24 Pediatric Neurosurgical Anesthesia
Pathophysiology
Intracranial Compartments
The skull can be compared to a rigid container with almost incompressible contents. Under normal conditions, the intracranial space is occupied by the brain and its interstitial fluid (80%), cerebrospinal fluid (CSF, 10%), and blood (10%). In pathologic states, space-occupying lesions such as edema, tumors, hematomas, or abscesses alter these proportions. The Monro-Kellie hypothesis, elaborated in the 19th century, states that the sum of all intracranial volumes is constant. An increase in volume of one compartment must be accompanied by an approximately equal decrease in volume of the other compartments, except when the cranium can expand to accommodate a larger volume. Gradual increases in intracranial volumes, such as a slow-growing tumor or hydrocephalus, can be compensated by the compliant nature of open fontanelles and sutures in young children; increasing head circumference can result.1 However, herniation can occur in children with open fontanelles if large increases in intracranial pressure (ICP) develop acutely. In the nonacute situation, the brain can compensate for pathologic increases in intracranial volume by intracellular dehydration and reduction of interstitial fluid.2–4
Under normal conditions, CSF exists in dynamic equilibrium, with absorption balancing production. The rate of CSF production in adults is approximately 0.35 mL/min or 500 mL/day.5 The average adult has 100 to 150 mL of CSF distributed throughout the brain and subarachnoid space. Children have correspondingly smaller volumes of CSF, but the rate of CSF production is similar to that of adults.5,6
Production of CSF is only slightly affected by alterations of ICP and is usually unchanged in children with hydrocephalus.6 Some drugs, including acetazolamide, furosemide, and corticosteroids, are mildly effective in transiently decreasing CSF production.1,7,8 There is an inverse relationship between the rate of CSF production and serum osmolality; an increase in serum osmolality causes a decrease in CSF production. Choroid plexus papillomas causing overproduction of CSF are rare but are more likely to occur during childhood.
Absorption of CSF is not well understood, but the arachnoid villi appear to be important sites for reabsorption of CSF into the venous system. One-way valves between the subarachnoid space and the sagittal sinus appear to open at a gradient of about 5 mm Hg. Some resorption may occur from the spinal subarachnoid space and from the ependymal lining of the ventricles. Resorption increases with an increase in ICP. However, CSF absorption is decreased by pathologic processes that obstruct arachnoid villi or interfere with CSF flow, such as intracranial hemorrhage, infection, tumor, and congenital malformations.9
Intracranial Pressure
Increased ICP causes secondary brain injury by producing cerebral ischemia and ultimately causing herniation. Ischemia occurs when ICP increases and cerebral perfusion pressure (CPP) decreases. As cerebral blood flow (CBF) and the supply of nutrients are curtailed, cell damage and death occur, leading to increased intracellular and extracellular water and further increases in ICP. When ICP increases, CPP can decrease, the brain can become ischemic, and cell death can ensue (Fig. 24-1).10
Signs of Increased Intracranial Pressure
The clinical signs of increased ICP vary in children. Papilledema, pupillary dilation, hypertension, and bradycardia may be absent despite intracranial hypertension, or these signs may occur with normal ICP.9,11 When associated with increased ICP, they are usually late and dangerous signs.12 Chronic increases in ICP are often manifested by complaints of headache, irritability, and vomiting, particularly in the morning. Papilledema may not be present even in children dying as a result of intracranial hypertension.13 A diminished level of consciousness and abnormal motor responses to painful stimuli are frequently associated with an increased ICP.9 Computed tomography (CT) or magnetic resonance imaging (MRI) can reveal small or obliterated ventricles or basilar cisterns, hydrocephalus, intracranial masses, and midline shifts. Diffuse cerebral edema is a common finding when increased ICP is associated with closed-head injury, encephalopathy, or encephalitis.
Monitoring Intracranial Pressure
Techniques to monitor ICP in adults have been successfully used in children.14–16 Ventricular catheters are generally accepted as the most accurate and reliable means of measurement, permitting removal of CSF for diagnostic or therapeutic indications. The major risks of intraventricular catheters are infection and hemorrhage; although rare, they can lead to devastating complications. These catheters may be difficult to insert precisely when they are needed most, as in a patient with severe cerebral edema with small ventricles. Compared with intraventricular catheters, subarachnoid bolts can be placed even when the ventricles are obliterated. This procedure minimizes trauma to brain tissue and poses less risk of serious infection and hemorrhage. The major disadvantages are that subarachnoid bolts may underestimate ICP, particularly in areas distant from their insertion site, and they are difficult to stabilize in infants with thin calvaria.
Epidural monitors that do not require a fluid interface can be implanted outside the dura, avoiding the risks of CSF contamination and the limitations of fluid-dependent systems.17,18 Most epidural systems correlate well with intraventricular measurements, but they cannot be recalibrated after insertion. Epidural monitors have also been secured noninvasively to the open anterior fontanelle of infants and appear to reflect changes in ICP. Fiberoptic catheters with self-contained transducers can also be used to measure ICP from intraventricular, subarachnoid, or intraparenchymal sites. These monitors avoid some of the problems of external fluid-filled transducers, but like epidural transducers, they cannot be recalibrated after insertion.
The normal ICP in children is less than 15 mm Hg. In term neonates, normal ICP is 2 to 6 mm Hg; it is probably even less in preterm infants. Children with intracranial pathology but normal ICP values occasionally exhibit pressure waves, which are considered abnormal.9 In children with open fontanelles, the ICP may remain normal despite a significant intracranial pathologic process; increasing head circumference may be the first clinical sign. Bulging fontanelles may not develop, especially when the process evolves slowly.
Intracranial Compliance
The absolute value of ICP does not indicate how much compensation is possible. If the ICP increases significantly, compensatory mechanisms have failed. However, pathologic states may be present despite an ICP within the normal range. Intracranial compliance (i.e., the change in pressure relative to a change in volume) is a valuable concept. Figure 24-2 is a schematic diagram of the relationship between the addition of volume to intracranial compartments and ICP. The shape of the curve depends on the time over which the volume increases and the relative size of the compartments. At normal intracranial volumes (point 1), ICP is low, but compliance is high and remains so despite small increases in volume. If volume increases rapidly, compensatory abilities are surpassed, and further increases in volume are reflected as increases in pressure. This can occur when the ICP is still within normal limits but the compliance is low (point 2). If the ICP is already increased further volume expansion causes a rapid increase in ICP (point 3). In clinical practice, compliance can be evaluated with a ventriculostomy catheter or by observing the response of ICP to external stimulation (e.g., tracheal suction, coughing, agitation).
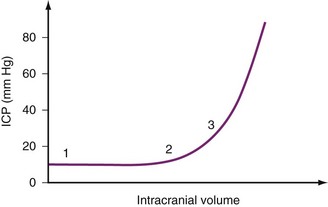
FIGURE 24-2 Idealized intracranial compliance curve for intracranial pressure (ICP) plotted against intracranial volume.
Several physiologic and mechanical factors such as a greater percentage of brain water content, less CSF volume, and greater percentage of brain content to intracranial capacity contribute to a relatively decreased intracranial compliance in children compared with adults.2 Children may be at increased risk for herniation compared with adults when similar relative increases in ICP have occurred. However, infants faced with a slowly increasing ICP may have a greater compliance due to their open fontanelles and sutures.
Cerebral Blood Volume and Cerebral Blood Flow
In addition to CSF, cerebral blood volume (CBV) represents another compartment in which compensatory mechanisms influence ICP. Although the CBV occupies only 10% of the intracranial space, changes related to dynamic blood volume occur, often initiated by anesthesia or intensive care procedures. As with other vascular beds, most intracranial blood is contained in the low-pressure, high-capacitance venous system. Increases in intracranial volume are initially met by decreases in CBV. This response is apparent in hydrocephalic infants, in whom venous blood shifts from intracranial to extracranial vessels, producing distended scalp veins.19
In the normal adult, CBF is approximately 55 mL/100 g of brain tissue per minute.20–22 This represents almost 15% of the cardiac output for an organ that accounts for only 2% of body weight. Estimates of CBF are less uniform for children. Normal CBF in healthy awake children is approximately 100 mL per 100 g of brain tissue per minute, which represents up to 25% of cardiac output.23,24 CBF in neonates and preterm infants (approximately 40 mL/100 g/min) is less than in children and adults.25,26 In infants, CBF is subject to modification by sleep states and feeding.27
CBF is regulated to meet the metabolic demands of the brain. In adults, the cerebral metabolic rate for oxygen consumption (CMRo2) is 3.5 to 4.5 mL O2/100 g/min; in children, it is greater.23 General anesthesia reduces CMRo2 by as much as 50%.28 Coupling of CBF and CMRo2 is probably mediated by the effect of local hydrogen ion concentration on cerebral vessels. Conditions that cause acidosis (e.g., hypoxemia, hypercarbia, ischemia) dilate the cerebral vasculature, which augments CBF and CBV. A reduction in brain metabolism (i.e., CMRo2) similarly reduces CBF and CBV. When autoregulation is impaired, CBF is determined by factors other than metabolic demand. If the CBF exceeds metabolic requirements, luxury perfusion or hyperemia exists. Many pharmacologic agents act directly on the cerebral vasculature to alter CBF and CBV.
Cerebrovascular Autoregulation
Effects of Blood Pressure
In adults, CBF remains relatively constant within a MAP range of 50 to 150 mm Hg (Fig. 24-3). Autoregulation enables brain perfusion to remain stable despite moderate changes in MAP or ICP. Autoregulation is partially mediated by myogenic control of arteriolar resistance. When CPP decreases, cerebral vessels dilate to maintain CBF, thereby increasing CBV. When CPP increases, cerebral vasoconstriction occurs, maintaining the CBF with a reduced CBV. When ICP and CVP are low, MAP normally approximates CPP. Beyond the range of autoregulation, CBF becomes pressure dependent. In children with chronic hypertension, the upper and lower limits of autoregulation are increased. Cerebral autoregulation can be abolished by acidosis, medications, tumor, cerebral edema, and vascular malformations, even at sites far removed from a discrete lesion.20
The limits of autoregulation are not known for normal infants and children, but autoregulation probably occurs at lower absolute values than in adults.29 Although the lower limit of autoregulation in adults is approximately 50 mm Hg, this blood pressure may be beyond that of the neonate. Intact autoregulatory mechanisms have been demonstrated within lower blood pressure ranges in newborn animals compared with mature animals.30 Cerebral autoregulation may also be abolished in critically ill humans.31
Effects of Oxygen
CBF is constant over a wide range of oxygen tensions. When the partial pressure of arterial O2 (Pao2) decreases to less than 50 mm Hg, CBF increases exponentially in adults; for example, at a Pao2 of 15 mm Hg, CBF is doubled compared with normal (see Fig. 24-3).32 The resulting increase in CBV increases ICP when intracranial compliance is low; the lower limit for Pao2 is probably less in neonates. Oxygen delivery is more important than the actual Pao2. Evidence suggests that hyperoxia decreases CBF. Kety and Schmidt demonstrated a 10% decrease in CBF in adults breathing 100% O2, although decreases of 33% have been reported in neonates.33,34
Effects of Carbon Dioxide
The relationship between the arterial partial pressure of carbon dioxide (Paco2) and CBF typically is linear (see Fig. 24-3). In adults, a 1-mm Hg increase in Paco2 increases CBF by approximately 2 mL/100 g/min.33 The direct effect of changes in Paco2 on CBF and the consequent effect on CBV are the basis for the fact that hyperventilation reduces ICP. Likewise, increases in Paco2 increase the CBF, although the limits at which this occurs in neonates differ from those in adults. In lambs and monkeys, CBF does not seem to change in response to decreased Paco2.35 There are no data to suggest what the limits of Paco2 are in human infants and children. Similarly, there is little information about the extent and duration of cerebrovascular responsiveness to hyperventilation in brain-injured and critically ill children. Moderate hyperventilation has been used to reduce ICP immediately, but several reports have demonstrated worsening cerebral ischemia in children with compromised cerebral perfusion.36–38
Autoregulation of CBF is impaired in areas of damaged brain.39 Blood vessels in an ischemic zone are subject to hypoxemia, hypercarbia, and acidosis, which are potent stimuli for vasodilation. These vessels develop maximally reduced cerebrovascular tone or vasomotor paralysis. Small, localized lesions may impair autoregulation in areas far removed from the site of injury.20 The extent of autoregulatory impairment varies in brain-damaged children.
Management of Anesthesia
Preoperative Evaluation
History
Preoperative evaluation of infants and children is discussed in Chapter 4. Children who are scheduled for neurosurgery might have been healthy until the onset of their symptoms, might have been developmentally delayed from birth, or may have impaired neuromuscular function. The anesthetic plan, including postoperative care, needs to consider the particular issues of each child and the disease state.
A history of food or drug allergies, eczema, or asthma may provide warning of an adverse reaction to the contrast agents frequently used in neuroradiologic procedures. Special attention should be given to symptoms of allergy to latex products, such as lip swelling after blowing up a toy balloon or tongue swelling after insertion of a rubber dam is into the mouth by a dentist, because latex anaphylaxis has been reported in some children who have undergone multiple operations, especially those with a meningomyelocele.40 Latex allergic children may also report allergies to fruits (e.g., kiwi, banana, avocado, strawberry, and others).
