cost intratympanic gentamicin protocol should be attempted in these patients before surgery is considered.108
There is some debate as to whether otologic surgery is a contraindication to intratympanic gentamicin treatment. Minor68 reported that of patients with prior otologic surgery, only one of six achieved vertigo control. Eklund and colleagues95 also noted that patients with prior endolymphatic sac surgery had poorer hearing outcomes. Other authors have found that prior otologic surgery does not affect either vertigo or hearing outcomes.24 The importance of this debate is that several practitioners advocate endolymphatic shunt procedures before resorting to intratympanic gentamicin when treating intractable vertigo in patients with Meniere’s disease.68
Otoprotective Agents
Several otologic conditions may benefit from protective or preventive treatment, including noise-induced hearing loss, exposure to ototoxic medications, and salvage of spiral ganglion neurons (SGNs) for the purposes of maximizing the effectiveness of cochlear prostheses. Otoprotective agents can be categorized as either primary or secondary interventions to the overall pathophysiologic process. Primary interventions act directly at the site of insult, whereas secondary interventions act on the intracellular sequelae of primary insults (i.e., apoptosis). Examples of primary interventions include MK801, an N-methyl-D-aspartate receptor antagonist, which has shown protection against acoustic trauma and aminoglycoside toxicity,109,110 and methionine for gentamicin and cisplatin toxicity.111,112 Secondary interventions include steroids,28 apoptosis inhibitors,95 and neurotrophins.113
There is a sort of “intermediate intervention” that is possible with noise-induced hearing loss and the use of antioxidants. The contribution of free radical damage in noise-induced hearing loss results from metabolic and oxidative stress, ionic flux and dysregulation of calcium homeostasis, and mitochondrial injury. A more recent example of antioxidant treatment for noise-induced hearing loss is the use of N-acetyl cysteine.114 We focus here mainly on neurotrophins as otoprotective agents because these are most cogent to the emerging pharmacology of the cochlea and labyrinth.
Apoptosis has been shown to be the major pathway of cell death in various conditions, including aminoglycoside toxicity,53 cisplatin toxicity,115 hypoxia,116 acoustic trauma,77 neurotrophin withdrawal,113,115 and presbycusis.117 Neurotrophic support may be crucial in preventing apoptosis. Malgrange and associates113 prevented apoptosis in explanted outer hair cells by administering various neurotrophins. In the same experiment, these investigators prevented apoptosis by administering protein synthesis inhibitors. These observations imply that neurotrophic support (among other things) provides tonic suppression of proteins in the apoptotic cascade. Rong and colleagues118 provided proof of this notion by showing that nerve growth factor (NGF) promotes cell survival by regulating proteins that are essential to the apoptotic cascade.
Free radicals also play an important role in hearing loss. They are primary inducers of apoptosis (as in free radical–generating aminoglycoside-iron compounds) and secondary consequences of cochlear insult (as in the oxidative stress of excitotoxicity). The generation of free radicals may be a required step in apoptosis. There is a strong connection between free radicals and neurotrophins. Dugan and coworkers119 showed that removal of NGF from cultured auditory neurons caused a rapid increase in intracellular free radical generation and subsequent apoptosis. Reapplying NGF rapidly diminished the concentration of these free radicals and decreased apoptotic cell death. Shulz and coworkers4 showed that inhibition of caspases (proteases associated with apoptosis) not only blocked apoptosis, but also blocked the formation of free radicals, implying that the formation of free radicals is downstream from caspases in the apoptotic cascade. Likewise, exogenous brain-derived neurotrophic factor (BDNF) not only protects cisplatin-exposed neuronal cultures from apoptosis, but also reduces the free radical species in the culture.120 These results have led researchers to believe that free radicals are effector molecules in the apoptotic cascade, and that they can be regulated not only by antioxidants, but also by neurotrophins.
Neurotrophins modulate calcium homeostasis,94 regulate apoptotic proteins,118 and alter expression of endogenous free radical scavengers,4,119,120 and any of these functions may account for their otoprotective effects. Several specific neurotrophins have been used for in vivo models of noise-induced hearing loss with variable success. Glial cell line–derived neurotrophic factor (GDNF) greatly enhances the survival of guinea pig cochlear neurons (but not hair cells) if administered 4 days after noise-induced trauma.121 Keithley and associates122 showed that hair cells are preserved if GDNF is administered before or immediately after toxic noise exposure. Damage was attenuated if GDNF was applied after 2 hours, but not after 4 hours. The method of delivery in this experiment was topically to the RWM.
Shoji and coworkers123 found that GDNF provides bilateral protection even when administered in only one ear. Because their experiment used long-term application of GDNF, it may have diffused through the cerebrospinal fluid. Another possibility is that GDNF influences higher cortical centers in the auditory pathway, which has a rich efferent projection and is highly bilateral. Yamasoba and colleagues124 combined GDNF with antioxidants to determine whether synergistic protection could be achieved. They found that additional functional, but not morphologic, protection was achieved, implying that the interventions in antioxidant and neurotrophin therapy are additive, not synergistic. Neurotrophin-3 (NT-3) showed equal or greater physiologic and histologic protection from acoustic trauma relative to GDNF (with the same animal model).125 BDNF and fibroblastic growth factor show no protective effect against toxic noise.125
Many experimental otoprotective treatments have been shown to be effective only if administered prophylactically or immediately after injury. Many of these injuries clinically manifest long after the initial insult, precluding any hope of salvaging hair cells. There is a much larger window of opportunity to salvage the auditory neurons after injury, however. Although there is rapid degeneration of the peripheral processes of the auditory nerve after inner hair cell loss (within hours or days), the degeneration of SGN soma and central auditory projections takes much longer (weeks to years).126 There is intense interest in the use of neurotrophic agents to enhance SGN survival, mostly in anticipation of future cochlear implantation. This is because the hearing benefits of cochlear implants are directly related to the number of surviving auditory neurons127 and their proximity to stimulating electrodes.126,128
Neurotrophins are essential not only for the normal development of the central and peripheral nervous systems, but also for their maintenance and normal function.126 The destruction of cochlear hair cells and supporting cells because of exogenous insults (e.g., ototoxicity, acoustic trauma) results in the de facto withdrawal of trophic support. The ensuing degeneration of SGNs after cochlear insult is most likely a result of the loss of cochlear trophic support, rather than from direct toxic insult.126 Withdrawal of neurotrophins in vitro causes apoptosis in SGNs.116 The application of antisense oligonucleotides to inhibit expression of BDNF and NT-3 induces programmed cell death in explanted auditory neurons.115 Apoptosis inhibitors have been shown to block neurotrophin withdrawal–induced cell death.116
Numerous neurotrophins have been shown to be effective in preventing SGN demise after experimental destruction by various insults. Exogenously applied NT-3,129,130 BDNF,130–132 BDNF in combination with a ciliary neurotrophic factor analogue,133 NGF,134 and 4-methylcatechol (an inducer of NGF synthesis)135 enhance SGN survival after aminoglycoside insult. BDNF and GDNF enhance SGN survival after acoustic trauma121; BDNF136 and GDNF137 enhance SGN survival after cisplatin exposure. The enhancement of SGN survival with electric stimulation may also involve neurotrophins because depolarization induces an autocrine neurotrophin loop.138 A strategy to use neurotrophins to enhance SGN survival before implantation and electric stimulation to promote autocrine neurotrophin release after implantation may be effective in enhancing hearing outcomes in patients receiving cochlear implants.
