16 Neurological Assessment and Monitoring
After reading this chapter, you should be able to:
• describe the anatomy and physiology of the nervous system
• differentiate between the central and peripheral nervous systems
• describe the techniques used for neurological assessment
• identify the distinction between normal and abnormal findings
• state the determinants of intracranial pressure and describe compensatory mechanisms used to prevent large changes in intracranial pressure when there are changes in brain, blood and cerebrospinal fluid volumes
• explain the importance and process of continuous neurological assessment in the brain-injured patient
• relate the procedures of selected neurodiagnostic tests to nursing implications for patient care
Neurological Anatomy and Physiology
Components of the Nervous System
The central nervous system (CNS) consists of the spinal cord and the brain and is responsible for integrating, processing and coordinating sensory data and motor commands1 (see Figure 16.1). The CNS is linked to all parts of the body by the PNS which transmits signals to and from the CNS. The human PNS is composed of 43 pairs of spinal nerves that issue in orderly sequence from the spinal cord, and 12 pairs of cranial nerves that emerge from the base of the brain. All branch and diversify prolifically as they distribute to the tissues and organs of the body. The peripheral nerves carry input to the CNS via their sensory afferent fibres and deliver output from the CNS via the efferent fibres. Specific physiology of the CNS and PNS is discussed in detail later in the chapter. First, however, neuron cell anatomy and physiology is examined.
Neurons
Neurons are specialised cells in the nervous system; each is comprised of a dendrite, cell body (soma) and axon.2 Each neuron is a cell that uses biochemical reactions to receive, process and transmit information. Most synaptic contacts between neurons are either axodendritic (excitatory) or axosomatic (inhibitory). A neuron’s dendritic tree is connected to many neighbouring neurons and receives positive or negative charges from other neurons. The input is then passed to the soma (cell body). The primary role of the soma and the enclosed nucleus is to perform the continuous maintenance required to keep the neuron functional. Most neurons lack centrioles, important organelles involved in the organisation of the cytoskeleton and the movement of chromosomes during mitosis. As a result, typical CNS neurons cannot divide and cannot be replaced if lost to injury or disease. The fuel source for the neuron is glucose; insulin is not required for cellular uptake in the CNS.
A myelin sheath, consisting of a lipid-protein casing, covers the neuron and provides protection to the axon and speeds the transmission of impulses along nerve cells from node to node.3 (see Figure 16.2b). Myelin is not a continuous layer but has gaps called nodes of Ranvier (see Figure 16.2a).
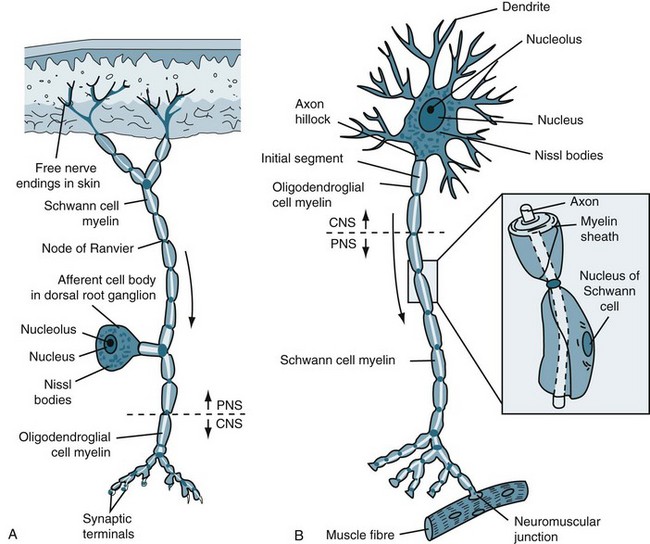
FIGURE 16.2 (A) Afferent and (B) efferent neurons, showing the soma or cell body, dendrites and axon. Arrows indicate the direction for conduction of action potentials.3
Each synaptic knob contains mitochondria, portions of the endoplasmic reticulum, and thousands of vesicles filled with neurotransmitter molecules. Breakdown products of neurotransmitter released at the synapse are reabsorbed and reassembled at the synaptic knob. The synaptic knob also receives a continuous supply of neurotransmitter synthesised in the cell body, along with enzymes and lysosomes. The movement of materials between the cell body and synaptic knobs is called axoplasmic transport. Some materials travel slowly, at rates of a few millimetres per day. This transport mechanism is known as the ‘slow stream.’ Vesicles containing neurotransmitter move much more rapidly, travelling in the ‘fast stream’ at 5–10 mm per hour which increases synaptic activity. Axoplasmic transport occurs in both directions. The flow of materials from the cell body to the synaptic knob is anterograde flow. At the same time, other substances are being transported towards the cell body in retrograde flow (’retro’ meaning backward). If debris or unusual chemicals appear in the synaptic knob, retrograde flow soon delivers them to the cell body. The arriving materials may then alter the activity of the cell by turning appropriate genes on or off. Retrograde flow is the means of transport for viruses, pathogenic bacteria, heavy metals and toxins to the CNS, with resulting disease such as tetanus, viral encephalitis and lead intoxication. Defective anterograde transport seems to be involved in certain neuropathies, including critical illness neuropathies.4
Synapses
The human brain contains at least 100 billion neurons, each with the ability to influence many other cells. Although there are many kinds of synapses within the brain, they can be divided into two general classes: electrical synapses and chemical synapses. Electrical synapses permit direct, passive flow of electrical current from one neuron to another in the form of an action potential; they are described in Table 16.1. The current flows through gap junctions, which are specialised membrane channels that connect the two cells. Chemical synapses, in contrast, enable cell-to-cell communication via the secretion of neurotransmitters; the chemical agents released by the presynaptic neurons produce secondary current flow in postsynaptic neurons by activating specific receptor molecules5 (see Figure 16.3).
STEP 4: Return to normal permeability
Neurotransmitters
Chemically, there are four classes of neurotransmitters:
1. acetylcholine (ACh): the dominant neurotransmitter in the peripheral nervous system, released at neuromuscular junctions and synapses of the parasympathetic division
2. biogenic amines: serotonin, histamine, and the catecholamines dopamine and noradrenaline
3. excitatory amino acids: glutamate and aspartate, and the inhibitory amino acids gamma-aminobutyric acid (GABA), glycine and taurine
4. neuropeptides: over 50 of which are known, amino acid neurotransmitters being the most numerous.
In 2009, it was discovered that there is also more than one neurotransmitter per synapse; these are called co-transmitters. For example, neuropeptide Y (NPY) and adenosine triphosphate (ATP) are co-transmitters of noradrenaline, which are released together and mediate their function by activation of α- and β-adrenoceptors, and regulate renovascular resistance.6 Similarly, receptors are an important control point for the effectiveness of synapses. Neurotransmitters are the common denominator between the nervous, endocrine and immune systems. Many neurotransmitters are endocrine analogues and acetylcholine, the main parasympathetic neurotransmitter, interacts with immune cells such as macrophages through the anti-inflammatory cholinergic pathway.7
Neuroglia
Neuroglia are the non-neuronal cells of the nervous system and are 10–50 times more prevalent than the number of neurons.1 They are divided into macroglia (astrocytes, oligodendroglia and Schwann cells) and microglia, and are described in Table 16.2. They not only provide physical support but also respond to injury, regulate the ionic and chemical composition of the extracellular milieu, participate in the blood–brain and blood–retina barriers, form the myelin insulation of nervous pathways, guide neuronal migration during development, and exchange metabolites with neurons.8 The CNS has a greater variety of neuroglia. Unlike neurons, neuroglia continue to multiply throughout life. Because of their capacity to reproduce, most tumours of the nervous system are tumours of neuroglial tissue and not of nervous tissue itself.9
TABLE 16.2 Neuroglia, their location and role as supporting nervous tissue
Type | Location | Main Function |
---|---|---|
Astrocytes | CNS: The largest and most numerous neuroglial cells in the brain and spinal cord. |
PNS: Schwann cells are the supporting cells of the PNS.