Concurrent pediatric diseases and symptoms of neurologic lesions may influence the conduct of anesthesia. Protracted vomiting, enuresis, and anorexia due to intracranial lesions should prompt evaluation of hydration and electrolytes. Diabetes insipidus or inappropriate secretion of antidiuretic hormone is common. A history of the use of aspirin or aspirin-containing remedies for headaches or respiratory tract infections is information that is not usually forthcoming but may have important implications for operative and postoperative bleeding. Corticosteroids are often initiated at the time of diagnosis of intracranial tumors, and they should be continued and a pulse dose administered during the perioperative period. Therapeutic concentrations of anticonvulsants should be verified preoperatively and maintained perioperatively. Children receiving long-term anticonvulsants may develop toxicity, especially if seizures are difficult to control; this is frequently manifested as abnormalities in hematologic or hepatic function, or both. Children receiving chronic anticonvulsant therapy may also require increased amounts of sedatives, nondepolarizing muscle relaxants, and opioids because of enhanced metabolism of these drugs (see also Chapters 6 and 22).41–43
Physical Examination
The physical examination should encompass a brief neurologic evaluation, including level of consciousness, motor and sensory function, normal and pathologic reflexes, integrity of the cranial nerves, and signs and symptoms of intracranial hypertension. Examination of pupillary size and responsiveness can detect benign anisocoria. Preoperative respiratory assessment should include the effects of motor weakness, impaired gag and swallowing mechanisms, and evidence of active pulmonary disease, such as aspiration pneumonia. Muscle atrophy and weakness should be documented, because upregulation of acetylcholine receptors may precipitate sudden hyperkalemia after administration of succinylcholine and induce resistance to nondepolarizing muscle relaxants in the affected limbs.44
Laboratory and Radiologic Evaluation
In all but the most minor procedures, laboratory data should include a hematocrit determination. Blood typing and crossmatching should be performed for any major procedure. The need for additional studies, such as evaluation of coagulation parameters, serum electrolyte levels and osmolality, blood urea nitrogen and creatinine values, arterial blood gas analysis, chest radiography, or electrocardiography (ECG), is determined on an individual basis. Liver function tests and a hematologic profile should be obtained if not recently reviewed in children on long-term therapy with anticonvulsants. Specific neuroradiologic studies are usually obtained by the neurosurgeon and should be reviewed by the anesthesiologist. For example, the anesthesiologist should know which children with a ventriculoperitoneal shunt have “slit ventricles,” because these children have special risks in the perioperative period45 (see “Hydrocephalus”). Information on the amount of sedation needed to perform radiologic studies may also be helpful in planning the induction of anesthesia. Preoperative neurophysiologic studies, including electroencephalography (EEG) and evoked potentials, may provide a baseline for comparison of intraoperative and postoperative evaluations.
Monitoring
An arterial catheter is placed for craniotomies in which there is a potential for sudden and severe hemodynamic changes. Small child size should not preclude the use of invasive monitoring and may actually be an indication for a more aggressive approach. An increase in the paradoxical arterial pressure waveform with positive-pressure ventilation is often an excellent indication of intravascular volume deficiency and the need for fluid replacement (see Fig. 10-10). Intraarterial catheters can be placed percutaneously in the radial, dorsalis pedis, or posterior tibial arteries even in small infants, and it is rarely necessary to resort to surgical cutdown. The arterial transducer should be zeroed at the level of the head if the head and heart positions are different so that CPP can be accurately assessed. The lateral corner of the eye or the external auditory meatus approximate the level of the foramen of Monro, and either is a convenient landmark. In the first days of life, the umbilical artery and the umbilical vein can be cannulated. These catheters should be discontinued as soon as alternative access is established because of the potential for serious complications.
Percutaneous central venous cannulation (i.e., external or internal jugular, femoral, or subclavian veins) using the Seldinger technique is possible even in the smallest infants (see Chapter 48). However, in children undergoing neurosurgical resections, consideration should be given to sites other than neck veins, such as the femoral vein, thereby avoiding the Trendelenburg position during catheter insertion and the risk of accidental carotid artery puncture and hematoma formation, which may compromise CBF and intracranial venous drainage. If there is no issue with ICP, the subclavian vein is a reasonable alternative. Cannulation of antecubital veins may provide central venous access, but threading the catheter into the inlet of the right atrium may be technically difficult in small children. When rapid blood loss is a consideration in a small child in whom adequate peripheral venous access is difficult to obtain, a single-lumen, large-bore catheter is most commonly inserted in a femoral vein. Catheters inserted into the femoral veins usually are accessible to the anesthesiologist during most neurosurgical procedures. Multiple-lumen central venous catheters are inadequate for rapid blood transfusion. All central catheters should be removed as soon as possible after the procedure to minimize the risk of venous thrombosis.
Induction
For children with intracranial hypertension, the primary goals during induction are to minimize severe increases in ICP and decreases in blood pressure. Most intravenous drugs decrease CMRo2 and CBF, which consequently decreases ICP.46 Historically, sodium thiopental (4 to 8 mg/kg) was the default induction agent for neurosurgical cases. However, sodium thiopental is no longer available in the United States, although it remains available in other countries. In the United States, propofol has become the intravenous induction agent of choice for most children. Propofol (2 to 4 mg/kg) appears to have similar cerebral properties and an antiemetic effect; however, its antiemetic effect is usually not relevant for lengthy procedures. Etomidate, a possible neuroprotective agent, can be used if hemodynamic stability is a concern.47–49 Ketamine should be avoided because of its known ability to increase cerebral metabolism, CBF, and ICP. Sudden increases in ICP have been reported after ketamine administration, especially in infants and children with hydrocephalus.50,51
Other measures to reduce ICP during induction include controlled hyperventilation and administration of fentanyl and supplemental hypnotics before laryngoscopy and intubation. Lidocaine (1.5 mg/kg) limits the increase in ICP when administered intravenously just before laryngoscopy.52
Sevoflurane has replaced halothane for inhaled inductions because of its more rapid onset, acceptability for pediatric patients, and hemodynamic stability. Similar to isoflurane in its cerebral physiologic effects, sevoflurane with hyperventilation appears to blunt the increase in ICP due to cerebral vasodilatation from inhalational anesthetic agents alone.53–55 Sevoflurane offers an additional advantage because it causes less myocardial depression compared with halothane.56 However, sevoflurane when combined with hyperventilation produces epileptiform activity as measured by EEG. This may occur even in children with no history of clinical seizure activity (see Chapter 6).57
Airway Management and Intubation
Contraindications to nasal intubation include choanal stenosis, possible basilar skull fracture, transsphenoidal procedures, and sinusitis. If nasotracheal intubation is planned, it is advantageous to prepare the nares with topical vasoconstrictors, recognizing that systemic hypertension can occur in response to nasally administered vasoconstrictors. Placing a few drops of 0.25% phenylephrine (Neo-Synephrine) or oxymetazoline on cotton-tipped applicators and positioning them in the nares against the nasal mucosa can prevent overdosage and help to gauge the patency of the nasal passage when anesthesia has been induced. It may also be useful to use a red rubber catheter or a nonlatex nasal trumpet to gently dilate the nares and minimize the risk of bleeding.58 Whichever route is chosen for intubation, it is important to secure the tracheal tube with care because loss of airway intraoperatively in a prone child in pins or a child with limited airway access can result in disaster.
Neuromuscular Blocking Drugs
Because of its rapid onset and brief duration of action, succinylcholine is frequently used to facilitate intubation in children with a full stomach. The intubating dose is 1 to 2 mg/kg given intravenously or 4 to 5 mg/kg given intramuscularly.59 In children, it may be safest to precede this with atropine (0.01 to 0.02 mg/kg) to prevent bradycardia. Succinylcholine does not significantly increase ICP in humans,60 and any effect may be minimized by pretreatment with a nondepolarizing muscle relaxant.61 However, this may make succinylcholine less effective, even when the dose of succinylcholine is increased. Succinylcholine is contraindicated when it may induce life-threatening hyperkalemia in the presence of denervation injuries due to various causes, including severe head trauma, crush injury, burns, spinal cord dysfunction, encephalitis, multiple sclerosis, muscular dystrophies, stroke, or tetanus.62
Alternatively, nondepolarizing muscle relaxants such as rocuronium, pancuronium, cisatracurium, or vecuronium may be used, but all have a slower onset of action than succinylcholine. However, when rocuronium is administered in sufficiently large doses (1.2 mg/kg), the onset of action is comparable with that of succinylcholine, with equivalent intubating conditions achieved in less than 1 minute.63
Positioning
After the child is in the operating room, the neurosurgeons and anesthesiologists must have adequate access to the child. In infants and small children, slight displacement of the tracheal tube can result in extubation or endobronchial intubation. During prolonged procedures, it is important for the anesthesiologist to be able to visually inspect the tracheal tube and circuit connections and to suction the tracheal tube when necessary. Using proper draping and a flashlight, the operator can usually create a “tunnel” to ensure access to the airway. All but very small children are placed in pins in a Mayfield head holder. The direction of the tube exiting the nares should be adjusted to remove pressure and avoid the risk of ischemia, particularly for cases that will continue for several hours. Neonates and small infants have thin calvaria, so head-pinning systems are often avoided. Instead, there are a variety of non–pin-based headrests available for these children. Adequate padding should be used in such situations (Figs. 24-4 and 24-5). Extreme head flexion can cause brainstem compression in children with posterior fossa pathology, such as a mass lesion or Arnold-Chiari malformation. Extreme flexion can also cause high cervical spinal cord ischemia and tracheal tube kinking and obstruction.64
Extremities should be well padded and secured in a neutral position (i.e., palm supinated or neutral to avoid ulnar nerve compression). It is important to avoid stretching peripheral nerves and to prevent skin and soft tissue pressure injury because of direct contact with surgical accessories such as instrument stands and grounding wires (see Fig. 24-5). It is also important to ensure that extremities that are not directly visible to the anesthesiologist (e.g., those on the opposite side of the operating room table) cannot fall off the table during surgery, even if the table is rotated. In older children and adolescents undergoing prolonged procedures, deep vein thrombosis prophylaxis should be considered using compression or pneumatic stockings.65,66
Prone Position
The head position depends on the surgical procedure. If surgery is limited to the lower spine, the head may be rotated and supported by padding, with care taken to avoid direct pressure on the eyes and nose and to keep the ears flat. For posterior fossa surgery, the head usually is suspended in pins to maintain central alignment of the head and maximal flexion. For infants and toddlers, a cerebellar head frame is another alternative when the cranium is too thin for pins. In this situation, the child’s forehead and cheeks rest on a well-padded head frame, and the eyes are free in the center of a horseshoe-shaped support. Ensure that the tracheal tube is properly positioned (after taping) and does not migrate to a main-stem position while positioning the child prone. This can be confirmed while the child is still supine by flexing the child’s head onto the chest and auscultating air entry bilaterally. Tape used to fix other tubes (e.g., gastric, esophageal) should not adhere to the tracheal tube tape so that accidental dislodgement of these tubes does not cause an extubation. An emergency plan should be formulated to turn the child supine if it suddenly becomes necessary.67
Significant airway edema may develop in a child who is in the prone position for an extended period. Oral airways are best avoided because they can cause edema of the tongue. Alternatively, a folded piece of gauze can be inserted between the teeth to prevent the tongue from extruding. Rarely, prophylactic postoperative intubation may be necessary if a great deal of facial swelling has developed during a prolonged surgery. Postoperative vision loss has been linked with prolonged spine surgery in the prone position and significant blood loss.68 Avoidance of direct pressure on the globe of the eyes, staged procedures to decrease surgical time, and maintenance of stable hemodynamics with avoidance of excessive intraoperative fluid administration should be ensured in prone children.69
Sitting Position
The sitting position is now used less commonly in pediatric neurosurgical procedures and is rarely used in children younger than 3 years of age. However, this position may be used for morbidly obese children who cannot tolerate the prone position due to excessive intrathoracic and abdominal pressures. When it is used, precautions to prevent hypotension and air embolism must be followed. The lower extremities should be wrapped in elastic bandages. The head must be carefully flexed to avoid kinking the endotracheal tube, advancing it into a bronchial position, or avoid compressing the chin on the chest, which can block venous and lymphatic drainage of the tongue. Extreme flexion can also result in brainstem or cervical spinal cord ischemia, or both. As in the prone position, nasotracheal tubes are often used because they are more secure. The child’s upper extremities are supported in the child’s lap. Control levers to lower the head position should be easily accessible to the anesthesiologist and unencumbered by wires and drapes (see Fig. 24-5).
Local Anesthesia
Local anesthetic should be injected subcutaneously before a skin incision to provide analgesia, and epinephrine is included in the local anesthetic to reduce cutaneous blood loss. If 0.25% bupivacaine with 1 : 200,000 epinephrine is used, the dose should be limited to 0.5 mL/kg. When greater volumes are required, the solution can be diluted with normal saline. This dilute solution is still effective for vasoconstriction and provides a prolonged sensory block postoperatively. Specific blocks of supraorbital and supratrochlear nerves can provide analgesia from the frontal area to the midcoronal portion of the occiput.70 Blockade of the great occipital nerve provides analgesia from the posterior of the occiput to the midcoronal area of the occiput, whereas block of the supraorbital nerve provides analgesia to the front of the occiput (see Figs. 41-9 and 41-10).71,72
Maintenance of General Anesthesia
Maintenance of general anesthesia can be accomplished using inhalational anesthetics, intravenous infusions, or a combination of these drugs. Anesthetics that decrease ICP and CMRo2 and maintain CPP are most desirable (Table 24-1). The commonly used inhalational agents uncouple CBF and CMRo2 such that CBF increases while CMRo2 decreases. All potent inhalational agents are cerebral vasodilators, which increase the CBF and ICP. Low concentrations of isoflurane, sevoflurane, or desflurane, combined with ventilation to maintain normocarbia, minimally affect CBF and ICP.53,54,73 Isoflurane is often the inhalational agent of choice for maintenance of neuroanesthesia. At two times the minimal alveolar concentration (MAC), this dose of isoflurane induces a level of anesthesia that is associated with an isoelectric EEG while, unlike several other inhalational agents, maintaining hemodynamic stability. Enflurane is no longer used and may be epileptogenic, especially when combined with hyperventilation.74 Other studies have demonstrated a similar effect with sevoflurane and hyperventilation, but the clinical implications of this are yet to be defined.75
Practitioners debate the routine use of nitrous oxide for intracranial neurosurgical procedures. Opponents cite the increased risk of postoperative nausea and vomiting (PONV) with nitrous oxide in a surgical population already at greater risk for PONV.76 Proponents cite studies that failed to demonstrate an increased risk of PONV.77 Nitrous oxide can increase CBF in humans in a dose-dependent fashion through cerebral vasodilatation.78,79 This increase in CBF can lead to an increase in ICP, which can be deleterious if the child already has reduced intracranial compliance.80 Nitrous oxide can also affect somatosensory and motor evoked potentials, especially when concentrations in excess of 50% are used.81–83 Animal data have shown that nitrous oxide can counteract the protective effects of thiopental in a model of cerebral ischemia.84
Proponents of the use of nitrous oxide for intracranial procedures cite the long track record of safety. There are no outcome studies in humans showing a difference between using nitrous oxide or not. It is often of great clinical interest to obtain a neurologic assessment immediately after the conclusion of an intracranial procedure, and some practitioners prefer the use of nitrous oxide to aid in achieving this goal. Studies have demonstrated the safety of using nitrous oxide in a variety of combinations with other agents during intracranial procedures.85 Nitrous oxide is relatively contraindicated, however, if the child has undergone a craniotomy within the past few weeks because air can remain in the head for prolonged periods after previous neurosurgery.86
Fentanyl is often administered as part of an opioid-based technique because it is easily titratable with minimal adverse effects. A common loading dose is 5 to 10 µg/kg, with a dose of 2 to 5 µg/kg/hr usually adequate for maintenance. Adverse effects, including hypotension, can be avoided by giving the loading dose incrementally. Practitioners commonly use other opioids such as remifentanil and sufentanil. Dexmedetomidine, an α2-agonist sedative, has also been used in children for neurophysiologic monitoring, for awake craniotomies, to facilitate smooth wake-ups after neurosurgical procedures, and for neuroprotection.87–90
Apoptotic Neurodegeneration
Several investigators have demonstrated that commonly used anesthesia drugs accelerate programmed cell death (i.e., apoptosis) in the CNS of immature rodents and rhesus monkeys.91–93 This laboratory observation has provoked a heated debate about its relevance to anesthetizing neonates,94–97 which has been extended to the lay press.98 Although these experimental paradigms have yielded some surprising findings, extrapolating these data to the practice of anesthetizing human neonates is questionable (see Chapter 23).