Aside from neuronal salvage, numerous studies have documented the ability of neurotrophins to stimulate innate regenerative and repair capabilities in auditory cells. Enfors and colleagues129 showed that NT-3 induced neurofilament growth after aminoglycoside ototoxicity, as evidenced by the appearance of Gap 43, a growth cone marker. Lefebvre and colleagues139 showed that NGF is a potent stimulator of repair and reinnervation in auditory neurons. Ylikoski and coworkers121 showed that GDNF has potent trophic effects on the cochlear-neuron perikarya. Fibroblastic growth factor alone140 or in combination with BDNF141 produces a dose-dependent increase in the number and length of SGNs. These and other similar findings are significant because closer contact between stimulating electrodes on cochlear implants and auditory neurons leads to reduced stimulation thresholds, increased dynamic range of responsiveness, and potentially enhanced specificity of excitation.126,128 It is possible that these growth-stimulating properties of neurotrophins could be exploited to engineer intimate contact with implanted electrodes, such that increasingly focused tonotopic stimulation could be matched to advanced signal-processing strategies.126
Preliminary studies on the interactions between auditory neurons and alloplastic cochlear implant materials have been performed, paving the way for the development of implant-tissue interfaces for the next generation of cochlear prostheses.142 Neurotrophins are expected to play a role in this effort to unite biologic and synthetic materials, an effort that may lead to the development of a fully functional artificial cochlea. Neurotrophins have also been tested as a secondary intervention after aminoglycoside toxicity. Ruan and colleagues143 used long-term infusion of NT-3 and BDNF in the setting of aminoglycoside challenge, and found that NT-3, and to a much lesser extent BDNF, protected hair cells from aminoglycoside toxicity. Although the mechanism of action is unclear, the authors speculate that just as SGNs rely on trophic support from hair cells, these hair cells may have trophic dependence on SGNs. This notion is supported by an increasing body of evidence showing that there is anterograde transport, transcytosis, and recycling of neurotrophic factors in neural networks.144 Hair cell survival is partly determined by neurotrophin support. Malgrange and colleagues113 tested a panel of 13 different growth factors and found that acidic fibroblastic growth factor, insulin-like growth factor-1, epidermal growth factor, transforming growth factor-β1, and GDNF were able to sustain adult explanted outer hair cells (which otherwise experience rapid demise in in vitro culture media).
Gene Therapy
Otologic science was revolutionized by the observation that avian hair cells regenerate after insult.145,146 Since then, barriers to inducing regeneration within the mammalian cochlea have been elucidated, including strategies that can address mature and damaged inner ear.147 Beyond hair cell regeneration, there is the related problem of inducing synaptogenesis and nerve regrowth to these newly created hair cells.148 These and a host of other concerns are currently being investigated. The ultimate vision is to orchestrate through pharmacology and gene therapy a recapitulation of the embryonic events that lead to a functioning auditory system. Although this section deals mainly with gene therapy, there are exciting new stem cell therapies that address similar topics on the horizon as well.149
Vectors
There is no single ideal vector for gene transfer because each vector has characteristics that make it more or less suitable to each application (Table 155-1). The length of the gene to be transferred, the specific inner ear tissue that is targeted, the desired method of delivery, and the duration of transgene expression are all factors that determine the most suitable vectors. Various viral and nonviral vectors are available to the gene therapist. The variability in the transgene expression pattern with different gene transfer vectors is likely a consequence of numerous factors, including the size of the viral particle, presence or absence of viral receptors, and mode of delivery. Inspection of Table 155-1 allows for some generalization about the ability of various viral vectors in transfecting cochlear tissues. The spiral ganglion cells, spiral ligament, and Reissner’s membrane were transfected by every virus tested. Only adenovirus showed transgene expression within the stria vascularis. Immune response was present in the cochlea after transfection with adenovirus, herpes simplex virus, and vaccinia virus.
Delivery
Local gene therapy to the inner ear is feasible because of its relatively closed anatomy. Developing a delivery method for genetic vectors to the inner ear without causing local destruction is a significant obstacle. Several delivery methods have been used, including mini–osmotic pump infusion (either through cochleostomy or through the RWM), microinjection into the RWM, injection into the endolymphatic sac, and diffusion across the RWM after local Gelfoam placement. Cochleostomy has been shown to cause histopathologic alterations (including localized surgical trauma and inflammation), and may lead to hearing loss.150
Introduction of viral vector by means of infusion with a mini–osmotic pump was characterized by evidence of trauma at the basal turn adjacent to the cochleostomy associated with an inflammatory response and connective tissue deposition. Carvalho and Lalwani150 showed preservation of preoperative auditory brainstem response thresholds in the lower frequencies (1 to 2 kHz), mild postoperative elevation of thresholds (<10 dB) in the midfrequencies (4 to 8 kHz), and marked increase (>30 dB) in auditory brainstem response thresholds at higher frequencies (>16 kHz) after mini–osmotic pump infusion by way of a cochleostomy.
Several studies have documented that direct microinjection through the RWM can be accomplished without causing permanent hearing loss or tissue destruction seen with cochleostomy.56,124 Histologically, cochleae microinjected through the RWM showed intact cochlear cytoarchitecture and an absence of inflammatory response 2 weeks after microinjection through the RWM. Microinjection through the RWM did not cause permanent hearing dysfunction.151
The potential for surgical trauma, inflammation, and hearing loss associated with these infusion or microinjection techniques led to the investigation of a less invasive delivery method. Diffusion across the RWM has been shown to be an effective, atraumatic, but vector-dependent method of delivery for gene transfer vectors. Jero and coworkers152 investigated the potential to deliver various vectors across an intact RWM by loading vectors onto a Gelfoam patch that was placed in the round window niche. Adenovirus and liposome vectors, but not the AAV vector, effectively infected inner ear tissues, as evidenced by detection of reporter genes. Lipocomplexed plasmid vectors have also been shown to cross the RWM effectively.153
Preclinical Applications
Staecker and colleagues130 used a herpes simplex virus-1 vector to deliver BDNF to the inner ear. After neomycin injection, the gene therapy group showed a 94.7% salvage rate for SGNs, in contrast to a 64.3% loss of SGNs in control animals. Although BDNF staining was ubiquitous in inner ear tissues, this was not the case for the reporter gene, β-galactosidase. Although only 50% of cells were transfected (as determined by the number of β-galactosidase cells), this was enough to cause cochlea-wide BDNF distribution and to ensure 95% SGN survival. The authors speculate that SGNs must require only a few BDNF-producing cells to ensure the survival of the entire ganglion.