Central Nervous System
The CNS is composed of the brain and spinal cord (see Figure 16.4).5 The primary purpose is to acquire, coordinate and disseminate information about the body and its environment. This section describes the anatomy and physiology of the brain and spinal cord.
Brain
The brain is divided into three regions: forebrain, midbrain and hindbrain, as described in Table 16.3. The forebrain, which consists of two hemispheres and is covered by the cerebral cortex, contains central masses of grey matter, the basal ganglia, the neural tube and the diencephalon with its adult derivatives: the thalamus and hypothalamus.1 Midbrain structures include two pairs of dorsal enlargements, the superior and inferior colliculi. The medulla, pons and midbrain compose the brainstem.1 The hindbrain includes the medulla oblongata, the pons and its dorsal outgrowth, the cerebellum.
Division | Description | Functions |
---|---|---|
Forebrain | ||
Cerebrum | Largest and uppermost portion of the brain. Divided into two hemispheres, each subdivided into the frontal, parietal, temporal and occipital lobes. | Cortex (outer layer) is the site of conscious thought, memory, reasoning and abstract mental functions, all localised within specific lobes. |
Nervous tissue has a high rate of metabolism. Although the brain constitutes only 3% of the body’s weight, it receives approximately 15% of the resting cardiac output and consumes 20% of its oxygen.1 Despite its substantial energy requirements, the brain can neither store oxygen nor effectively engage in anaerobic metabolism. An interruption in the blood or oxygen supply to the brain rapidly leads to clinically observable signs and symptoms. Without oxygen, brain cells continue to function for approximately 10 seconds. Glucose is virtually the sole energy substrate for the brain, and it is entirely oxidised.10 The brain can be seen as an almost exclusive glucose-processing machine, producing water (H2O) and carbon dioxide (CO2). Glucose also provides the carbon backbone for regeneration of the neuronal pool of glutamate. This process results from close astrocyte–neuron cooperation.11
Cerebral cortex
The forebrain contains the cerebral cortex and the subcortical structures rostral (sideways) to the diencephalon. The cortex, or outermost surface of the cerebrum, makes up about 80% of the human brain. The cerebral cortex varies in thickness from 2 mm to 4 mm, being thinnest in the primary sensory areas and thickest in the motor and association areas. It contains the cell bodies and dendrites of neurons or grey matter which receive, integrate, store and transmit information. Conscious deliberation and voluntary actions also arise from the cerebral cortex. White matter lies beneath the cerebral cortex and is composed of myelinated nerve fibres. The cortex is involved in the processing of both sensory information from the body and the delivery of motor commands. These occur in specific areas of the brain and can be mapped. Topographically, the cerebral cortex is divided into areas of specialised functions, including the primary sensory areas for vision (occipital cortex), hearing (temporal cortex), somatic sensation (postcentral gyrus), and primary motor area (precentral gyrus). As shown in Figure 16.5,1 these well-defined areas comprise only a small fraction of the surface of the cerebral cortex.