The animal and in vitro studies have significant limitations with respect to the experimental model, agent dosage or concentration, duration of exposure (absolute and compared with human exposures), lack of surgical stimulation, and developmental age and stage. No detectable clinical marker or syndrome is associated with early anesthesia exposure in former neonates who have undergone surgery and anesthesia at birth or in the first several years of life during rapid brain growth (i.e., synaptogenesis). In the only primate study, the degree of apoptosis after 3 hours of a ketamine infusion was similar to that of the control but significantly less than after a 24-hour infusion.93 This occurred in the presence of blood concentrations of ketamine that were 10-fold to several hundred-fold greater than those reported after a single dose of ketamine in infants. These findings suggest that in this model, ketamine-associated neurodegeneration is a time-dependent, dose-dependent phenomenon whose limits have not been established.
Despite the confounding effects of prematurity and coexisting congenital anomalies, clearly characterized syndromes have been associated with maternal consumption of alcohol and anticonvulsant drugs. Discrepancies in neurocognitive outcomes exist.92,99 Most neonatal and infant surgery is urgent, and anesthesia care is essential to proceed safely. Several retrospective database studies suggest that multiple anesthesia episodes are associated with learning disabilities and cognitive dysfunction, but most of these children were anesthetized before pulse oximetry and capnography were a standard of care. Unrecognized episodes of hypoxemia or excessive ventilation with reduced CBF might have contributed. It is also unclear whether children who required more than one surgical procedure when younger than 4 years of age might have had neurocognitive developmental issues that were associated with the pathology requiring surgery and were totally separate from exposure to anesthetic agents.100–102 One retrospective study demonstrated that identical twins who were discordant for general anesthesia and surgery showed no evidence of cognitive dysfunction in follow-up assessments.103
Blood and Fluid Management
Fluid and blood product management is discussed in Chapters 8 and 10. Disruption of the blood-brain barrier by underlying pathologic processes, trauma, or surgery predisposes neurosurgical patients to cerebral edema, which may be exacerbated by excessive administration of intravenous fluids. Intravenous fluid management during neurosurgical anesthesia involves cerebral perfusion, cerebral edema, water and sodium homeostasis, and serum glucose concentration.
In most cases, blood transfusions are not planned and attempts are made to avoid administration of blood products with their associated risks. Crystalloid solutions are commonly administered. Lactated Ringer solution is not considered a truly isotonic solution because its osmolality is 273 mOsm/L (normal: 285 to 290 mOsm/L). Normal saline, which is slightly hypertonic (308 mOsm/L), is the fluid of choice because reduction of serum osmolality is not desirable. However, rapid infusion of large volumes of normal saline has been associated with a hyperchloremic non-anion gap metabolic acidosis.104 The clinical significance of this acidosis is not clear. If there are large fluid requirements during surgery, alternating bags of lactated Ringer solution with normal saline can minimize the risk of hypernatremia and acidosis and avoid hypoosmolality.
Inducing dehydration with osmotic and loop diuretics is a useful strategy to minimize cerebral edema and provide an optimal surgical field. However, hypotension and rebound effects may be associated with their use. Rapid administration of hypertonic solutions can cause profound but transient hypotension due to peripheral vasodilation.105 Glucose-containing solutions usually are unnecessary during neurosurgical procedures because blood glucose concentrations are well-maintained even in small children in the absence of intravenous glucose administration during typical (balanced) neurosurgical anesthetics. However, glucose may be indicated when hypoglycemia is a concern, such as in diabetic children, children receiving hyperalimentation, preterm and full-term neonates, and malnourished or debilitated children. In these situations, glucose solutions should be administered at or slightly below maintenance rates (by constant infusion pump) and serum glucose concentrations should be monitored periodically throughout surgery. The potential association of larger cerebral infarct size with hyperglycemia (i.e., blood glucose values in excess of 250 mg/dL) during ischemia is of particular concern.106
Temperature Control
Because the head accounts for a large proportion of an infant’s body surface area, infants are particularly susceptible to heat loss during neurosurgical procedures. Attention should be focused on maintaining normal temperature from the time the child is brought into the operating room, although moderate hypothermia during neurosurgery may be useful to decrease the CMRo2. Ambient room temperature should be increased during positioning, preparation, and draping. Infrared warming lights may be helpful for infants, and warming blankets may be useful for infants weighing less than 10 kg. Forced-air warming remains the most effective means of maintaining body temperature.107
Venous Air Emboli
Venous air embolism (VAE) is a potential danger during intracranial procedures. The larger the pressure gradient between the operative site and the heart, the greater the potential for clinically significant entrainment of air into the central circulation.108 For example, when the operative site is far above the heart (e.g., in a seated craniotomy) or when the CVP is low (e.g., acute blood loss during craniofacial procedures), it creates an environment for a VAE. Intracranial procedures are a particular concern because intracranial venous sinuses have dural attachments that impede their ability to collapse. Other potential air entry sites during neurosurgical procedures include bone, bridging veins, and spinal epidural veins. The sequence of events that should be followed when a VAE occurs is to identify the problem, stop further air entrainment, and support the circulation. Understanding the cause, prevention, and treatment of VAE is crucial because the consequences can be life-threatening.
When air enters the central circulation, it can accumulate in the right atrium or the right ventricular outflow tract. Cardiac output may be reduced, depending on the size of the air lock. If enough air is entrained into the circulation, the preload to the right ventricle decreases, or the right-sided heart afterload increases acutely, which can lead to cor pulmonale, acutely decreasing left ventricular preload and ultimately causing cardiovascular collapse. One study in dogs demonstrated that as little as 1 mL/kg of air could increase pulmonary artery pressure 200% to 300%.109 Intracardiac shunts such as a patent foramen ovale, atrial or ventricular septal defects, and other congenital cardiac defects may allow air to access the systemic circulation, including the coronaries and brain. The risk of VAE is even greater in infants and children because potential intracardiac shunts exist in many otherwise healthy infants and children. They may become clinically important if pulmonary hypertension develops acutely after a large air embolism. Some clinicians recommend preoperative echocardiographic screening for patent foramen ovale in any child being considered for a sitting craniotomy; others regard a patent foramen ovale to be an absolute contraindication to the sitting position.110,111
Although the incidence of VAE is greatest in the sitting position, the lateral, supine, and prone positions are not free of risk. VAE have also been observed during craniotomy for craniosynostosis, even when the operating room table is flat and rarely when the surgery involved endoscopic strip craniectomy, although most occur without clinical sequelae.112,113 The incidence of VAE in children undergoing suboccipital craniotomy in the sitting position is not significantly different from that in adults, but children appear to have a greater incidence of hypotension and a smaller likelihood of successful aspiration of central (intravascular) air.114
Several techniques may be used to detect VAE. Depending on the study design, the usual order of sensitivity of detecting air in the heart is transvenous intracardiac echocardiography (0.15 mL/kg) > transesophageal echocardiography (0.19 mL/kg) = precordial Doppler probe (0.24 mL/kg) > pulmonary artery pressure (0.61 mL/kg) = end-tidal CO2 tension (0.63 mL/kg) = arterial O2 tension > mean arterial pressure (1.16 mL/kg) = arterial CO2 tension.115,116 Transvenous intracardiac echocardiography is commonly used to guide catheters in cardiac ablation procedures or insertion of foramen ovale occlusion devices, but it is invasive and infrequently used in pediatric anesthesia. A precordial Doppler probe has been traditionally placed over the fourth or fifth intercostal space at the right sternal border to best monitor right heart sounds, although evidence suggests that placing the Doppler probe at the left parasternal border may be at least as sensitive (Fig. 24-6).117,118 Appropriate Doppler positioning can be confirmed by listening for the characteristic change in sounds after rapid administration of a few milliliters of saline into a venous catheter. The precordial Doppler probe is particularly valuable because it is inexpensive, easy to use, benign, and noninvasive. Although transthoracic or transesophageal echocardiography is the most specific method for detecting small air emboli, it is not easily used intraoperatively, especially in children during neurosurgical procedures.110,119,120
Monitoring end-tidal gas tensions is important during neurosurgical procedures. When VAE occurs, there is a ventilation/perfusion mismatch caused by the air blocking passage of blood through the pulmonary circulation, increasing dead space ventilation with a sudden decrease in end-tidal CO2 partial pressure and activation of complement resulting in pulmonary interstitial edema, neutrophil infiltration, and lung injury (Fig. 24-7).115 The end-tidal CO2 partial pressure remains a useful and cost-effective strategy in diagnosing massive VAE, although its sensitivity has been surpassed by other approaches (see Fig. 24-6). An increase in end-tidal nitrogen partial pressure during continuous monitoring is a specific sign of air emboli. Although slightly more sensitive than a decrease in end-tidal CO2, an increase in end-tidal nitrogen is not detected by most infrared analyzers in practice and is usually of such small magnitude that it may be difficult to detect.
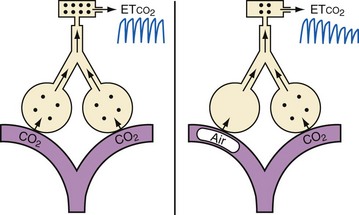
FIGURE 24-7 Mechanism of decreased end-tidal carbon dioxide (etco2) after an air embolus.
(Courtesy J. Drummond, MD.)
Aspiration of air from a central venous catheter is rarely successful unless massive amounts have been entrained. When central venous catheters are necessary, such as for a child in the sitting position or when massive blood loss is anticipated, an attempt should be made to place the tip at the junction of the superior vena cava and right atrium to provide the optimal location for aspiration of entrained air. More importantly, a central venous catheter is useful to estimate maintenance of circulating blood volume and to rapidly administer fluids and resuscitative medications when necessary. The position of a central venous catheter near the heart should be confirmed by radiograph, by transducing CVPs, or with the aid of ECG monitoring (i.e., biphasic P waves develop in a lead at the tip of the catheter). The threshold for aspirating air may be increased by properly positioning a multiple-orifice central venous catheter using a transvenous intracardiac echocardiography probe.115 Because erosion of the catheter tip through the heart causing fatal pericardial tamponade has occurred after surgery in small children, soft silicone catheters are recommended.121,122
Emergence
Protecting the brain is a major concern during neurosurgical procedures (Table 24-2). Emergence and extubation should be smooth and controlled to prevent fluctuations in ICP and in venous and arterial pressures.123 To avoid vomiting during emergence, a multimodal antiemetic approach is advised.124 Despite this approach, the incidence of PONV is high, which may be attributed to several factors: blood in the CSF is a potent emetic, opioids are often used to treat postoperative pain, and headache itself can precipitate emesis.
Goals | Avoid cerebral edema |
Avoid cerebral hypoxia | |
Avoid cerebral hypoperfusion | |
Avoid cerebral hypermetabolism | |
Avoid neuronal membrane damage | |
Maneuvers | |
Head of bed at 30 degrees in midline | Increases cerebral venous drainage while maintaining CPP |
Corticosteroids | May improve outcome in spinal cord injury |
Decrease vasogenic cerebral edema in children with tumors | |
Stabilize neuronal membranes | |
Free-radical scavengers | |
Controlled ventilation | Maintain Paco2 at normal to slightly low levels: prevents both cerebral vasodilation and increased ICP |
Muscle paralysis | Avoids coughing, straining, child movement, and other causes of increased ICP |
Ventricular drainage | Decreases ICP |
Antihypertensives | Prevent further cerebral edema, ischemia, and cerebral hemorrhage. Severe hypotension can significantly decrease CPP. |
Anticonvulsants | Prevent seizure activity and increased ICP |
Hypothermia | Decreases CMRo2 and CMRglu consumption |
Barbiturate coma | Membrane-stabilizing effect |
Decreases CBF and CMRo2 |
CBF, Cerebral blood flow; CMRglu, cerebral metabolic rate for glucose; CMRo2, cerebral metabolic rate for oxygen; CPP, cerebral perfusion pressure; ICP, intracranial pressure; Paco2, partial pressure of arterial carbon dioxide.
Intravenous lidocaine (1.0 to 1.5 mg/kg) given before extubation may help to suppress coughing and straining on the tracheal tube, although fentanyl appears to be equally effective and may be less sedating. Labetalol, an α- and β-adrenergic blocking agent, can be administered incrementally for the control of blood pressure during the acute period of emergence, but this is rarely necessary in children who have received adequate doses of opioids during surgery. For adolescents, intravenous labetalol (0.1 to 0.4 mg/kg given every 5 to 10 minutes until desired effect is achieved) may be necessary, but this usually does not have to be repeated in the postoperative period. Esmolol is used by some practitioners and has been as effective as labetalol in controlling hypertension after intracranial surgery in adults.125 However, esmolol should be used with caution in infants and smaller children because their cardiac output depends on heart rate. There are no studies evaluating the use of esmolol in children for such an application. Dexmedetomidine may be useful in facilitating a smooth emergence while still allowing evaluation of the child’s neurologic status.