Lalwani and colleagues154 used in vitro and in vivo models to test effectiveness of an AAV vector for BDNF transfer. They found a significant survival effect from controls after aminoglycoside challenge in cochlear explants. Although direct measurements of BDNF could not be recorded, the vector’s ability to salvage SGNs was tested against a gradient of known BDNF concentrations. They found that the vector system was able to achieve the same protective effects as 0.1 ng/mL of BDNF. This dose is subtherapeutic because the most efficient dose was determined to be 50 ng/mL, a concentration of BDNF that results in almost total SGN protection. In the in vivo experiment, animals infused with AAV-BDNF with a mini-osmotic pump displayed enhanced SGN survival. The protection from AAV-BDNF therapy was region specific; there was protection at the basal turn of the cochlea, but not the middle or apical turn. The authors propose that this regional selectivity is a pharmacokinetic phenomenon.
NT-3 gene transfer for protection against cisplatin-induced ototoxicity has been achieved by the use of a herpes simplex virus-1 amplicon–mediated delivery system.155,156 Chen and associates156 established efficacy of the vector in an in vitro study, in which amplicon-mediated transfer of NT-3 (shown by production of NT-3 mRNA proteins and by reporter gene expression) conferred increased survival to cochlear explants after cisplatin exposure. Bowers and colleagues155 confirmed these effects in an in vivo model, in which amplicon-mediated transfer of NT-3 to SGNs suppressed cisplatin-induced apoptosis and necrosis. The authors suggest that these findings not only may be useful to prevent cisplatin-related injury, but also may provide preventive treatment for hearing degeneration because of normal aging.
Several studies have established the efficacy of an Ad vector carrying the GDNF gene (Ad.GDNF) to protect against various ototoxic insults. When administered before aminoglycoside challenge, Ad.-GDNF significantly protects cochlear157 and vestibular158 hair cells from cell death. Pretreatment with Ad.GDNF also provides significant protection against noise-induced trauma.50 Finally, Ad.GDNF enhances SGN survival when administered 4 to 7 days after ototoxic deafening with aminoglycosides.159
Some studies have used gene therapy to induce the expression of target proteins other than neurotrophins. Several studies have transduced genes that express proteins that inhibit the apoptosis cascade, and protect against ototoxic insult. Liu and associates160 used an AAV vector to induce Bcl-x(L), an apoptosis inhibitor to mitigate aminoglycoside toxicity. Chan and colleagues161 successfully induced the expression of the X-linked apoptosis inhibitor using an AAV virus, and this proved to be protective against cisplatin toxicity. In another line of research, attempts are being made to overexpress Math1 through gene transfer. The significance of Math1 gene is that it is intimately associated with cochlear and vestibular stem cells, and the overexpression of the Math1 protein stimulates hair cell regeneration. Izumikawa and colleagues162 used an adenovirus vector to induce overexpression of Math1 after aminoglycoside deafening, and showed cellular and functional repair in the organ of Corti. Similarly, Staecker and coworkers163 used an adenovirus vector to induce expression of Math1 after vestibulotoxic aminoglycoside exposure, and successfully induced the regeneration of vestibular hair cells. These and other studies are adding to the list of potential targets for gene therapy for the inner ear.
Safety Concerns
Early investigations in the development of gene transfer in the cochlea identified potential safety concerns. Through use of AAV as the gene therapy vector, Lalwani and associates164 observed transgene expression within the contralateral cochlea of the AAV-perfused animal, albeit much weaker than within the directly perfused cochlea. Subsequently, Stover and colleagues165 showed transgene expression in the contralateral cochlea using adenovirus. This finding of transgene expression in the contralateral cochlea raises concern about dissemination of the virus from the target tissue. The appearance of the virus, distant from the site of infection, may be due to its hematogenous dissemination to near and distant tissues; however, this is unlikely.165,166 Other possible explanations include migration of AAV by way of the bone marrow space of the temporal bone166 or the cerebrospinal fluid space to the contralateral ear.165,166 The perilymphatic space into which the virus is perfused is directly connected to the cerebrospinal fluid by way of the cochlear aqueduct; transgene expression within the contralateral cochlear aqueduct has been shown after introduction of the viral vector in the ipsilateral cochlea. Collectively, these results suggest potential routes for AAV dissemination from the infused cochlea through the cochlear aqueduct, or by extension through the temporal bone marrow spaces. Subsequent investigations have shown that dissemination outside the target cochlea can largely be eliminated by the use of microinjection or RWM application of vector, and avoidance of the infusion technique.152,167,168
Chandrasekhar SS. Intratympanic dexamethasone for sudden sensorineural hearing loss: clinical and laboratory evaluation. Otol Neurotol. 2001;22:18.
Chandrasekhar SS, Rubinstein RY, Kwartler JA, et al. Dexamethasone pharmacokinetics in the inner ear: comparison of route of administration and use of facilitating agents. Otolaryngol Head Neck Surg. 2000;122:521.
Chen JM, Kakigi A, Harakawa H, et al. Middle ear instillation of gentamicin and streptomycin in chinchillas: morphological appraisal of selective ototoxicity. J Otolaryngol. 1999;28:121.
Chia SH, Gamst AC, Anderson JP, et al. Intratympanic gentamicin therapy for Ménière’s disease: a meta-analysis. Otol Neurotol. 2004;25:544-552.
Fitzgerald DC, McGuire JF. Intratympanic steroids for idiopathic sudden sensorineural hearing loss. Ann Otol Rhinol Laryngol. 2007;116:253-256.
Garduño-Anaya MA, Couthino De Toledo H, Hinojosa-González R, et al. Dexamethasone inner ear perfusion by intratympanic injection in unilateral Ménière’s disease: a two-year prospective, placebo-controlled, double-blind, randomized trial. Otolaryngol Head Neck Surg. 2005;133:285-294.
Goycoolea MV, Lundman L. Round window membrane: structure function and permeability: a review. Microsc Res Tech. 1997;36:201.
Hoffer ME, Allen K, Kopke RD, et al. Transtympanic versus sustained-release administration of gentamicin: kinetics, morphology, and function. Laryngoscope. 2001;111:1343.
Hoffer ME, Kopke RD, Weisskopf P, et al. Microdose gentamicin administration via the round window microcatheter. Ann N Y Acad Sci. 2001;942:46-51.
Izumikawa M, Minoda R, Kawamoto K, et al. Auditory hair cell replacement and hearing improvement by Atoh1 gene therapy in deaf mammals. Nat Med. 2005;11:271-276.
Kopke RD, Hoffer ME, Wester D, et al. Targeted topical steroid therapy in sudden sensorineural hearing loss. Otol Neurotol. 2001;22:475.
Lalwani AK, Jero J, Mhatre AN. Current issues in cochlear gene transfer. Audiol Neurotol. 2002;7:146.