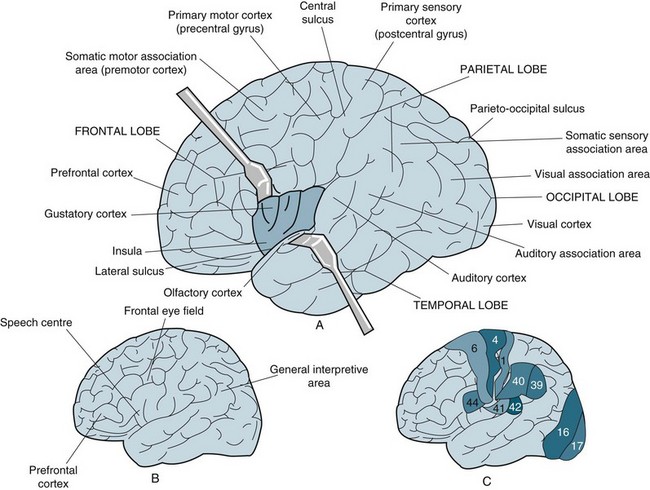
FIGURE 16.5 (A) Major anatomical landmarks on the surface of the left cerebral hemisphere. The lateral sulcus has been pulled apart to expose the insula. (B) The left hemisphere generally contains the general interpretive area and the speech centre. The prefrontal cortex of each hemisphere is involved with conscious intellectual functions. (C) Regions of the cerebral cortex as determined by histological analysis. Several of the 47 regions described by Brodmann are shown for comparison with the results of functional mapping.1
The majority of the remaining cortical area is known as the association cortex, where the processing of extensive and sophisticated neural information is performed.12 The association areas are also sites of long-term memory, and they control human functions such as language acquisition, speech, musical ability, mathematical ability, complex motor skills, abstract thought, symbolic thought, and other cognitive functions. Association areas interconnect and integrate information from the primary sensory and motor areas via intra-hemispheric connections. The parietal–temporal–occipital association cortex integrates neural information contributed by visual, auditory, and somatic sensory experiences. The prefrontal association cortex is extremely important as the coordinator of emotionally motivated behaviours, by virtue of its connections with the limbic system. In addition, the prefrontal cortex receives neural input from the other association areas and regulates motivated behaviours by direct input to the premotor area, which serves as the association area of the motor cortex. Sensory and motor functions are controlled by cortical structures in the contralateral hemisphere. Particular cognitive functions or components of these functions may be lateralised to one side of the brain.
The cerebral cortex receives sensory information from many different sensory organs and processes the information. The two hemispheres receive the information from the opposite sides of the body. Sensory information is relayed to the cortex by the thalamus. Parts of the cortex that receive this information are called primary sensory areas and cross at various points in the sensory pathway, because the cerebral cortex operates on a contralateral basis.13 The discriminative touch system crosses high, in the medulla. The pain system crosses low, in the spinal cord. The proprioceptive sensory system that guards balance and position goes to the cerebellum, which works ipsilaterally and therefore doesn’t cross. Almost every region of the body is represented by a corresponding region in both the primary motor cortex and the somatic sensory cortex.14
The homunculus (see Figure 16.6) visualises the connection between different areas of the body and areas in brain hemispheres.15 The body on the right side is the motor homunculus and on the left the sensory homunculus. Representations of parts of the body that exhibit fine motor control and sensory capabilities occupy a greater amount of space than those that exhibit less precise motor or sensory functions.
Basal ganglia and cerebellum
The basal ganglia, consisting of the caudate, putamen, globus pallidus, substantia nigra, subthalamic nucleus, and related nuclei in the brainstem, play an important role in movement, as evidenced by the hypokinetic/rigid and hyperkinetic disorders seen with lesions of various components. However, their role in the initiation and control of movement cannot be isolated from the motor activities of the cortex and brainstem centres discussed previously. Procedural memories for motor and other unconscious skills depend on the integrity of the premotor cortex, basal ganglia and cerebellum.16 The cerebellum plays a more obvious role in coordinating movements by giving feedback to the motor cortex, as well as by providing important influences on eye movements through brainstem connections, and on postural activity through projections down the spinal cord.