Pain is usually not severe after a craniotomy, but it can be treated with incremental doses of opioids. Ketorolac is best avoided in the early postoperative period because of its effects on platelet function. Acetaminophen may be administered orally, rectally, or intravenously for mild pain.126
Diabetes insipidus or inappropriate secretion of antidiuretic hormone may complicate postoperative fluid and electrolyte management, particularly when surgery is in the region of the hypothalamus and pituitary gland. Careful observation of fluid status and repeated laboratory evaluation of blood and urine osmolality and sodium levels are important in this situation. When diabetes insipidus occurs, it can be managed with a continuous infusion of dilute aqueous vasopressin (1 to 10 mU/kg/hr).127 In such circumstances, large volumes of hypotonic intravenous solutions must be avoided because they may rapidly decrease the serum sodium level and osmolality. If normal saline is administered in strictly limited volumes, aqueous vasopressin can control the electrolyte and fluid balances of children with diabetes insipidus until they resume oral fluids. At that time, intranasal or oral desmopressin (DDAVP) can be substituted. When diabetes insipidus develops after surgery in the pituitary region (e.g., during resection of a craniopharyngioma), it may only be transient, and it is important to repeatedly assess the need for vasopressin.
Special Situations
Trauma
Head Injury
Among children, trauma is the primary cause of death, and head injuries produce most of this mortality and cause much of the morbidity in survivors.128–130 Motor vehicle accidents continue to be the most frequent preventable cause of head injury, although domestic violence and sports-related head injury are also common in children (see Fig. 38-1). Assaults and suicide attempts have become increasingly common among adolescents.
Children with head trauma may have minimal neurologic abnormalities at the time of initial evaluation. However, increased ICP and neurologic deficits may progressively develop. They develop slowly because brain injuries occur in two stages. The primary insult that occurs at the time of impact results from the biomechanical forces that disrupt the cranium, neural tissue, and vasculature. The secondary insult is the parenchymal damage caused by the pathologic sequelae of the primary insult. These changes can result from hypotension, hypoxia, cerebral edema, or intracranial hypertension. Whereas prevention of primary injuries must be addressed in a sociopolitical forum such as through seatbelt laws, sports injury prevention, and domestic violence legislation, anesthesiologists are instrumental in preventing or minimizing secondary insults (see Chapter 38).
There are significant differences between children and adults in the pattern of CNS injuries. Although intracranial hematomas (i.e., epidural, subdural, or intraparenchymal) are common in adults, they are less common in children. In contrast, diffuse cerebral edema after blunt head trauma occurs more often in children than in adults.131
Skull Fractures
Skull fractures are a common manifestation of head trauma in children. Most are linear and do not require surgical treatment. These fractures are of concern primarily because the force required to produce them may damage the underlying brain and vasculature. A linear fracture over a major blood vessel (e.g., middle meningeal artery) or a large dural sinus may result in intracranial hemorrhage. Most children have an uneventful course after sustaining a simple skull fracture. A few develop a leptomeningeal cyst or growing fracture that eventually requires surgical treatment. Multiple skull fractures in the absence of documented major trauma should always raise the suspicion of child abuse (See Fig. 38-2), which is also referred to as nonaccidental trauma.
Depressed skull fractures often require surgical repair. They may occur even in the absence of a scalp laceration. However, displacement of the inner table of the skull requires greater force than that needed to produce a simple linear fracture and has greater potential to damage underlying tissues. Approximately one third of all depressed fractures are uncomplicated, another third are associated with dural lacerations, and the remaining third are associated with cortical lacerations. The extent of cortical injury is the primary determinant of morbidity and mortality. Surgical débridement and elevation of the depressed bone are usually performed as soon as possible after the injury (Fig. 24-8, A).
Basilar skull fractures are less common in children. Despite the force needed to produce these fractures, they typically have an excellent prognosis and rarely require surgical intervention. However, the possibility of a basilar skull fracture should be considered when caring for children with altered mental status, seizures, or associated trauma requiring surgery. Findings include periorbital ecchymoses (“raccoon eyes”), retroauricular ecchymosis (i.e., the Battle sign) (see Fig. 38-2, B), hemotympanum, clear rhinorrhea, or otorrhea. Unless absolutely necessary (e.g., mandibular wiring), nasotracheal intubation or passage of a nasogastric tube is best avoided because these tubes have inadvertently traversed these skull fractures and entered the cranium.132–134 Complications of basilar skull fracture include meningitis from a CSF leak, cranial nerve damage, and anosmia.
Epidural Hematoma
Epidural hematomas most commonly develop in the temporoparietal region due to arterial bleeding from a severed middle meningeal artery. They can also develop in the posterior fossa as a result of bleeding from a venous sinus. Epidural hematomas are not necessarily associated with an overlying skull fracture. The classic natural history in adults is a “lucid interval” between the initial loss of consciousness and subsequent neurologic deterioration. Infants and children may not demonstrate an altered mental status in the early stages after the injury. However, as the hematoma expands, it can lead to a loss of consciousness, hemiparesis, and pupillary dilatation. This deterioration can be quite rapid once a mass effect occurs. Treatment is prompt surgical evacuation because delays are associated with increased morbidity. Medical therapy directed at decreasing ICP should be instituted as soon as a diagnosis is suspected but should not delay surgical repair (see Fig. 24-8, B). Children recover well after these hemorrhages, although morbidity is usually a reflection of underlying brain injury or lengthy delay in treatment.
Subdural Hematoma
Subdural hematomas are usually associated with cortical damage resulting from direct parenchymal contusion or laceration of venous blood vessels. Acute subdural hematomas are almost always traumatic and are frequently a result of abuse, such as shaking of small children, particularly those younger than 1 year of age. Shaken baby syndrome occurs when an infant is shaken so vigorously that significant neuronal disruption occurs and tears in the cortical bridging veins cause subdural hematomas.135–137 These infants suffer significant brain damage complicated by episodes of apnea and further hypoxic insult.
Spinal Injury
Although isolated cervical spine injuries are uncommon in children, those with severe head trauma should always be managed as if they also have a cervical spine injury.138,139 Different causes of spinal injuries are associated with specific age groups. Motor vehicle accidents produce the largest number of injuries in older children and adolescents, whereas birth injuries and falls are the most common cause in infants and young children.140 Spinal cord injury itself may be caused by a variety of forces, including hyperflexion, hyperextension, rotation, vertical compression, flexion rotation, and shearing. The injury may involve bony, ligamentous, cartilaginous, vascular, or neural components of the spine or adjacent structures. The biomechanics and functional anatomy of the pediatric spine depend on the age of the child. Older children and teenagers are more likely to sustain injuries in the thoracolumbar region of the spine, whereas infants and younger children are more likely to suffer injuries in the high cervical region, particularly in the atlantoaxial region. The cervical spine is at greater risk in the infant and younger child because of the relatively weak and flexible neck muscles that support a proportionally large and heavy head, with the atlantooccipital area acting as a pivot point. Atlantooccipital dislocations are major neurologic injuries, leaving children neurologically devastated but not necessarily dead.
As with brain injury, spinal cord injury occurs in two phases. The primary insult results from biomechanical forces and bony fragments directly impacting the spinal cord. The secondary insult results from the pathologic sequelae of the primary insult: edema and ischemia due to cortical compression, hypotension, or hypoxia. Inappropriate manipulation of a child with an unstable fracture can exacerbate primary and secondary injuries. Anesthesiologists who provide care for a child with a potential cervical spine injury should be aware that spinal cord injuries in children commonly occur without actual evidence of spinal bone fractures on plain cervical radiographs. These injuries are known as spinal cord injuries without radiologic abnormality (SCIWORA).141 Injuries to the cervical spine in particular are often difficult to recognize but may be identified by odontoid displacement or prevertebral swelling on radiographs. As a result, CT is frequently indicated when a spinal injury is initially suspected in a child with trauma. After a child with a potential spinal injury is determined to be medically stable, these studies should be obtained as soon as possible. The child’s airway and cardiorespiratory function must be continuously and closely monitored until a spinal cord injury can be ruled out. Sometimes, as with brain injury, there can be a delay in the onset of neurologic deficits with SCIWORA injuries.142
Prompt airway management is essential to avoid hypoxia, ensure adequate respiratory mechanics, preserve neural function, and prevent extension of spinal injury (see Figs. 38-5 through 38-7). The head and neck must be immediately immobilized; restraint of the extremities may also be required. Various tracheal tubes and laryngoscope blades should be available, as well as equipment and personnel for an emergency tracheostomy. Insertion of a laryngeal mask airway may be lifesaving until a more secure airway can be achieved with fiberoptic or other means.143–148 Small fiberoptic bronchoscopes (2.2-mm diameter) can fit through infant-sized tracheal tubes. Retrograde intubation using a guidewire introduced through the cricothyroid membrane may be useful in older children or adolescents (see Chapter 12). However, an unstable infant or child whose airway cannot be secured by conventional means is probably best managed by an emergency tracheostomy. As a temporizing measure, a cricothyroidotomy can be performed (see Figs. 12-25 through 12-27).149 This permits oxygenation (although inadequate ventilation) until personnel and equipment for tracheostomy are assembled. An emergent surgical airway can be extremely difficult to perform on a small child or infant, even by experienced and skilled hands.
Although there are few data for adults and children, corticosteroids are often administered with spinal injuries as soon as possible after the initial trauma in the hope of reducing the neurologic injury. The most commonly used drug is methylprednisolone; 30 mg/kg is administered over the first 15 minutes, followed by an infusion of 5.4 mg/kg/hr for the next 23 hours.150,151 Methylprednisolone is thought to be effective through multiple mechanisms, including improved spinal blood flow, inhibition of the arachidonic acid cascade, and modulation of the local immune response.152 Some evidence suggests that GM1 ganglioside, with or without methylprednisolone, may be advantageous in decreasing demyelination and promoting neurologic recovery if administered soon after a spinal injury.153–159
If the spinal cord injury is more than 24 hours old, succinylcholine should be avoided because it can result in massive hyperkalemia.160 Physiologic changes may result from autonomic hyperreflexia, which frequently develops after cervical or high thoracic spinal lesions. Autonomic hyperreflexia can produce severe and life-threatening vasomotor instability with hypertension and arrhythmias.161,162
Craniotomy
Tumors
Brain tumors are the most common solid tumors in children, exceeded only by the leukemias as the most common pediatric malignancy.163 Between 1500 and 2000 new brain tumors are diagnosed annually in children in the United States. Unlike those in adults, most brain tumors in children are infratentorial in the posterior fossa. They include medulloblastomas, cerebellar astrocytomas, brainstem gliomas, and ependymomas of the fourth ventricle. Because posterior fossa tumors usually obstruct CSF flow, increased ICP occurs early. Presenting signs and symptoms include early morning vomiting and irritability or lethargy. Cranial nerve palsies and ataxia are also common findings, with respiratory and cardiac irregularities usually occurring late. Sedation or general anesthesia may be required for radiologic evaluation or radiation therapy.
Surgical resection of a posterior fossa tumor presents a number of anesthetic challenges. Children are usually positioned prone, although the lateral or sitting positions are used by some neurosurgeons. In any case, the head is flexed, and the position and patency of the tracheal tube must be meticulously ensured. In the event that the tracheal tube does become dislodged when the child is in a head holder and prone, successful emergent airway management has been described using a laryngeal mask airway.164
Postoperative diabetes insipidus is marked by a sudden large increase in dilute urine output associated with an increasing serum sodium concentration and osmolality. Protocols have been developed to guide intraoperative and postoperative management of diabetes insipidus (see Chapter 8).127 Return of antidiuretic hormone activity a few days postoperatively may cause a marked decrease in urinary output, water intoxication, seizures, and cerebral edema if it is not recognized and fluid administration is not adjusted appropriately.
Approximately 25% of intracranial tumors in children involve the cerebral hemispheres. They are primarily astrocytomas, oligodendrogliomas, ependymomas, and glioblastomas. Neurologic symptoms are more likely to include a seizure disorder or focal deficits. Succinylcholine should be avoided if motor weakness is present because it can cause sudden severe hyperkalemia. Nondepolarizing NMBDs and opioids may be metabolized more rapidly than usual in children who are receiving chronic anticonvulsants. Choroid plexus papillomas are rare but occur most often in children younger than 3 years of age. They usually arise from the choroid plexus of the lateral ventricle and produce early hydrocephalus as a result of increased production of CSF and obstruction of CSF flow. Hydrocephalus usually resolves with surgical resection. When lesions lie near the motor or sensory strip, a special type of somatosensory evoked potential monitoring called phase reversal may be used to delineate the locations.165 If cortical stimulation is planned to help identify motor areas, NMBDs must be permitted to wear off and the anesthetic technique adjusted to achieve immobilization without paralysis.
Stereotactic biopsies or craniotomies present special concerns regarding airway accessibility. Newer head frames have adjustable anterior positions so that the airway is readily accessible (E-Fig. 24-1). They are especially useful for stereotactic neurosurgery. It is more comfortable and less distressing for the child to be anesthetized before the head frame is applied, even though this means the anesthesiologist must induce anesthesia in the radiology suite and then transport the child from the CT scanner to the operating room. The wrench that is used to apply and remove the head frame should be taped to the frame at all times so that it is always readily available if emergent removal of the head frame becomes necessary (e.g., during transport).
Vascular Anomalies
Arteriovenous Malformations
Arteriovenous malformations consist of large arterial feeding vessels, dilated communicating vessels, and large draining veins carrying arterialized blood. Large malformations, especially those involving the posterior cerebral artery and vein of Galen, may manifest as congestive heart failure (i.e., high-output heart failure, often with pulmonary hypertension) in the neonate. The prognosis for these types of arteriovenous malformations is quite poor. Saccular dilation of the vein of Galen may manifest later in infancy or childhood as hydrocephalus due to obstruction of the aqueduct of Sylvius. Malformations not large enough to produce congestive heart failure usually remain clinically silent unless they cause seizures or a stroke or until the acute rupture of a communicating vessel results in subarachnoid or intracerebral hemorrhage.166 Intracranial hemorrhages are the most common presentation in this population, with an associated mortality rate of 25%.