Malgrange B, Rigo JM, Coucke P, et al. Identification of factors that maintain mammalian outer hair cells in adult organ of Corti explants. Hear Res. 2002;170:48.
Montandon P, Guillemin P, Hausler R. Prevention of vertigo in Ménière’s syndrome by means of transtympanic ventilation tubes. Otorhinolaryngology. 1988;50:377.
Parnes LS, Sun AH, Freeman DJ. Corticosteroid pharmacokinetics in the inner ear fluids: an animal study followed by clinical application. Laryngoscope. 1999;109(Suppl 9):1.
Plontke SKR, Wood AW, Salt AN. Analysis of gentamicin kinetics in fluids of the inner ear with round window administration. Otol Neurotol. 2002;23:967.
Salt AN, Ma Y. Quantification of solute entry into cochlear perilymph through the round window membrane. Hear Res. 2001;154:88.
Schuknect H. Ablation therapy in Ménière’s disease. Acta Otolaryngol (Stockh). 1957;132(Suppl):1.
Sennaroglu L, Sennaroglu G, Gursel B, et al. Intratympanic dexamethasone, intratympanic gentamicin, and endolymphatic sac surgery for intractable vertigo in Ménière’s disease. Otolaryngol Head Neck Surg. 2001;125:537.
Shinohara T, Bredberg G, Ulfendahl M, et al. Neurotrophic factor intervention restores auditory function in deafened animals. Proc Natl Acad Sci U S A. 2002;99:1657.
Shirwany NA, Seidman MD, Tang W. Effects of transtympanic injection of steroids on cochlear blood flow, auditory sensitivity, and histology in the guinea pig. Am J Otol. 1998;19:230.
Silverstein H, Isaacson JE, Olds MJ, et al. Dexamethasone inner ear injection for the treatment of Ménière’s disease: a prospective, randomized, double blind crossover trial. Am J Otol. 1998;19:196.
Silverstein H, Rowan PT, Olds MJ, et al. Inner ear perfusion and the role of round window patency. Am J Otol. 1997;18:586.
Staecker H, Praetorius M, Baker K, et al. Vestibular hair cell regeneration and restoration of balance function induced by math1 gene transfer. Otol Neurotol. 2007;28:223-231.
Wilson WR, Byl FM, Laird N. The efficacy of steroids in the treatment of idiopathic sudden hearing loss: a double blind clinical study. Arch Otolaryngol Head Neck Surg. 1980;106:772.
1. Silverstein H, Isaacson JE, Olds MJ, et al. Dexamethasone inner ear injection for the treatment of Ménière’s disease: a prospective, randomized, double blind crossover trial. Am J Otol. 1998;19:196.
2. Goycoolea MV, Lundman L. Round window membrane: structure function and permeability: a review. Microsc Res Tech. 1997;36:201.
3. Yoon YJ, Hellstrom S. Ultrastructural characteristics of the round window membrane during pneumococcal otitis media in rat. J Korean Med Sci. 2002;17:230.
4. Shulz JB, Bremen D, Reed JC, et al. Cooperative interception of neuronal apoptosis by BCL-2 and BAG-1 expression: prevention of caspase activation and reduced production of reactive oxygen species. J Neurochem. 1997;69:2075.
5. Engel F, Blatz R, Kellner J, et al. Breakdown of the round window membrane permeability barrier evoked by streptolysin O: possible etiologic role in development of sensorineural hearing loss in acute otitis media. Infect Immun. 1995;63:1305.
6. Chandrasekhar SS, Rubinstein RY, Kwartler JA, et al. Dexamethasone pharmacokinetics in the inner ear: comparison of route of administration and use of facilitating agents. Otolaryngol Head Neck Surg. 2000;122:521.
7. Kitamura Y, Teranishi M, Sone M, et al. Round window membrane in young and aged C57BL/6 mice. Hear Res. 2002;174:142.
8. Goycoolea MV. Clinical aspects of round window membrane permeability under normal and pathological conditions. Acta Otolaryngol. 2001;121:437.
9. Goycoolea MV, Munchow D, Schachern PA. Experimental studies on round window membrane structure, function and permeability. Laryngoscope. 1988;98(Suppl 44):1.
10. Juhn SK, Hamguchi Y, Goycoolea M. Review of round window membrane permeability. Acta Otolaryngol Suppl. 1988;457:43.
11. Nordang L, Anniko M. Hearing loss in relation to round window membrane morphology in experimental otitis media. Otorhinolaryngology. 2001;63:333.
12. Sahni RS, Paparella MM, Schachern PA, et al. Thickness of the human round window membrane in different forms of otitis media. Arch Otolaryngol Head Neck Surg. 1987;113:630.
13. Sha SH, Zajic G, Epstein CJ, et al. Overexpression of copper/zinc-superoxide dismutase protects from kanamycin-induced hearing loss. Audiol Neurotol. 2001;6:117.
14. Salt AN, Ma Y. Quantification of solute entry into cochlear perilymph through the round window membrane. Hear Res. 2001;154:88.
15. Plontke SKR, Wood AW, Salt AN. Analysis of gentamicin kinetics in fluids of the inner ear with round window administration. Otol Neurotol. 2002;23:967.
16. Silverstein H, Rowan PT, Olds MJ, et al. Inner ear perfusion and the role of round window patency. Am J Otol. 1997;18:586.
17. Alzamil KS, Linthicum FH. Extraneous round window membranes and plugs: possible effect on intratympanic therapy. Ann Otol Rhinol Laryngol. 2000;109:30.
18. Silverstein H, Durand B, Jackson LE, et al. Use of the malleus handle as a landmark for localizing the round window membrane. Ear Nose Throat J. 2001;80:444.
19. Jackson LE, Silverstein H. Chemical perfusion of the inner ear. Otolaryngol Clin North Am. 2002;35:639.
20. Montandon P, Guillemin P, Hausler R. Prevention of vertigo in Ménière’s syndrome by means of transtympanic ventilation tubes. Otorhinolaryngology. 1988;50:377.
21. Plontke SKR, Plinkert PK, Plinkert B, et al. Transtympanic endoscopy for drug delivery to the inner ear using a new microendoscope. Adv Otorhinolaryngol. 2002;59:149.
22. Hoffer ME, Allen K, Kopke RD, et al. Transtympanic versus sustained-release administration of gentamicin: kinetics, morphology, and function. Laryngoscope. 2001;111:1343.
23. Hoffer ME, Balough B, Henderson J, et al. Use of sustained release vehicles in the treatment of Ménière’s disease. Otolaryngol Clin North Am. 1997;30:1159.
24. Seidman M. Continuous gentamicin therapy using an IntraEAR microcatheter for Ménière’s disease: a retrospective study. Otolaryngol Head Neck Surg. 2002;126:244.
25. Praetorius M, Limberger A, Muller M, et al. A novel microperfusion system for the long-term local supply of drugs to the inner ear: implantation and function in the rat model. Audiol Neurotol. 2001;6:250.