Aneurysms
Intracranial aneurysms most often result from a congenital malformation in an arterial wall. Children with coarctation of the aorta or polycystic kidney disease have an increased incidence of these aneurysms. They usually remain asymptomatic during childhood; most ruptures that occur in childhood are fatal. Symptoms of subarachnoid or intracerebral hemorrhage frequently appear suddenly in a previously healthy young adult. When technically feasible, surgical ligation or clipping constitutes the treatment of choice.167
Controlled hypotension may be valuable in some situations for brief periods to reduce tension in the abnormal blood vessels and improve the safety of surgical manipulation.168 It is not clear, however, whether the benefits of controlled hypotension are worth the risks, especially in small children (see Chapter 10). Controlled hypotension should not be used in children with increased ICP because of the risk of decreasing CPP, with resulting ischemia and further increased ICP. Although the absolute limits of acceptable hypotension are unknown, a mean blood pressure greater than 40 mm Hg for infants or 50 mm Hg for older children appears to be safe; teenagers should have a target mean arterial pressure no less than 55 mm Hg. At the conclusion of the procedure, the blood pressure is returned to normal, but before closing the dura, the operative site should be inspected for bleeding.
Moyamoya Disease
Moyamoya disease is an anomaly that results in progressive and life-threatening occlusion of intracranial vessels, primarily the internal carotid arteries near the circle of Willis.169 An abnormal vascular network of collaterals develops at the base of the brain, and the appearance of these many, small vessels on angiography was originally described by the Japanese name moyamoya, which roughly translates as “puff of smoke.” The congenital form of the disease can involve the systemic vasculature, including pulmonary, coronary, and renal vessels; affected renal arteries are the most commonly identified angiographic lesion. The acquired variety (i.e., moyamoya syndrome) may be associated with meningitis, neurofibromatosis, chronic inflammation, connective tissue diseases, certain hematologic disorders, Down syndrome, or prior intracranial radiation. Some children with neurologic symptoms from sickle cell disease may also have moyamoya.170 Moyamoya disease appears to be more common among children of Japanese ancestry. Associated intracranial aneurysms are rare in children but may occur in more than 10% of affected adult patients. Abnormal electrocardiographic findings have been described with the syndrome in adults.
Moyamoya disease usually manifests as transient ischemic attacks progressing to strokes and fixed neurologic deficits in children. The attacks may be precipitated by hyperventilation.171 The morbidity and mortality rates are high if the condition is left untreated. Medical management consists of antiplatelet therapy, such as aspirin, or calcium channel blockers. The most common surgical operation for correction in children is pial synangiosis, which involves suturing a scalp artery (usually the superficial temporal artery) directly onto the pial surface of the brain to enhance angiogenesis (Fig. 24-9).172
Careful and continuous monitoring of end-tidal CO2 partial pressure is essential in anesthesia management.173 Children with moyamoya disease have reduced hemispheric blood flow bilaterally, and hyperventilation may further reduce regional blood flow and cause significant EEG and neurologic changes.174 Normocapnia must be maintained throughout all phases of the procedure, including induction of anesthesia. Adequate hydration and maintenance of baseline blood pressure are indispensable. Most of these children have an intravenous catheter inserted the night before surgery and are given 1.5 times the amount of maintenance fluids to avoid dehydration during the perioperative period. EEG monitoring during these procedures can detect and help to treat ischemia that appears to be a result of cerebral vasoconstriction in response to direct surgical manipulation of the brain.175 Normothermia is maintained, particularly at the end of the procedure, to avoid postoperative shivering and an exaggerated stress response. As with most neurosurgical procedures, a smooth extubation without hypertension or crying is desirable. Although scant literature exists regarding intraoperative and postoperative complications during moyamoya surgery, it appears that most complications (e.g., strokes) occur postoperatively and are associated with dehydration and crying (i.e., hyperventilation) episodes.176
Seizure Surgery
Epilepsy is one of the most common neurologic disorders of childhood. Despite the development of new drugs and regimens, the prevalence of pharmacologically intractable seizures remains high. Advances in neuroimaging and EEG monitoring provide epileptologists anatomic targets that mediate some medically intractable seizure disorders. Advances in pediatric neurosurgery have exploited these technologies and dramatically improved the outcomes for infants and children.177
Some practitioners perform the entire procedure, including line placement, infiltration of local anesthetic, skull and dural opening, and resection, with the child completely awake or with minimal sedation. This particular approach requires an extremely motivated child. A variation on this technique uses short-acting sedatives and analgesics, such as propofol and fentanyl, titrated to induce unconsciousness but maintain spontaneous ventilation for instillation of local anesthetics, insertion of monitoring catheters, placement of head pins, and skull opening.178 Subsequently, children can be allowed to awaken during surgical resection. They can then have sedatives and opioids reinstituted for the craniotomy closure.
Younger children (up to 12 years of age) or uncooperative children of any age do not tolerate this approach and require general anesthesia throughout. In these circumstances, intraoperative electrophysiologic studies, such as somatosensory evoked potentials, EEG, and motor stimulation, may be used to help localize and determine the function of the site of planned resection. If EEG studies are to be performed, the anesthetic technique should be adjusted to maximize EEG signals. If direct cortical motor stimulation is planned, NMBDs must be permitted to wear off. Occasionally, a seizure focus is difficult to identify intraoperatively. In these situations, hyperventilation or methohexital (in small doses, 0.25 to 0.5 mg/kg) may be helpful in lowering the seizure threshold and producing EEG seizure activity.179,180
In some children, the site of origin of generalized seizures is difficult to determine. When this occurs, evaluation with intracranial EEG monitoring (“grids and strips”) may be accomplished with direct electrocorticography (E-Fig. 24-2). The leads are placed on the surface of the cortex after a craniotomy performed under general anesthesia. Intraoperative EEG monitoring is limited during these procedures to ensuring that all leads are functional; monitoring for seizures takes place over the next several days to identify a focus that is amenable to resection. These children need to be observed carefully in the postoperative period because complications can develop from having intracranial electrodes in place. Because air frequently persists in the skull for up to 3 weeks after a craniotomy,86 these children should not have nitrous oxide administered for a subsequent procedure (e.g., to resect a seizure focus, to remove the electrocorticography leads) until their dura has been opened to prevent the development of tension pneumocephalus.
When a focal resection is not possible, a lobectomy or corpus callosotomy may be attempted. However, children undergoing the latter procedure are often somnolent for the first few postoperative days, especially if a complete callosotomy is performed. This also occurs in children who have undergone insertion of multiple subdural grids and strips. Occasionally, small children undergo a hemispherectomy because their seizures are attributed to an abnormal hemisphere that is already severely dysfunctional, as when affected by hemiparesis. These can be challenging cases for the anesthesiologist because much blood can be lost (from one half to multiples of the estimated blood volume).181 This procedure is usually performed when children are very young to permit the other hemisphere to take over the function of both sides. Large-bore intravenous access is necessary in these cases to facilitate rapid replacement of blood, crystalloid solutions, and medications. Arterial pressure monitoring is routine, and many practitioners also use CVP monitoring.
An advance in the treatment of epilepsy has been the development of the vagal nerve stimulator. Although its exact mechanism of action is not well understood, it appears to inhibit seizure activity at brainstem or cortical levels.182,183 It is becoming a popular form of treatment because it has shown benefit with minimal side effects in many children who are disabled by intractable seizures. Large, randomized trials are being conducted to determine the overall efficacy of this treatment. There are few published series of vagal nerve stimulation in children, but it is estimated that there is a 60% to 70% improvement in seizure control, with the best results achieved in those with drop attacks.184,185
The vagal nerve stimulator is a programmable device that is similar to a cardiac pacemaker placed subcutaneously under the left anterior chest wall. Bipolar platinum stimulating electrode coils, which are implanted around the left vagus nerve, are connected to the generator by subcutaneously tunneled wires. The device automatically activates for up to 30 seconds every 5 minutes. Although stimulation of the vagal nerve in this manner may affect vocal cord function, sudden bradycardia or other side effects are uncommon.186 When children with vagal nerve stimulators return for subsequent operations, it may be appropriate to deactivate the stimulator while the child is under general anesthesia to prevent repetitive vocal cord motion.
Hydrocephalus
Hydrocephalus is a condition involving a mismatch of CSF production and absorption, resulting in an increased intracranial CSF volume. It can be caused by a variety of pathologic processes, including arachnoid cysts (E-Fig. 24-3). Except for rare instances of excess CSF production, such as in choroid plexus papillomas, most cases of hydrocephalus result from some type of obstruction or an inability to absorb CSF appropriately. Commonly, this is a result of neonatal intraventricular or subarachnoid hemorrhage, congenital problems (e.g., aqueductal stenosis), trauma, infection, or tumors, especially those in the posterior fossa. Hydrocephalus can be classified as nonobstructive/communicating or obstructive/noncommunicating based on the ability of CSF to flow around the spinal cord in its usual manner.
Unless the cause of the hydrocephalus can be definitively treated, treatment usually involves surgical placement of an extracranial shunt. Most shunts transport CSF from the lateral ventricles to the peritoneal cavity (i.e., ventriculoperitoneal shunts). The distal end of the shunt occasionally must be placed in the right atrium or pleural cavity, usually because of problems with the ability of the peritoneal cavity to absorb CSF. Newer shunt systems with programmable valves are being tried to reduce the need for shunt revisions.187
The use of a percutaneous flexible neuroendoscope through a burrhole in the skull has provided an alternative to extracranial shunt placement.188,189 During these procedures, a ventriculostomy may be made to bypass an obstruction (e.g., aqueductal stenosis) by forming a communicating hole from one area of CSF flow to another using a blunt probe inserted through the neuroendoscope (Video 24-1). Common locations for a ventriculostomy are through the septum pellucidum (allowing lateral ventricles to communicate) or through the floor of the third ventricle into the adjacent CSF cisterns. Complications such as damage to the basilar artery or its branches or neural injuries can be life-threatening when they occur, and the anesthesiologist should be prepared for an emergency craniotomy during these procedures. Hemodynamic instability may occur intraoperatively if excessive cold irrigating solutions or large volumes are infused through the endoscope.
The anesthetic plan for a child with hydrocephalus should be directed at controlling ICP and relieving the obstruction as soon as possible. Children with an increased ICP are at risk for vomiting and pulmonary aspiration. Rapid-sequence induction and tracheal intubation should be performed. Ketamine is avoided in these children because of its potential to cause sudden massive intracranial hypertension.190 Despite this concern, there is some evidence that ketamine may ameliorate the increased intracranial pressure in children.191,192 In infants, hydrocephalus often produces large, dilated scalp veins, and they can be used for induction of anesthesia if necessary. If intravenous access cannot be established, induction with sevoflurane and gentle cricoid pressure may be an alternative, although less desirable, method of induction.193 This method results in venodilation and usually facilitates establishment of intravenous access. After an intravenous catheter is inserted, the child may be paralyzed, the lungs ventilated, the trachea intubated, and the inhalational agent decreased or discontinued. The possibility of VAE during placement of the distal end of a ventriculoatrial shunt should always be considered. Postoperatively, children should be observed carefully because an altered mental status and recent peritoneal incision place them at increased risk for pulmonary aspiration after feeding begins. Analgesia may be provided with a variety of easily performed blocks of the head (see Figs. 41-15 and 41-16).
Anesthesiologists should be aware of the condition known as slit ventricle syndrome (Fig. 24-10). This situation develops in 5% to 10% of children with CSF shunts and is associated with overdrainage of CSF and small, slitlike, lateral ventricular spaces. Children with this condition do not have the usual amount of intracranial CSF to compensate for alterations in brain or intracranial blood volume. Special attention should be paid when CT scans identify this condition. It is probably safest to avoid the administration of excess or hypotonic intravenous solutions in these situations in the intraoperative and postoperative periods to minimize the potential for brain swelling. Some of these children cannot accommodate to situations that otherwise healthy children can easily tolerate. Episodes of postoperative cerebral herniation have been reported after uneventful surgical procedures.45
Congenital Anomalies
Congenital CNS anomalies typically occur as midline defects. This dysraphism may occur anywhere along the neural axis, involving the head (i.e., encephalocele) or spine (i.e., meningomyelocele) (Fig. 24-11, A). The defect may be relatively minor and affect only superficial bony and membranous structures, or it may include a large segment of malformed neural tissue.
Encephalocele
Encephaloceles can occur anywhere from the occiput to the frontal area. They can even appear to be nasal polyps if they protrude through the cribriform plate. They are rarely filled with so much CSF that the defect can be almost as large as the head itself (see Fig. 24-11, B). Large defects may present challenges to tracheal intubation. Blood loss can be severe, especially if venous sinuses are involved. Adequate intravenous access should be ensured and blood products readily available. If hemodynamic instability is anticipated, an arterial catheter is indicated.
Myelodysplasia
A major anesthesia consideration is positioning the neonate for induction at surgery. In most cases, tracheal intubation can be performed with the infant in the supine position and the uninvolved portion of the child’s back supported with towels (or a donut ring) so there is no direct pressure on the meningomyelocele. For very large defects, it is occasionally necessary to place the infant in the left lateral decubitus position for induction and tracheal intubation. Succinylcholine is rarely needed for tracheal intubation, although it is not associated with hyperkalemia because the defect develops early in gestation and is not associated with muscle denervation.194 Airway management, mask fit, and intubation may be difficult in infants with massive hydrocephalus or very large defects. In such cases, awake intubation after preoxygenation and administration of atropine occasionally may be the safest alternative. Blood loss may be considerable during repair of a larger defect when skin is undermined to cover the defect.
Children with myelodysplasia are at high risk for latex sensitivity and possibly anaphylaxis.40 This likely results from repeated exposure to latex products encountered during frequent bladder catheterizations and multiple (usually more than five) surgical procedures, during which latex gloves have been in contact with large mucosal surfaces. These children should be managed in a latex-free environment from birth to minimize the chances for sensitization.195 Latex allergy should be suspected if signs and symptoms of anaphylaxis develop during surgery. Suspected anaphylaxis should be treated with intravenous epinephrine in a dose of 1 to 10 µg/kg, as required. Many hospitals have replaced most or all of their latex-containing supplies with nonlatex alternatives; this has resulted in complete elimination of latex anaphylactic reactions during anesthesia in some institutions.196 Children who develop latex allergy exhibit cross-reactivity with some antibiotics197,198 and foods, especially tropical fruits such as avocados, kiwis, and bananas.
Postoperatively, respiratory status should be carefully assessed. Pulse oximetry is valuable during recovery from anesthesia because breathing difficulties may occur after a tight skin closure and the ventilatory responses to hypoxia and hypercarbia may be diminished or absent when a Chiari malformation coexists.199 Intrauterine surgery has been advocated as a way of diminishing the degree of damage caused by myelodysplasia.200–202
Chiari Malformations
There are several types of Chiari malformations (Table 24-3). The Arnold-Chiari malformation (type II) usually coexists in children with myelodysplasia. This defect consists of a bony abnormality in the posterior fossa and upper cervical spine with caudal displacement of the cerebellar vermis, fourth ventricle, and lower brainstem below the plane of the foramen magnum. Medullary cervical cord compression can occur (Figs. 24-12 and 24-13). Vocal cord paralysis with stridor and respiratory distress, apnea, abnormal swallowing and pulmonary aspiration, opisthotonos, and cranial nerve deficits may be associated with the Arnold-Chiari malformation and usually manifests during infancy. Children with vocal cord paralysis or a diminished gag reflex may require tracheostomy and gastrostomy to secure the airway and to minimize chronic aspiration. Children of any age may have abnormal responses to hypoxia and hypercarbia because of cranial nerve and brainstem dysfunction.199,203 Extreme head flexion may cause brainstem compression in otherwise asymptomatic children.
Type I | Caudal displacement of cerebellar tonsils below the plane of the foramen magnum |
Type II (Arnold-Chiari; associated with myelomeningocele) | Caudal displacement of the cerebellar vermis, fourth ventricle, and lower brainstem below the plane of the foramen magnum Dysplastic brainstem with characteristic kink, elongation of the fourth ventricle, beaking of the quadrigeminal plate, hypoplastic tentorium with small posterior fossa, polymicrogyria, enlargement of the massa intermedia |
Type III | Caudal displacement of the cerebellum and brainstem into a high cervical meningocele |
Type IV | Cerebellar hypoplasia |
Type I Chiari malformations can occur in healthy children without myelodysplasia. These defects also involve caudal displacement of the cerebellar tonsils below the foramen magnum, but children usually have much milder symptoms, sometimes manifesting only as headache or neck pain.204 Surgical treatment usually involves a decompressive suboccipital craniectomy with cervical laminectomies.
Neuroradiologic Procedures
Many neuroradiologic procedures are performed in children. Anesthetic considerations for neurodiagnostic procedures (e.g., CT, MRI) are discussed elsewhere in this book (see Chapters 45 and 47), but certain therapeutic neuroradiologic procedures are addressed here.
To improve intraoperative navigation during intracranial procedures, the concept of intraoperative MRI was introduced in the mid-1990s. The technology has advanced to include a variety of operating room suite designs that encompass MRI machines (E-Fig. 24-4). These procedures present special challenges for neurosurgeons and anesthesiologists.205–209 As with anesthesia for diagnostic MRI scans, special monitors, infusion pumps, and an MRI-safe or MRI-conditional anesthesia machine are required. It is challenging to do surgical procedures in this environment, especially because these procedures may take many hours, they may be associated with significant blood loss, and access to the child is severely limited. Some monitoring equipment found in conventional operating rooms (e.g., precordial Doppler ultrasonography, core temperature probes, fluid warmers) is not MRI safe or conditional. Nevertheless, numerous neurosurgical procedures have been safely performed in children in these MRI operating rooms, and the equipment for these procedures is rapidly evolving.
Coles JP, Fryer TD, Coleman MR, et al. Hyperventilation following head injury: effect on ischemic burden and cerebral oxidative metabolism. Crit Care Med. 2007;35:568–578.
Cox RG, Levy R, Hamilton MG, et al. Anesthesia can be safely provided for children in a high-field intraoperative magnetic resonance imaging environment. Paediatr Anaesh. 2011;21:454–458.
DiMaggio C, Sun LS, Li G. Early childhood exposure to anesthesia and risk of developmental and behavioral disorders in a sibling birth cohort. Anesth Analg. 2011;113:1143–1151.
Jevtovic-Todorovic V, Hartman RE, Izumi Y, et al. Early exposure to common anesthetic agents causes widespread neurodegeneration in the developing rat brain and persistent learning deficits. J Neurosci. 2003;23:876–882.
Lassen NA, Christensen MS. Physiology of cerebral blood flow. Br J Anaesth. 1976;48:719–734.
This review article discusses the various physiologic mechanisms of control of cerebral blood flow.
Pollack IF. Brain tumors in children. N Engl J Med. 1994;331:1500–1507.
Reasoner DK, Todd MM, Scamman FL, Warner DS. The incidence of pneumocephalus after supratentorial craniotomy: observations on the disappearance of intracranial air. Anesthesiology. 1994;80:1008–1012.
1 Shapiro HM. Intracranial hypertension: therapeutic and anesthetic considerations. Anesthesiology. 1975;43:445–471.
2 Arieff AI, Ayus JC, Fraser CL. Hyponatraemia and death or permanent brain damage in healthy children. BMJ. 1992;304:1218–1222.
3 Ayus JC, Arieff AI. Pathogenesis and prevention of hyponatremic encephalopathy. Endocrinol Metab Clin North Am. 1993;22:425–446.
4 Trachtman H. Cell volume regulation—a review of cerebral adaptive mechanisms and implications for clinical treatment of osmolal disturbances: I. Pediatr Nephrol. 1991;5:743–750.
5 Minns RA, Brown JK, Engleman HM. CSF production rate: “real time” estimation. Z Kinderchir. 1987;42:36–40.
6 Blomquist HK, Sundin S, Ekstedt J. Cerebrospinal fluid hydrodynamic studies in children. J Neurol Neurosurg Psychiatry. 1986;49:536–548.
7 Rubin RC, Henderson ES, Ommaya AK, Walker MD, Rall DP. The production of cerebrospinal fluid in man and its modification by acetazolamide. J Neurosurg. 1966;25:430–436.
8 Clasen RA, Pandolfi S, Casey DJ. Furosemide and pentobarbital in cryogenic cerebral injury and edema. Neurology. 1974;24:642–648.
9 Bruce DA, Berman WA, Schut L. Cerebrospinal fluid pressure monitoring in children: physiology, pathology and clinical usefulness. Adv Pediatr. 1977;24:233–290.
10 Jones TH, Morawetz RB, Crowell RM, et al. Thresholds of focal cerebral ischemia in awake monkeys. J Neurosurg. 1981;54:773–782.
11 Marshall LF, Smith RW, Shapiro HM. The influence of diurnal rhythms in patients with intracranial hypertension: implications for management. Neurosurgery. 1978;2:100–102.
12 McDowall DG. Monitoring the brain. Anesthesiology. 1976;45:117–134.
13 Chaves-Carballo E, Gomez MR, Sharbrough FW. Encephalopathy and fatty infiltration of the viscera (Reye-Johnson syndrome): a 17-year experience. Mayo Clin Proc. 1975;50:209–215.
14 Hanlon K. Description and uses of intracranial pressure monitoring. Heart Lung. 1976;5:277–282.
15 Lundberg N, Troupp H, Lorin H. Continuous recording of the ventricular-fluid pressure in patients with severe acute traumatic brain injury: a preliminary report. J Neurosurg. 1965;22:581–590.
16 Coroneos NJ, McDowall DG, Pickerodt VW, Keaney NP, Gibson RM. A comparison of intracranial extradural pressure with subarachnoid pressure. Br J Anaesth. 1971;43:1198.
17 Ream AK, Silverberg GD, Corbin SD, Schmidt EV, Fyer TB. Epidural measurement of intracranial pressure. Neurosurgery. 1979;5:36–43.
18 Levin AB, Kahn AR, Bahr DE. Epidural intracranial pressure monitoring: a new system. Med Instrum. 1983;17:293–296.
19 Di Rocco C, McLone DG, Shimoji T, Raimondi AJ. Continuous intraventricular cerebrospinal fluid pressure recording in hydrocephalic children during wakefulness and sleep. J Neurosurg. 1975;42:683–689.
20 Lassen NA, Christensen MS. Physiology of cerebral blood flow. Br J Anaesth. 1976;48:719–734.
21 Lassen NA, Hoedt-Rasmussen K. Human cerebral blood flow measured by two inert gas techniques: comparison of the Kety-Schmidt method and the intra-arterial injection method. Circ Res. 1966;19:681–694.
22 Kety SS, Schmidt CF. The nitrous oxide method for the quantitative determination of cerebral blood flow in man: theory, procedure, and normal values. J Clin Invest. 1948;27:476–483.
23 Kennedy C, Sokoloff L. An adaptation of the nitrous oxide method to the study of cerebral circulation in children: normal values for cerebral blood flow and cerebral metabolic rate in childhood. J Clin Invest. 1957;36:1130–1137.
24 Mehta S, Kalsi HK, Nain CK, Menkes JH. Energy metabolism of brain in human protein-calorie malnutrition. Pediatr Res. 1977;11:290–293.
25 Cross KW, Dear PR, Hathorn MK, et al. An estimation of intracranial blood flow in the new-born infant. J Physiol (Lond). 1979;289:329–345.
26 Younkin DP, Reivich M, Jaggi J, et al. Noninvasive method of estimating human newborn regional cerebral blood flow. J Cereb Blood Flow Metab. 1982;2:415–420.
27 Milligan DW. Cerebral blood flow and sleep state in the normal newborn infant. Early Hum Dev. 1979;3:321–328.
28 Settergren G, Lindblad BS, Persson B. Cerebral blood flow and exchange of oxygen, glucose, ketone bodies, lactate, pyruvate and amino acids in infants. Acta Paediatr Scand. 1976;65:343–353.
29 Luerssen TG. Intracranial pressure: current status in monitoring and management. Semin Pediatr Neurol. 1997;4:146–155.
30 Hernandez MJ, Brennan RW, Bowman GS. Autoregulation of cerebral blood flow in the newborn dog. Brain Res. 1980;184:199–202.
31 Lou HC, Lassen NA, Friis-Hansen B. Impaired autoregulation of cerebral blood flow in the distressed newborn infant. J Pediatr. 1979;94:118–121.
32 Cohen PJ, Alexander SC, Smith TC, Reivich M, Wollman H. Effects of hypoxia and normocarbia on cerebral blood flow and metabolism in conscious man. J Appl Physiol. 1967;23:183–189.
33 Kety SS, Schmidt CF. The effects of altered arterial tensions of carbon dioxide and oxygen on cerebral blood flow and cerebral oxygen consumption in normal young men. J Clin Invest. 1948;27:484–492.
34 Rahilly PM. Effects of 2% carbon dioxide, 0.5% carbon dioxide, and 100% oxygen on cranial blood flow of the human neonate. Pediatrics. 1980;66:685–689.
35 Rogers MC, Nugent SK, Traystman RJ. Control of cerebral circulation in the neonate and infant. Crit Care Med. 1980;8:570–574.
36 Coles JP, Fryer TD, Coleman MR, et al. Hyperventilation following head injury: effect on ischemic burden and cerebral oxidative metabolism. Crit Care Med. 2007;35:568–578.
37 Marion DW, Firlik A, McLaughlin MR. Hyperventilation therapy for severe traumatic brain injury. New Horiz. 1995;3:439–447.
38 Skippen P, Seear M, Poskitt K, et al. Effect of hyperventilation on regional cerebral blood flow in head-injured children. Crit Care Med. 1997;25:1402–1409.
39 Lassen NA. Control of cerebral circulation in health and disease. Circ Res. 1974;34:749–760.
40 Holzman RS. Clinical management of latex-allergic children. Anesth Analg. 1997;85:529–533.
41 Tempelhoff R, Modica PA, Spitznagel ELJ. Anticonvulsant therapy increases fentanyl requirements during anaesthesia for craniotomy. Can J Anaesth. 1990;37:327–332.
42 Alloul K, Whalley DG, Shutway F, Ebrahim Z, Varin F. Pharmacokinetic origin of carbamazepine-induced resistance to vecuronium neuromuscular blockade in anesthetized patients. Anesthesiology. 1996;84:330–339.
43 Soriano SG, Kaus SJ, Sullivan LJ, Martyn JA. Onset and duration of action of rocuronium in children receiving chronic anticonvulsant therapy. Paediatr Anaesth. 2000;10:133–136.
44 Melton AT, Antognini JF, Gronert GA. Prolonged duration of succinylcholine in patients receiving anticonvulsants: evidence for mild upregulation of acetylcholine receptors? Can J Anaesth. 1993;40:939–942.
45 Eldredge EA, Rockoff MA, Medlock MD, Scott RM, Millis MB. Postoperative cerebral edema occurring in children with slit ventricles. Pediatrics. 1997;99:625–630.
46 Shapiro HM, Galindo A, Wyte SR, Harris AB. Rapid intraoperative reduction of intracranial pressure with thiopentone. Br J Anaesth. 1973;45:1057–1062.
47 Modica PA, Tempelhoff R. Intracranial pressure during induction of anaesthesia and tracheal intubation with etomidate-induced EEG burst suppression. Can J Anaesth. 1992;39:236–241.
48 Tulleken CA, van Dieren A, Jonkman J, Kalenda Z. Clinical and experimental experience with etomidate as a brain protective agent. J Cereb Blood Flow Metab. 1982;2:S92–S97.
49 Milde LN, Milde JH. Preservation of cerebral metabolites by etomidate during incomplete cerebral ischemia in dogs. Anesthesiology. 1986;65:272–277.
50 Lockhart CH, Jenkins JJ. Ketamine-induced apnea in patients with increased intracranial pressure. Anesthesiology. 1972;37:92–93.
51 Crumrine RS, Nulsen FE, Weiss MH. Alterations in ventricular fluid pressure during ketamine anesthesia in hydrocephalic children. Anesthesiology. 1975;42:758–761.
52 Abou-Madi MN, Keszler H, Yacoub JM. Cardiovascular reactions to laryngoscopy and tracheal intubation following small and large intravenous doses of lidocaine. Can Anaesth Soc J. 1977;24:12–19.
53 Baker KZ. Desflurane and sevoflurane are valuable additions to the practice of neuroanesthesiology: pro. J Neurosurg Anesthesiol. 1997;9:66–68.