26. Ryan AF, Pak K, Low W, et al. Immunological damage to the inner ear: current and future therapeutic strategies. Adv Otorhinolaryngol. 2002;59:66.
27. Shirwany NA, Seidman MD, Tang W. Effects of transtympanic injection of steroids on cochlear blood flow, auditory sensitivity, and histology in the guinea pig. Am J Otol. 1998;19:230.
28. Lamm K, Arnold W. The effect of prednisolone and non-steroidal anti-inflammatory agents on the normal and noise-damaged guinea pig inner ear. Hear Res. 1998;115:149.
29. Gloddek B, Lamm K, Arnold W. Pharmacological influence on inner ear endothelial cells in relation to the pathogenesis of sensorineural hearing loss. Adv Otorhinolaryngol. 2002;59:75.
30. Curtis LM, ten Cate WJ, Rarey KE. Dynamics of Na,K-ATPase sites in later cochlear wall tissues of the rate. Eur Arch Otorhinolaryngol. 1993;250:265.
31. Lee JH, Marcus DC. Nongenomic effects of corticosteroids on ion transport by stria vascularis. Audiol Neurotol. 2002;7:100-106.
32. Wheling M. Specific, nongenomic actions of steroid hormones. Annu Rev Physiol. 1997;59:365.
33. Parnes LS, Sun AH, Freeman DJ. Corticosteroid pharmacokinetics in the inner ear fluids: an animal study followed by clinical application. Laryngoscope. 1999;109(Suppl 9):1.
34. Tobita T, Senarita M, Hara A, et al. Determination of prednisolone in the cochlear tissue. Hear Res. 2002;165:30.
35. Gianoli GJ, Li JC. Transtympanic steroids for treatment of sudden hearing loss. Otolaryngol Head Neck Surg. 2001;125:142.
36. Wilson WR, Byl FM, Laird N. The efficacy of steroids in the treatment of idiopathic sudden hearing loss: a double blind clinical study. Arch Otolaryngol Head Neck Surg. 1980;106:772.
37. Ariyasu L, Byl FM, Sprague MS, et al. The beneficial effect of methylprednisolone in acute vestibular vertigo. Arch Otolaryngol Head Neck Surg. 1990;116:700.
38. Hughes GB, Freedman MA, Haberkamp TJ, et al. Sudden sensorineural hearing loss. Otolaryngol Clin North Am. 1996;29:393.
39. Ryback LP, Ravi R, Somani SM. Mechanism of protection by diethyldithiocarbamate against cisplatin ototoxicity: antioxicarb system. Fundam Appl Toxicol. 1995;26:293.
40. Shea JJ. The role of dexamethasone or streptomycin perfusion in the treatment of Ménière’s disease. Otolaryngol Clin North Am. 1997;30:1051.
41. Itoh A, Sakata E. Treatment of vestibular disorders. Acta Otolaryngol. 1991;481(Suppl):617.
42. Sennaroglu L, Sennaroglu G, Gursel B, et al. Intratympanic dexamethasone, intratympanic gentamicin, and endolymphatic sac surgery for intractable vertigo in Ménière’s disease. Otolaryngol Head Neck Surg. 2001;125:537.
43. Barrs DM, Keyser JS, Stallworth C, et al. Intratympanic steroid injections for intractable Ménière’s disease. Laryngoscope. 2001;111:2100.
44. Committee on Hearing and Equilibrium. Committee on hearing and equilibrium guidelines for the diagnosis and evaluation of therapy in Ménière’s disease. Otolaryngol Head Neck Surg. 1995;113:181.
45. Garduño-Anaya MA, Couthino De Toledo H, Hinojosa-González R, et al. Dexamethasone inner ear perfusion by intratympanic injection in unilateral Ménière’s disease: a two-year prospective, placebo-controlled, double-blind, randomized trial. Otolaryngol Head Neck Surg. 2005;133:285-294.
46. Kopke RD, Hoffer ME, Wester D, et al. Targeted topical steroid therapy in sudden sensorineural hearing loss. Otol Neurotol. 2001;22:475.
47. Chandrasekhar SS. Intratympanic dexamethasone for sudden sensorineural hearing loss: clinical and laboratory evaluation. Otol Neurotol. 2001;22:18.
48. Fitzgerald DC, McGuire JF. Intratympanic steroids for idiopathic sudden sensorineural hearing loss. Ann Otol Rhinol Laryngol. 2007;116:253-256.
49. Sha SH, Schacht J. Salicylate attenuates gentamicin-induced ototoxicity. Lab Invest. 1999;79:807.
50. Kanzaki S, Kawamoto K, Oh SH, et al. From gene identification to gene therapy. Audiol Neurotol. 2002;7:161.
51. Arriaga MS, Goldman S. Hearing results of intratympanic steroid injection treatment of endolymphatic hydrops. Laryngoscope. 1998;108:1682.
52. Nadel DM. The use of systemic steroids in otolaryngology. Ear Nose Throat J. 1996;75:502.
53. Lang H, Lin C. Apoptosis and hair cell degeneration in the vestibular sensory epithelium of the guinea pig following gentamicin insult. Hear Res. 1997;111:177.
54. Spandow O, Anniko M, Hellstrom S. Hydrocortisone applied to the round window niche causes electrophysiological dysfunction of the inner ear. J Otorhinolaryngol. 1989;51:94.
55. Fowler E. Streptomycin treatment of vertigo. Trans Am Acad Ophthalmol Otolaryngol. 1948;52:239.
56. Schuknecht H. Ablation therapy in Ménière’s disease. Acta Otolaryngol (Stockh). 1957;132(Suppl):1.
57. Beck C, Schmidt CL. 10 years experience with intratympanically applied streptomycin (gentamicin) in the therapy of morbus Ménière. Arch Otolaryngol. 1978;221:149.
58. Chen JM, Kakigi A, Harakawa H, et al. Middle ear instillation of gentamicin and streptomycin in chinchillas: morphological appraisal of selective ototoxicity. J Otolaryngol. 1999;28:121.
59. Wanamaker HH, Slepecky NB, Cefaratti LK, et al. Comparison of vestibular and cochlear ototoxicity from transtympanic streptomycin administration. Am J Otol. 1999;20:457.
60. Kaplan DM, Nedzelski JM, Al-Abidi A, et al. Hearing loss following intratympanic installation of gentamicin for the treatment of unilateral Ménière’s disease. J Otolaryngol. 2002;31:106.
61. Kaplan DM, Nedzelski JM, Chen JM, et al. Intratympanic gentamicin for the treatment of unilateral Ménière’s disease. Laryngoscope. 2000;110:1298.
62. Fujiwara Y. Relationship between ototoxicities and chemical structures of ototoxic drugs. Nippon Jibiinkoka Gakkai Kaiho (J Otolaryngol Jpn). 1993;96:1482.