54 Tempelhoff R. The new inhalational anesthetics desflurane and sevoflurane are valuable additions to the practice of neuroanesthesia: con. J Neurosurg Anesthesiol. 1997;9:69–71.
55 Scheller MS, Tateishi A, Drummond JC, Zornow MH. The effects of sevoflurane on cerebral blood flow, cerebral metabolic rate for oxygen, intracranial pressure, and the electroencephalogram are similar to those of isoflurane in the rabbit. Anesthesiology. 1988;68:548–551.
56 Holzman RS, van der Velde ME, Kaus SJ, et al. Sevoflurane depresses myocardial contractility less than halothane during induction of anesthesia in children. Anesthesiology. 1996;85:1260–1267.
57 Jääskeläinen SK, Kaisti K, Suni L, Hinka S, Scheinin H. Sevoflurane is epileptogenic in healthy subjects at surgical levels of anesthesia. Neurology. 2003;61:1073–1078.
58 Watt S, Pickhardt D, Lerman J, et al. Telescoping tracheal tubes into catheters minimizes epistaxis during nasotracheal intubation in children. Anesthesiology. 2007;106:238–242.
59 Liu LM, DeCook TH, Goudsouzian NG, Ryan JF, Liu PL. Dose response to intramuscular succinylcholine in children. Anesthesiology. 1981;55:599–602.
60 Kovarik WD, Mayberg TS, Lam AM, Mathisen TL, Winn HR. Succinylcholine does not change intracranial pressure, cerebral blood flow velocity, or the electroencephalogram in patients with neurologic injury. Anesth Analg. 1994;78:469–473.
61 Minton MD, Grosslight K, Stirt JA, Bedford RF. Increases in intracranial pressure from succinylcholine: prevention by prior nondepolarizing blockade. Anesthesiology. 1986;65:165–169.
62 Cooperman LH. Succinylcholine-induced hyperkalemia in neuromuscular disease. JAMA. 1970;213:1867–1871.
63 Mazurek AJ, Rae B, Hann S, et al. Rocuronium versus succinylcholine: are they equally effective during rapid-sequence induction of anesthesia? Anesth Analg. 1998;87:1259–1262.
64 Todres ID, deBros F, Kramer SS, Moylan FM, Shannon DC. Endotracheal tube displacement in the newborn infant. J Pediatr. 1976;89:126–127.
65 Levy ML, Granville RC, Hart D, Meltzer H. Deep venous thrombosis in children and adolescents. J Neurosurg. 2004;101:32–37.
66 Morgan J. Perioperative venous thrombosis in children: is it time for primary prophylaxis? Pediatr Anesth. 2007;17:99–101.
67 Meridy HW, Creighton RE, Humphreys RP. Complications during neurosurgery in the prone position in children. Can Anaesth Soc J. 1974;21:445–453.
68 Lee LA, Roth S, Posner KL, et al. The American Society of Anesthesiologists Postoperative Visual Field Loss Registry: analysis of 93 spine cases with postoperative visual loss. Anesthesiology. 2006;105:652–659.
69 Practice advisory for perioperative visual loss associated with spine surgery: a report by the American Society of Anesthesiologists Task force on perioperative blindness. Anesthesiology. 2006;104:1319–1328.
70 Lorenc ZP, Ivy E, Aston SJ. Neurosensory preservation in endoscopic forehead plasty. Aesthetic Plast Surg. 1995;19:411–413.
71 Pinosky ML, Fishman RL, Reeves ST, et al. The effect of bupivacaine skull block on the hemodynamic response to craniotomy. Anesth Analg. 1996;83:1256–1261.
72 Becser N, Bovim G, Sjaastad O. Extracranial nerves in the posterior part of the head: anatomic variations and their possible clinical significance. Spine. 1998;23:1435–1441.
73 Scheller MS, Nakakimura K, Fleischer JE, Zornow MH. Cerebral effects of sevoflurane in the dog: comparison with isoflurane and enflurane. Br J Anaesth. 1990;65:388–392.
74 Neigh JL, Garman JK, Harp JR. The electroencephalographic pattern during anesthesia with ethrane: effects of depth of anesthesia, Paco2, and nitrous oxide. Anesthesiology. 1971;35:482–487.
75 Yli-Hankala A, Vakkuri A, Sarkela M, et al. Epileptiform electroencephalogram during mask induction of anesthesia with sevoflurane. Anesthesiology. 1999;91:1596–1603.
76 Tramer M, Moore A, McQuay H. Omitting nitrous oxide in general anaesthesia: meta-analysis of intraoperative awareness and postoperative emesis in randomized controlled trials. Br J Anaesth. 1996;76:186–193.
77 Bortone L, Picetti E, Mergoni M. Anaesthesia with sevoflurane in children: nitrous oxide does not increase postoperative vomiting. Paediatr Anaesth. 2002;12:775–779.
78 Moss E, McDowall DG. ICP increases with 50% nitrous oxide in oxygen in severe head injuries during controlled ventilation. Br J Anaesth. 1979;51:757–761.
79 Reinstrup P, Ryding E, Algotsson L, Berntman L, Uski T. Effects of nitrous oxide on human regional cerebral blood flow and isolated pial arteries. Anesthesiology. 1994;81:396–402.
80 Misfeldt BB, Jörgensen PB, Rishöj M. The effect of nitrous oxide and halothane upon the intracranial pressure in hypocapnic patients with intracranial disorders. Br J Anaesth. 1974;46:853–858.
81 Sloan TB, Koht A. Depression of cortical somatosensory evoked potentials by nitrous oxide. Br J Anaesth. 1985;57:849–852.
82 Peterson DO, Drummond JC, Todd MM. Effects of halothane, enflurane, isoflurane, and nitrous oxide on somatosensory evoked potentials in humans. Anesthesiology. 1986;65:35–40.
83 Pathak KS, Ammadio M, Kalamchi A, et al. Effects of halothane, enflurane, and isoflurane on somatosensory evoked potentials during nitrous oxide anesthesia. Anesthesiology. 1987;66:753–757.
84 Hartung J, Cottrell JE. Nitrous oxide reduces thiopental-induced prolongation of survival in hypoxic and anoxic mice. Anesth Analg. 1987;66:47–52.
85 Guy J, Hindman BJ, Baker KZ, et al. Comparison of remifentanil and fentanyl in patients undergoing craniotomy for supratentorial space-occupying lesions. Anesthesiology. 1997;86:514–524.
86 Reasoner DK, Todd MM, Scamman FL, Warner DS. The incidence of pneumocephalus after supratentorial craniotomy: observations on the disappearance of intracranial air. Anesthesiology. 1994;80:1008–1012.
87 Ard J, Doyle W, Bekker A. Awake craniotomy with dexmedetomidine in pediatric patients. J Neurosurg Anesthesiol. 2003;15:263–266.
88 Ma D, Hossain M, Rajakumaraswamy N, et al. Dexmedetomidine produces its neuroprotective effect via the alpha 2A-adrenoreceptor subtype. Eur J Pharmacol. 2004;502:87–97.
89 Ibacache ME, Munoz HR, Brandes V, Morales AL. Single-dose dexmedetomidine reduces agitation after sevoflurane anesthesia in children. Anesth Analg. 2004;98:60–63.
90 Bekker A, Sturaitis MK. Dexmedetomidine for neurologic surgery. Neurosurgery. 2005;57(Suppl 1):1–10.
91 Ikonomidou C, Bosch F, Miksa M, et al. Blockade of NMDA receptors and apoptotic neurodegeneration in the developing brain. Science. 1999;283:70–74.
92 Jevtovic-Todorovic V, Hartman RE, Izumi Y, et al. Early exposure to common anesthetic agents causes widespread neurodegeneration in the developing rat brain and persistent learning deficits. J Neurosci. 23, 2003. 876–872
93 Slikker W, Jr., Zou X, Hotchkiss CE, et al. Ketamine-induced neuronal cell death in the perinatal rhesus monkey. Toxicol Sci. 2007;98:145–158.
94 Todd MM. Anesthetic neurotoxicity: the collision between laboratory neuroscience and clinical medicine. Anesthesiology. 2004;101:533–534.
95 Olney JW, Young C, Wozniak DF, et al. Anesthesia induced developmental neuroapoptosis: does it happen in humans? Anesthesiology. 2004;101:273–275.
96 Anand KJS, Soriano SG. Anesthetic agents and the immature brain: are these toxic or therapeutic agents? Anesthesiology. 2004;101:527–530.
97 Flick RP, Katusic SK, Colligan RC, et al. Cognitive and behavioral outcomes after early exposure to anesthesia and surgery. Pediatrics. 2011;128:e1053–e1061.
98 Shekhar C. Anesthesia: a medical mainstay re-examined. Some worry about brain cell death in studies of young animals. Human trials are planned. Los Angeles Times. 2007. May 14
99 Loepke AW, McCann JC, Kurth CD, McAuliffe JJ, et al. The physiologic effects of isoflurane anesthesia in neonatal mice. Anesth Analg. 2006;102:72–74.
100 DiMaggio C, Sun LS, Li G. Early childhood exposure to anesthesia and risk of developmental and behavioral disorders in a sibling birth cohort. Anesth Analg. 2011;113:1143–1151.
101 Flick RP, Katusic SK, Colligan RC, et al. Cognitive and behavioral outcomes after early exposure to anesthesia and surgery. Pediatrics. 2011;128:e1053–e1061.
102 Wilder RT, Flick RP, Sprung J, et al. Early exposure to anesthesia and learning disabilities in a population-based birth cohort. Anesthesiology. 2009;110:796–804.
103 Bartels M, Althoff RR, Boomsma DI. Anesthesia and cognitive performance in children: no evidence for a causal relationship. Twin Res Human Genetics. 2009;12:246–253.
104 Scheingraber S, Rehm M, Sehmisch C, Finsterer U. Rapid saline infusion produces hyperchloremic acidosis in patients undergoing gynecologic surgery. Anesthesiology. 1999;90:1265–1270.
105 Coté CJ, Greenhow DE, Marshall BE. The hypotensive response to rapid intravenous administration of hypertonic solutions in man and in the rabbit. Anesthesiology. 1979;50:30–35.
106 Wass CT, Lanier WL. Glucose modulation of ischemic brain injury: review and clinical recommendations. Mayo Clin Proc. 1996;71:801–812.
107 Murat I, Berniere J, Constant I. Evaluation of the efficacy of a forced-air warmer (Bair Hugger) during spinal surgery in children. J Clin Anesth. 1994;6:425–429.
108 Harris MM, Yemen TA, Davidson A, et al. Venous embolism during craniectomy in supine infants. Anesthesiology. 1987;67:816–819.
109 Yoshida M, Alkalay I, Rotman HH, Kimbel P. Pulmonary vascular changes following air embolism in the dog. Arch Int Physiol Biochem. 1978;86:317–325.
110 Schwarz G, Fuchs G, Weihs W, et al. Sitting position for neurosurgery: experience with preoperative contrast echocardiography in 301 patients. J Neurosurg Anesthesiol. 1994;6:83–88.
111 Porter JM, Pidgeon C, Cunningham AJ. The sitting position in neurosurgery: a critical appraisal. Br J Anaesth. 1999;82:117–128.
112 Faberowski LW, Black S, Mickle JP. Incidence of venous air embolism during craniectomy for craniosysnostosis repair. Anesthesiology. 2000;92:20–23.
113 Meier PM, Goobie SM, DiNardo JA, et al. Endoscopic strip craniectomy in early infancy: the initial five years of anesthesia experience. Anesth Analg. 2011;112:407–414.
114 Cucchiara RF, Bowers B. Air embolism in children undergoing suboccipital craniotomy. Anesthesiology. 1982;57:338–339.
115 Schafer ST, Lindemann J, Brendt P, Kaiser G, Peters J. Intracardiac transvenous echocardiography is superior to both precordial Doppler and transesophgeal echocardiography techniques for detecting venous air embolism and cather-guided air aspiration. Anesth Analg. 2008;106:45–54.
116 Glenski JA, Cucchiara RF, Michenfelder JD. Transesophageal echocardiography and transcutaneous O2 and CO2 monitoring for detection of venous air embolism. Anesthesiology. 1986;64:541–545.
117 Albin MS, Carroll RG, Maroon JC. Clinical considerations concerning detection of venous air embolism. Neurosurgery. 1978;3:380–384.
118 Schubert A, Deogaonkar A, Drummond JC. Precordial Doppler probe placement for optimal detection of venous air embolism during craniotomy. Anesth Analg. 2006;102:1543–1547.
119 Furuya H, Suzuki T, Okumura F, Kishi Y, Uefuji T. Detection of air embolism by transesophageal echocardiography. Anesthesiology. 1983;58:124–129.
120 Mammoto T, Hayashi Y, Ohnishi Y, Kuro M. Incidence of venous and paradoxical air embolism in neurosurgical patients in the sitting position: detection by transesophageal echocardiography. Acta Anaesthesiol Scand. 1998;42:643–647.
121 Pesce C, Mercurella A, Musi L, Campobasso P, Negri M. Fatal cardiac tamponade as a late complication of central venous catheterization: a case report. Eur J Pediatr Surg. 1999;9:113–115.
122 van Engelenburg KC, Festen C. Cardiac tamponade: a rare but life-threatening complication of central venous catheters in children. J Pediatr Surg. 1998;33:1822–1824.
123 Bruder N, Ravussin P. Recovery from anesthesia and postoperative extubation of neurosurgical patients: a review. J Neurosurg Anesthesiol. 1999;11:282–293.
124 Furst SR, Sullivan LJ, Soriano SG, et al. Effects of ondansetron on emesis in the first 24 hours after craniotomy in children. Anesth Analg. 1996;83:325–328.
125 Muzzi DA, Black S, Losasso TJ, Cucchiara RF. Labetalol and esmolol in the control of hypertension after intracranial surgery. Anesth Analg. 1990;70:68–71.
126 Birmingham PK, Tobin MJ, Henthorn TK, et al. Twenty-four-hour pharmacokinetics of rectal acetaminophen in children: an old drug with new recommendations. Anesthesiology. 1997;87:244–252.
127 Wise-Faberowski L, Soriano SG, Ferrari L, et al. Perioperative management of diabetes insipidus in children. J Neurosurg Anesthesiol. 2004;16:220–225.
128 Kissoon N, Dreyer J, Walia M. Pediatric trauma: differences in pathophysiology, injury patterns and treatment compared with adult trauma. Can Med Assoc J. 1990;142:27–34.
129 Pascucci RC. Head trauma in the child. Intensive Care Med. 14, 1988. 185–185
130 Bruce DA, Raphaely RC, Goldberg AI, et al. Pathophysiology, treatment and outcome following severe head injury in children. Childs Brain. 1979;5:174–191.
131 Bruce DA, Alavi A, Bilaniuk L, et al. Diffuse cerebral swelling following head injuries in children: the syndrome of “malignant brain edema.”. J Neurosurg. 1981;54:170–178.
132 Rahimi-Movaghar V, Boroojeny SB, Moghtaderi A, Keshmiran B. Intracranial placement of a nasogastric tube: a lesson to be re-learnt? Acta Neurochir (Wien). 2005;147:573–574.
133 Cornett MA, Paris A, Jr., Huang TY. Intracranial placement of a nasogastric tube. Am J Emerg Med. 1993;11:94–96.
134 Fremstad JD, Martin SH. Lethal complication from insertion of nasogastric tube after severe basilar skull fracture. J Trauma. 1978;18:820–822.
135 American Academy of Pediatrics Committee on Child Abuse and Neglect. Shaken baby syndrome: inflicted cerebral trauma. Pediatrics. 1993;92:872–875.
136 Duhaime AC, Christian CW, Rorke LB, Zimmerman RA. Nonaccidental head injury in infants: the “shaken-baby syndrome.”. N Engl J Med. 1998;338:1822–1829.
137 Conway EE, Jr. Nonaccidental head injury in infants: “the shaken baby syndrome revisited.”. Pediatr Ann. 1998;27:677–690.
138 Hamilton MG, Myles ST. Pediatric spinal injury: review of 174 hospital admissions. J Neurosurg. 1992;77:700–704.
139 Hamilton MG, Myles ST. Pediatric spinal injury: review of 61 deaths. J Neurosurg. 1992;77:705–708.
140 Osenbach RK, Menezes AH. Pediatric spinal cord and vertebral column injury. Neurosurgery. 1992;30:385–390.
141 Osenbach RK, Menezes AH. Spinal cord injury without radiographic abnormality in children. Pediatr Neurosci. 1989;15:168–174.
142 Pang D, Wilberger JE, Jr. Spinal cord injury without radiographic abnormalities in children. J Neurosurg. 1982;57:114–129.
143 Tunkel DE, Fisher QA. Pediatric flexible fiberoptic bronchoscopy through the laryngeal mask airway. Arch Otolaryngol Head Neck Surg. 1996;122:1364–1367.
144 Badr A, Tobias JD, Rasmussen GE, et al. Bronchoscopic airway evaluation facilitated by the laryngeal mask airway in pediatric patients. Pediatr Pulmonol. 1996;21:57–61.
145 Bahk JH, Han SM, Kim SD. Management of difficult airways with a laryngeal mask airway under propofol anaesthesia. Paediatr Anaesth. 1999;9:163–166.
146 Haynes SR, Morton NS. The laryngeal mask airway: a review of its use in paediatric anaesthesia. Paediatr Anaesth. 1993;3:65–73.
147 Martens P. The use of the laryngeal mask airway by nurses during cardiopulmonary resuscitation: results of a multicentre trial. Anaesthesia. 1994;49:3–7.
148 Reinhart DJ, Simmons G. Comparison of placement of the laryngeal mask airway with endotracheal tube by paramedics and respiratory therapists. Ann Emerg Med. 1994;24:260–263.
149 Coté CJ, Eavey RD, Todres ID, Jones DE. Cricothyroid membrane puncture: oxygenation and ventilation in a dog model using an intravenous catheter. Crit Care Med. 1988;16:615–619.
150 Bracken MB, Collins WF, Freeman DF, et al. Efficacy of methylprednisolone in acute spinal cord injury. JAMA. 1984;251:45–52.
151 Bracken MB, Shepard MJ, Collins WF, et al. A randomized, controlled trial of methylprednisolone or naloxone in the treatment of acute spinal-cord injury: results of the Second National Acute Spinal Cord Injury Study. N Engl J Med. 1990;322:1405–1411.
152 Hall ED. The neuroprotective pharmacology of methylprednisolone. J Neurosurg. 1992;76:13–22.
153 Geisler FH. Clinical trials of pharmacotherapy for spinal cord injury. Ann N Y Acad Sci. 1998;845:374–381.
154 Zeidman SM, Ling GS, Ducker TB, Ellenbogen RG. Clinical applications of pharmacologic therapies for spinal cord injury. J Spinal Disord. 1996;9:367–380.
155 Constantini S, Young W. The effects of methylprednisolone and the ganglioside GM1 on acute spinal cord injury in rats. J Neurosurg. 1994;80:97–111.
156 Nobile-Orazio E, Carpo M, Scarlato G. Gangliosides: their role in clinical neurology. Drugs. 1994;47:576–585.
157 Geisler FH, Dorsey FC, Coleman WP. Past and current clinical studies with GM-1 ganglioside in acute spinal cord injury. Ann Emerg Med. 1993;22:1041–1047.
158 Geisler FH. GM-1 ganglioside and motor recovery following human spinal cord injury. J Emerg Med. 1993;11:49–55.
159 Skaper SD, Leon A. Monosialogangliosides, neuroprotection, and neuronal repair processes. J Neurotrauma. 1992;9:S507–S516.
160 Gronert GA, Theye RA. Pathophysiology of hyperkalemia induced by succinylcholine. Anesthesiology. 1975;43:89–99.
161 Amzallag M. Autonomic hyperreflexia. Int Anesthesiol Clin. 1993;31:87–102.
162 Colachis SC, III. Autonomic hyperreflexia with spinal cord injury. J Am Paraplegia Soc. 1992;15:171–186.
163 Pollack IF. Brain tumors in children. N Engl J Med. 1994;331:1500–1507.
164 Dingeman RS, Goumnerova LC, Goobie SM. The use of a laryngeal mask airway for emergent airway management in a prone child. Anesth Analg. 2005;100:670–671.
165 Young WL, Solomon RA, Pedley TA, et al. Direct cortical EEG monitoring during temporary vascular occlusion for cerebral aneurysm surgery. Anesthesiology. 1989;71:794–799.
166 Millar C, Bissonnette B, Humphreys RP. Cerebral arteriovenous malformations in children. Can J Anaesth. 1994;41:321–331.
167 Ostergaard JR, Voldby B. Intracranial arterial aneurysms in children and adolescents. J Neurosurg. 1983;58:832–837.
168 Salem MR, Wong AY, Bennett EJ, Mani M. Deliberate hypotension in infants and children. Anesth Analg. 1974;53:975–981.
169 Suzuki J, Takaku A. Cerebrovascular “moyamoya” disease: disease showing abnormal net-like vessels in base of brain. Arch Neurol. 1969;20:288–299.
170 Smith ER, McClain CD, Heeney M, Scott RM. Pial synangiosis in patients with moyamoya disease and sickle cell anemia: perioperative management and surgical outcome. Neurosurg Focus. 2009;26:E10.
171 Sunder TR, Erwin CW, Dubois PJ. Hyperventilation induced abnormalities in the electroencephalogram of children with moyamoya disease. Electroencephalogr Clin Neurophysiol. 1980;49:414–420.
172 Adelson PD, Scott RM. Pial synangiosis for moyamoya syndrome in children. Pediatr Neurosurg. 1995;23:26–33.
173 Soriano SG, Sethna NF, Scott RM. Anesthetic management of children with moyamoya syndrome. Anesth Analg. 1993;77:1066–1070.
174 Takeuchi S, Tanaka R, Ishii R, et al. Cerebral hemodynamics in patients with moyamoya disease: a study of regional cerebral blood flow by the 133Xe inhalation method. Surg Neurol. 1985;23:468–474.
175 Vendrame M, Kaleyias J, Loddenkemper T, et al. Electroencephalogram monitoring during intracranial surgery for moyamoya disease. Pediatr Neurol. 2011;44:427–432.
176 Sakamoto T, Kawaguchi M, Kurehara K, et al. Postoperative neurological deterioration following the revascularization surgery in children with moyamoya disease. J Neurosurg Anesthesiol. 1998;10:37–41.
177 Vickrey BG, Hays RD, Rausch R, et al. Outcomes in 248 patients who had diagnostic evaluations for epilepsy surgery. Lancet. 1995;346:1445–1449.
178 Soriano SG, Eldredge EA, Wang FK, et al. The effect of propofol on intraoperative electrocorticography and cortical stimulation during awake craniotomies in children. Paediatr Anaesth. 2000;10:29–34.
179 Modica PA, Tempelhoff R, White PF. Pro- and anticonvulsant effects of anesthetics: II. Anesth Analg. 1990;70:433–444.
180 Ford EW, Morrell F, Whisler WW. Methohexital anesthesia in the surgical treatment of uncontrollable epilepsy. Anesth Analg. 1982;61:997–1001.
181 Carson BS, Javedan SP, Freeman JM, et al. Hemispherectomy: a hemidecortication approach and review of 52 cases. J Neurosurg. 1996;84:903–911.
182 McLachlan RS. Vagus nerve stimulation for intractable epilepsy: a review. J Clin Neurophysiol. 1997;14:358–368.
183 Schachter SC, Saper CB. Vagus nerve stimulation. Epilepsia. 1998;39:677–686.
184 FineSmith RB, Zampella E, Devinsky O. Vagal nerve stimulator: a new approach to medically refractory epilepsy. N J Med. 1999;96:37–40.
185 Hornig GW, Murphy JV, Schallert G, Tilton C. Left vagus nerve stimulation in children with refractory epilepsy: an update. South Med J. 1997;90:484–488.
186 Hatton KW, McLarney JT, Pittman T, Fahy BG. Vagal nerve stimulation: overview and implications for anesthesiologists. Anesth Analg. 2006;103:1241–1249.
187 Black PM, Hakim R, Bailey NO. The use of the Codman-Medos Programmable Hakim valve in the management of patients with hydrocephalus: illustrative cases. Neurosurgery. 1994;34:1110–1113.
188 Goumnerova LC, Frim DM. Treatment of hydrocephalus with third ventriculocisternostomy: outcome and CSF flow patterns. Pediatr Neurosurg. 1997;27:149–152.
189 Warf BC. Endoscopic third ventriculostomy and choroid plexus cauterization for pediatric hydrocephalus. Clin Neurosurg. 2007;54:78–82.
190 Filanovsky Y, Miller P, Kao J. Myth: ketamine should not be used as an induction agent for intubation in patients with head injury. CJEM. 2010;12:154–157.
191 Bar-Joseph G, Guilburd Y, Tamir A, Guilburd JN. Effectiveness of ketamine in decreasing intracranial pressure in children with intracranial hypertension. J Neurosurg Pediatr. 2009;4:40–46.
192 Schmidt A, Øye I, Akeson J. Racemic, S(+)- and R(-)-ketamine do not increase elevated intracranial pressure. Acta Anaesthesiol Scand. 2008;52:1124–1130.
193 Moynihan RJ, Brock-Utne JG, Archer JH, Feld LH, Kreitzman TR. The effect of cricoid pressure on preventing gastric insufflation in infants and children. Anesthesiology. 1993;78:652–656.
194 Dierdorf SF, McNiece WL, Rao CC, Wolfe TM, Means LJ. Failure of succinylcholine to alter plasma potassium in children with myelomeningocoele. Anesthesiology. 1986;64:272–273.
195 Birmingham PK, Dsida RM, Grayhack JJ, et al. Do latex precautions in children with myelodysplasia reduce intraoperative allergic reactions? J Pediatr Orthop. 1996;16:799–802.
196 De Queiroz M, Combet S, Bérard J, et al. Latex allergy in children: modalities and prevention. Pediatr Anesth. 2009;19:313–319.
197 Vandenplas O, Delwiche JP, De Jonghe M. Asthma to latex and amoxicillin. Allergy. 1997;52:1147–1149.
198 Ventura MT, Di Corato R, Dagnello M, et al. Latex and amoxicillin induced asthma. Allergy. 2000;55:587–588.
199 Oren J, Kelly DH, Todres ID, Shannon DC. Respiratory complications in patients with myelodysplasia and Arnold-Chiari malformation. Am J Dis Child. 1986;140:221–224.
200 Sutton LN, Adzick NS, Bilaniuk LT, et al. Improvement in hindbrain herniation demonstrated by serial fetal magnetic resonance imaging following fetal surgery for myelomeningocele. JAMA. 1999;282:1826–1831.
201 Bruner JP, Tulipan N, Paschall RL, et al. Fetal surgery for myelomeningocele and the incidence of shunt-dependent hydrocephalus. JAMA. 1999;282:1819–1825.
202 Adzick NS, Thom EA, Spong CY, et al. A randomized trial of prenatal versus postnatal repair of myelomeningocele. N Engl J Med. 2011;364:993–1004.
203 Ward SL, Nickerson BG, van der Hal A, et al. Absent hypoxic and hypercapneic arousal responses in children with myelomeningocele and apnea. Pediatrics. 1986;78:44–50.
204 Putnam PE, Orenstein SR, Pang D, et al. Cricopharyngeal dysfunction associated with Chiari malformations. Pediatrics. 1992;89:871–876.
205 Manninen PH, Kucharczyk W. A new frontier: magnetic resonance imaging-operating room. J Neurosurg Anesthesiol. 2000;12:141–148.
206 Berkenstadt H, Perel A, Ram Z, et al. Anesthesia for magnetic resonance guided neurosurgery. J Neurosurg Anesthesiol. 2001;13:158–162.
207 McClain CD, Soriano SG, Goumnerova LG, Black PM, Rockoff MA. Detection of unanticipated intracranial hemorrhage during intraoperative magnetic resonance image-guided neurosurgery: report of two cases. J Neurosurg. 2007;106(Suppl):398–400.
208 McClain CD, Rockoff MA, Soriano SG. Anesthetic concerns for pediatric patients in an intraoperative MRI suite. Curr Opin Anesthesiol. 2011;24:480–486.
209 Cox RG, Levy R, Hamilton MG, et al. Anesthesia can be safely provided for children in a high-field intraoperative magnetic resonance imaging environment. Paediatr Anaesh. 2011;21:454–458.