63. Nakashima T, Teranishi M, Hibi T, et al. Vestibular and cochlear toxicity of aminoglycosides: a review. Acta Otolaryngol. 2000;120:904.
64. Li L, Nevill G, Forge A. Two modes of hair cell loss from the vestibular sensory epithelia of guinea pig inner ear. J Comp Neurol. 1995;355:405.
65. Richardson GP, Forge A, Kros CJ, et al. Myosin VIIA is required for aminoglycoside accumulation in cochlear hair cells. J Neurosci. 1997;17:9506.
66. Lopez I, Honrubia V, Lee SC, et al. Quantification of the process of hair cell loss and recovery in the chinchilla crista ampullaris after gentamicin treatment. Int J Dev Neurosci. 1997;15:447.
67. Carey JP, Hirvonen T, Peng GCY, et al. Changes in the angular vestibulo-ocular reflex after a single dose of intratympanic gentamicin for Ménière’s disease. Ann N Y Acad Sci. 2002;956:581.
68. Minor LB. Intratympanic gentamicin for control of vertigo in Ménière’s disease: vestibular signs that specify completion of therapy. Am J Otol. 1999;20:209.
69. Altlas JT, Parnes LS. Intratympanic gentamicin titration therapy for intractable Ménière’s disease. Am J Otol. 1999;20:357.
70. Youssef TF, Poe DS. Intratympanic gentamicin injection for the treatment of Ménière’s disease. Am J Otol. 1998;19:435.
71. Hoffer ME, Kopke RD, Weisskopf P, et al. Microdose gentamicin administration via the round window microcatheter. Ann N Y Acad Sci. 2001;942:46-51.
72. Hirsch BE, Kamerer DB. Role of chemical labyrinthectomy in the treatment of Ménière’s disease. Otolaryngol Clin North Am. 1997;30:1039.
73. Wangemann P. Comparison of ion transport mechanisms between vestibular dark cells and strial marginal cells. Hear Res. 1995;90:149.
74. Wangemann P. K+ cycling and its regulation in the cochlea and the vestibular labyrinth. Audiol Neurotol. 2002;7:199.
75. Yoshihara T, Kaname H, Ishii T, et al. Effect of gentamicin on vestibular dark cells and melanocytes: an ultrastructural and cytochemical study. ORL J Otorhinolaryngol Relat Spec. 1994;56:24.
76. Abou-Halawa AS, Poe DS. Efficacy of increased gentamicin concentration for intratympanic injection therapy in Ménière’s disease. Otol Neurotol. 2002;23:494.
77. Wang J, Dib M, Lenoir M, et al. Rizuzole rescues cochlear sensory cells from acoustic trauma in the guinea-pig. Neuroscience. 2002;111:635.
78. Hibi T, Suzuki T, Nakashima T. Perilymphatic concentration of gentamicin administered intratympanically in guinea pigs. Acta Otolaryngol. 2001;121:336.
79. Becvarovski Z, Bojrab DI, Michaelides EM, et al. Round window gentamicin absorption: an in vivo human model. Laryngoscope. 2002;112:1610.
80. Balough BJ, Hoffer ME, Wester D, et al. Kinetics of gentamicin uptake in the inner ear of Chinchilla langier after middle-ear administration in a sustained release vehicle. Otolaryngol Head Neck Surg. 1998;119:427.
81. Tran Huy P, Bernard P, Schacht J. Kinetics of gentamicin uptake and release in the rat: comparison of inner ear tissues and fluids with other organs. J Clin Invest. 1986;77:1492.
82. Aran JM, Erre JP, Da Costa DL, et al. Acute and chronic effects of aminoglycosides on cochlear hair cells. Ann N Y Acad Sci. 1999;884:60.
83. Hiel H, Bennani H, Erre JP, et al. Kinetics of gentamicin in cochlear hair cells after chronic treatment. Acta Otolaryngol (Stockh). 1992;112:272.
84. Murofushi T, Halmagyi M, Yavor R. Intratympanic gentamicin in Ménière’s disease: results of therapy. Am J Otol. 1997;18:52.
85. McFeely WJ, Singleton GT, Rodriguez FJ, et al. Intratympanic gentamicin treatment for Ménière’s disease. Otolaryngol Head Neck Surg. 1998;118:589.
86. Nedzelski JM, Schessel DA, Bryce GE, et al. Chemical labyrinthectomy: local application of gentamicin for the treatment of unilateral Ménière’s disease. Am J Otol. 1992;13:18.
87. Toth AA, Parnes LS. Intratympanic gentamicin therapy for Ménière’s disease: preliminary comparison of two regimens. J Otolaryngol. 1995;24:340.
88. Quaranta A, Scaringi A, Aloisi A, et al. Intratympanic therapy for Ménière’s disease: effect of administration of low concentration of gentamicin. Acta Otolaryngol. 2001;121:387.
89. Schoendorf J, Neugebauer P, Michel O. Continuous intratympanic infusion of gentamicin via a microcatheter in Ménière’s disease. Otolaryngol Head Neck Surg. 2001;124:203.
90. Seidman MD, Shivapuja BG, Quirk WS. The protective effects of allopurinol and superoxide dismutase on noise-induced cochlear damage. Otolaryngol Head Neck Surg. 1993;109:1052.
91. Hoffer ME, Kopke RD, Weisskopf P, et al. Use of the round window microcatheter in the treatment of Ménière’s disease. Laryngoscope. 2001;111:2046.
92. Chia SH, Gamst AC, Anderson JP, et al. Intratympanic gentamicin therapy for Ménière’s disease: a meta-analysis. Otol Neurotol. 2004;25:544-552.
93. Blakely BW. Clinical forum: a review of intratympanic therapy. Am J Otol. 1997;18:520.
94. Blakely BW. Update on intratympanic gentamicin for Ménière’s disease. Laryngoscope. 2000;110:236.
95. Eklund S, Pyykko I, Aalto H, et al. effect of intratympanic gentamicin on hearing and tinnitus in Ménière’s disease. Am J Otol. 1999;20:350.
96. Thomsen J, Charabi S, Tos M. Preliminary results of a new delivery system for gentamicin to the inner ear in patients with Ménière’s disease. Eur Arch Otorhinolaryngol. 2000;257:362.
97. Rauch SD, Oas JG. Intratympanic gentamicin for treatment of intractable Ménière’s disease: a preliminary report. Laryngoscope. 1997;107:49.
98. Harner SG, Driscol CL, Facer GW, et al. Long-term follow-up of transtympanic gentamicin for Ménière’s syndrome. Otol Neurotol. 2001;22:210.
99. Chen J, Williamson P, Hutchin T, et al. Topical gentamicin-induced hearing loss: a mitochondrial ribosomal RNA study of genetic susceptibility. Am J Otol. 1996;17:850.
100. Chen JM, Williamson PA, Nedzelski JM, et al. Topical gentamicin-induced hearing loss: a mitochondrial ribosomal RNA study of genetic susceptibility. Am J Otol. 1996;17:850.
101. Corsten M, Marsan J, Schramm D, et al. Treatment of intractable Ménière’s disease with intratympanic gentamicin: review of the University of Ottawa experience. J Otolaryngol. 1997;26:361.
102. Hirsh BE, Kramerer DB. Intratympanic gentamicin therapy for Ménière’s disease. Am J Otol. 1997;18:44.
103. Colletti V. Medical treatment in Ménière’s disease: avoiding vestibular neurectomy and facilitating postoperative compensation. Acta Otolaryngol. 2000;544(Suppl):27.
104. Odkvist LM, Bergenius J, Moller C. When and how to use gentamicin in the treatment of Ménière’s disease. Acta Otolaryngol (Stockh). 1997;526(Suppl):54.
105. Longridge NS, Mallinson AI, Denton A. Visual vestibular mismatch in patients treated with intratympanic gentamicin for Ménière’s disease. J Otolaryngol. 2002;31:5.
106. Grant IL, Welling DB. The treatment of hearing loss in Ménière’s disease. Otolaryngol Clin North Am. 1997;30:1123.
107. Baylan FR, Taibah A, De Donato G, et al. Titration streptomycin therapy in Ménière’s disease: long-term results. Otolaryngol Head Neck Surg. 1998;118:261.
108. Bauer PW, MacDonald B, Cox LC. Intratympanic gentamicin therapy for vertigo in nonserviceable ears. Am J Otolaryngol. 2001;22:111.
109. Chen GD, Kong J, Reinhard K, et al. NMDA receptor blockage protects against permanent noise-induced hearing loss but not its potentiation by carbon monoxide. Hear Res. 2001;154:108.
110. Duan M, Agerman K, Ernfors P, et al. Complementary roles of neurotrophin 3 and a N-methyl-d-aspartate antagonist in the protection of noise and aminoglycoside-induced ototoxicity. Proc Natl Acad Sci U S A. 2000;97:7597.
111. Kopke RD, Coleman JKM, Liu J, et al. Enhancing intrinsic cochlear stress defenses to reduce noise-induced hearing loss. Laryngoscope. 2002;112:1515.
112. Sha SH, Schacht J. Antioxidants attenuate gentamicin-induced free radical formation in vitro and ototoxicity in vivo: d-methionine is a potential protectant. Hear Res. 2000;142:43.
113. Malgrange B, Rigo JM, Coucke P, et al. Identification of factors that maintain mammalian outer hair cells in adult organ of Corti explants. Hear Res. 2002;170:48.
114. Bielefeld EC, Kopke RD, Jackson RL, et al. Noise protection with N-acetyl-l-cysteine (NAC) using a variety of noise exposures, NAC doses, and routes of administration. Acta Otolaryngol. 2007;127:914-919.
115. Lefebvre PP, Melgrange B, Lallemend F, et al. Mechanisms of cell death in the injured auditory system: otoprotective strategies. Audiol Neurotol. 2002;7:165.
116. Cheng AG, Huang T, Stracher A, et al. Calpain inhibitors protect auditory sensory cells from hypoxia and neurotrophin-withdrawal induced apoptosis. Brain Res. 1999;850:234.
117. Alam SA, Oshima T, Suzuki M, et al. The expression of apoptosis-related proteins in the aged cochlea of Mongolian gerbils. Laryngoscope. 2001;111:528.
118. Rong P, Bennie AM, Epa WR, et al. Nerve growth factor determines survival and death of PC12 cells by regulation of the bcl-xbax, and caspase-3 genes. J Neurochem. 1999;72:2294.
119. Dugan LL, Creedon DJ, Johnson EM, et al. Rapid suppression of free radical formation by nerve growth factor involves the mitogen-activated protein kinase pathway. Proc Natl Acad Sci U S A. 1997;94:4086.
120. Gabaizadeh R, Staecker H, Liu W, et al. BDNF protection of auditory neurons from cisplatin involves changes in intracellular levels of both reactive oxygen species and glutathione. Mol Brain Res. 1997;50:71.
121. Ylikoski J, Pirvola U, Virkkala J, et al. Guinea pig auditory neurons are protected by glial cell line-derived growth factor from degeneration after noise trauma. Hear Res. 1998;124:17.
122. Keithley EM, Ma CL, Ryan AF, et al. GDNF protects the cochlea against noise damage. Neuroreport. 1998;9:2183.
123. Shoji F, Yamasoba T, Magal E, et al. Glial cell line-derived neurotrophic factor has a dose-dependent influence on noise-induced hearing loss in guinea pig cochlea. Hear Res. 2000;142:41.
124. Yamasoba T, Schacht J, Shoji F, et al. Attenuation of cochlear damage from noise trauma by an iron chelator, a free radical scavenger and glial cell line-derived neurotrophic factor in vivo. Brain Res. 1999;815:317.
125. Shoji F, Miller AL, Mitchell A, et al. Differential protective effects of neurotrophins in the attenuation of noise-induced hair cell loss. Hear Res. 2000;146:134.
126. Miller JM, Miller AL, Yamagata T, et al. Protection and regrowth of the auditory nerve after deafness: neurotrophins, antioxidants and depolarization are effective in vivo. Audiol Neurotol. 2002;7:175.
127. Gantz BJ, Woodworth GG, Knutson JF, et al. Multivariate predictors of audiological success with multichannel cochlear implants. Ann Otol Rhinol Laryngol. 1993;102:909.
128. Shepherd RK, Hatsushika S, Clark GM. Electrical stimulation of the auditory nerve: the effect of electrode position on neural excitation. Hear Res. 1993;66:108.
129. Enfors P, Duan ML, Elshamy WM, et al. Protection of auditory neurons from aminoglycoside toxicity by neurotrophin-3. Nat Med. 1996;2:463.
130. Staecker H, Kopke R, Malgrange B, et al. NT-3 and/or BDNF therapy prevents loss of auditory neurons following loss of hair cells. Neuroreport. 1996;7:889.
131. Miller JM, Chi DH, O’Keeffe LJ, et al. Neurotrophins can enhance spiral ganglion cell survival after inner hair cell loss. Int J Dev Neurosci. 1997;15:631.
132. Staecker H, Gabaizadeh R, Federoff H, et al. Brain-derived neurotrophic factor gene therapy prevents spiral ganglion degeneration after hair cell loss. Otolaryngol Head Neck Surg. 1998;119:7.
133. Shinohara T, Bredberg G, Ulfendahl M, et al. Neurotrophic factor intervention restores auditory function in deafened animals. Proc Natl Acad Sci U S A. 2002;99:1657.
134. Schindler RA, Gladstone HB, Scott N, et al. Enhanced preservation of the auditory nerve following cochlear perfusion with nerve growth factors. Am J Otol. 1995;16:304.
135. Kimura N, Nishizaki K, Orita Y, et al. 4-Methylcatechol, a potent inducer of nerve growth factor synthesis, protects spiral ganglion neurons from aminoglycoside ototoxicity: preliminary report. Acta Otolaryngol (Stockh). 1999;540(Suppl):12.
136. Gabaizadeh R, Staecker H, Liu W, et al. Protection of both auditory hair cells and auditory neurons from cisplatin induced damage. Acta Otolaryngol (Stockh). 1997;117:232.
137. Kuang K, Hever G, Zajic G, et al. Glial cell line-derived neurotrophic factor: potential for otoprotection. Ann N Y Acad Sci. 1999;884:270.
138. Hansen MR, Zha XM, Bok J, et al. Multiple distinct signal pathways, including an autocrine neurotrophic mechanism, contribute to the survival-promoting effect of depolarization on spiral ganglion neurons in vitro. J Neurosci. 2001;21:2256-2267.
139. Lefebvre PP, Malgrange B, Staecker H, et al. Neurotrophins affect survival and neuritogenesis by adult injured auditory neurons in vitro. Neuroreport. 1994;5:865.
140. Dazert S, Kim D, Luo L, et al. Focal delivery of fibroblast growth factor-1 by transfected cells induces spiral ganglion neurite targeting in vitro. J Cell Physiol. 1998;177:123.
141. Altschuler RA, Cho Y, Ylikoski J, et al. Rescue and regrowth of sensory neurons following deafferentation by neurotrophic factors. Ann N Y Acad Sci. 1999;884:305.
142. Brors D, Aletsee C, Schwager K, et al. Interaction of spiral ganglion neuron processes with alloplastic materials in vitro. Hear Res. 2002;167:110.
143. Ruan RS, Leong SK, Mark I, et al. Effects of BDNF and NT-3 on hari cell survival in guinea pig cochlea damaged by Kanamycin treatment. Neuroreport. 1999;10:2067.
144. von Bartheld CS, Wang X, Butowt R. Anterograde axonal transport, transcytosis, and recycling of neurotrophic factors: the concept of trophic currencies in neural networks. Mol Neurobiol. 2001;24:1.
145. Ryals BM, Rubel EW. Hair cell regeneration after acoustic trauma in adult Coturnix quail. Science. 1988;240:1774-1776.
146. Corwin JT, Cotanche DA. Regeneration of sensory hair cells after acoustic trauma. Science. 1988;240:1772-1774.
147. Breuskin I, Bodson M, Thelen N, et al. Strategies to regenerate hair cells: identification of progenitors and critical genes. Hear Res. 2008;236:1-10.
148. Wei D, Jin Z, Jarlebark L, et al. Survival, synaptogenesis, and regeneration of adult mouse spiral ganglion neurons in vitro. Dev Neurobiol. 2007;67:108-122.
149. Martinez-Monedero R, Yi E, Oshima K, et al. Differentiation of inner ear stem cells to functional sensory neurons. Dev Neurobiol. 2008;68:669-684.
150. Carvalho GJ, Lalwani AK. The effect of cochleostomy and intracochlear infusion on auditory brain stem response threshold in the guinea pig. Am J Otol. 1999;20:87.
151. Kho ST, Pettis RM, Mhatre AN, et al. Cochlear microinjection and its effects upon auditory function in the guinea pig. Eur Arch Otorhinolaryngol. 2000;257:469.
152. Jero J, Mhatre AN, Tseng CJ, et al. Cochlear gene delivery through an intact round window membrane in mouse. Hum Gene Ther. 2001;12:539.
153. Maeda Y, Fukushima K, Kawasaki A, et al. Cochlear expression of a dominant-negative GJB2R75W construct delivered through the round window membrane in mice. Neurosci Res. 2007;58:250-254.
154. Lalwani AK, Jero J, Mhatre AN. Current issues in cochlear gene transfer. Audiol Neurotol. 2002;7:146.
155. Bowers WJ, Chen X, Guo H, et al. Neurotrophin-3 transduction attenuates cisplatin spiral ganglion neuron ototoxicity in the cochlea. Mol Ther. 2002;6:12.
156. Chen X, Frisina RD, Bowers WJ, et al. HSV amplicon-mediated neurotrophin-3 expression protects murine spiral ganglion neurons from cisplatin-induced damage. Mol Ther. 2001;3:958.
157. Yagi M, Magal E, Sheng Z, et al. Hair cell protection from aminoglycoside ototoxicity by adenovirus-mediated overexpression of glial cell line-derived neurotrophic factor. Hum Gene Ther. 1999;10:813.
158. Suzuki M, Yagi M, Brown JN, et al. Effect of transgenic GDNF expression on gentamicin-induced cochlear and vestibular toxicity. Gene Ther. 2000;7:1046.
159. Yagi M, Shah P, Magal E, et al. Spiral ganglion neurons are protected from degeneration by GDNF gene therapy. J Am Rhinol Otolaryngol. 2000;1:315.
160. Liu YH, Ke XM, Qin Y, et al. Adeno-associated virus-mediated Bcl-xL prevents aminoglycoside-induced hearing loss in mice. Chin Med J (Engl). 2007;120:1236-1240.
161. Chan DK, Lieberman DM, Musatov S, et al. Protection against cisplatin-induced ototoxicity by adeno-associated virus-mediated delivery of the X-linked inhibitor of apoptosis protein is not dependent on caspase inhibition. Otol Neurotol. 2007;28:417-425.
162. Izumikawa M, Minoda R, Kawamoto K, et al. Auditory hair cell replacement and hearing improvement by Atoh1 gene therapy in deaf mammals. Nat Med. 2005;11:271-276.
163. Staecker H, Praetorius M, Baker K, et al. Vestibular hair cell regeneration and restoration of balance function induced by math1 gene transfer. Otol Neurotol. 2007;28:223-231.
164. Lalwani AK, Walsh B, Reilly PG, et al. Development of in vivo gene therapy for hearing disorders: introduction of adeno-associated virus into the guinea pig cochlea. Gene Ther. 1996;3:588.
165. Stover T, Yagi M, Raphael Y. Transduction of the contralateral ear after adenovirus-mediated cochlear gene transfer. Gene Ther. 2000;5:377.
166. Kho ST, Pettis RM, Mhatre AN, et al. Safety of adeno-associated virus as cochlear gene transfer vector: analysis of distant spread beyond injected cochleae. Mol Ther. 2000;2:368.
167. Han JJ, Mhatre AN, Wareing M, et al. Transgene expression in the guinea pig cochlea mediated by the lentivirus derived gene transfer vector. Hum Gene Ther. 1999;10:1867.
168. Wareing M, Mhatre AN, Han JJ, et al. Cationic liposome mediated transgene expression in the guinea pig cochlea. Hear Res. 1999;128:61.
169. Hoffer ME, Balough B, Kopke RD, et al. Morphologic changes in the inner ear of chinchilla langier after middle ear administration of gentamicin in a sustained-release vehicle. Otolaryngol Head Neck Surg. 1999;120:643-648.