Brainstem
The brainstem is composed of the midbrain, the pons and the medulla oblongata.1 These structures connect the cerebrum and diencephalon with the spinal cord. Brainstem centres are organised into medial, lateral and aminergic systems. Collectively, these integrate vestibular, visual and somatosensory inputs for the control of eye movements and, through projections to the spinal cord, provide for postural adjustments. For example, these centres keep the images on matching regions of the retinas when the head moves by causing conjugate eye movements in the opposite direction to which the head is turned. This is the basis for the ‘doll’s eyes’ test in neurological assessment, in which the head is rapidly turned and the eyes move conjugately in the opposite direction, demonstrating the integrity of much of the brainstem. The sequence of sleep states is governed by a group of brainstem nuclei that project widely throughout the brain and spinal cord.17
The midbrain, inferior to the centre of the cerebrum, forms the superior part of the brainstem. It contains the reticular formation (which collects input from higher brain centres and passes it on to motor neurons), the substantia nigra (which regulates body movements; damage to the substantia nigra causes Parkinson’s disease) and the ventral tegmental area (which contains dopamine-releasing neurons that are activated by nicotinic acetylcholine receptors).18 White matter at the anterior of the midbrain conducts impulses between the higher centres of the cerebrum and the lower centres of the pons, medulla, cerebellum and spinal cord. The midbrain contains the autonomic reflex centres for pupillary accommodations to light, which constrict the pupil and accommodate the lens. The fibres travel in cranial nerve III, so damage to that nerve will also produce a dilated pupil. It also contains the ventral tegmental area, packed with dopamine-releasing neurons that synapse deep within the forebrain and seem to be involved in pleasure: amphetamines and cocaine bind to the same receptors that it activates, and this may account at least in part for their addictive qualities.
The medulla oblongata lies between the pons and the spinal cord and looks like a swollen tip to the spinal cord. Running down the ventral aspect of the medulla are the pyramids, which contain corticospinal fibres. The function of the medulla oblongata is to control automatic functions (e.g. breathing and heart rate) and to relay nerve messages from the brain to the spinal cord. Processing of interaural time differences for sound localisation occurs in the olivary nuclei. The neurons controlling breathing have mu (µ) receptors, the receptors to which opiates bind. This accounts for the suppressive effect of opiates on breathing. Impairment of any of the vital functions or reflexes involving these cranial nerves suggests medullary damage.19
The pons varolii is the part of the brainstem that lies between the medulla oblongata and the mesencephalon. It contains pneumotaxic and apneustic respiratory centres and fibre tracts connecting higher and lower centres, including the cerebellum. The pons seems to serve as a relay station, carrying signals from various parts of the cerebral cortex to the cerebellum. Nerve impulses coming from the eyes, ears and touch receptors are sent on to the cerebellum. The pons also participates in the reflexes that regulate breathing. Table 16.4 contains a description of the cranial nerves including their type of tract, their function and location of origin.
Hypothalamus and limbic system
The hypothalamus, the cingulate gyrus of the cortex, the amygdala and hippocampus in the temporal lobes, and the septum and interconnecting nerve fibre tracts among these areas comprise the limbic system. The hypothalamus and limbic systems, which are closely linked to homeostasis, act to regulate endocrine secretion and the autonomic nervous system, and to influence behaviour through emotions and drives.1 The hypothalamus integrates information from the forebrain, brainstem, spinal cord and various endocrine systems. This area of the brain also contains some of the centres for coordinated parasympathetic and sympathetic stimulation, as well as those for temperature regulation, appetite regulation, regulation of water balance by antidiuretic hormone (ADH), and regulation of certain rhythmic psychobiological activities (e.g. sleep). The release of stored serotonin from axon terminals in the diencephalon, medulla, thalamus, and a small forebrain area (DMTF), results in inactivation of the RAS and activation of the DMTF. DMTF activity results in the four stages of sleep. The hypothalamus contains a plethora of neurotransmitters. These are found in the terminals of axons that originate from neurons outside the hypothalamus, but most are synthesised within the hypothalamus itself. The list of putative neurotransmitters includes the ‘classic’ transmitters ACh, GABA, glutamate, serotonin, dopamine and noradrenaline, as well as literally dozens of peptides that have been identified in recent years.20
Protection and Support of the Brain
Cerebral Spinal Fluid
Cerebral spinal fluid (CSF) is an ultrafiltrate of blood plasma composed of 99% water with other constituents, making it close to the composition of the brain extracellular fluid.1 Approximately 500 mL CSF is secreted each day, but only approximately 150 mL is in the ventricular system at any one time, meaning that the CSF is continuously being absorbed. The CSF produced in the ventricles must flow through the interventricular foramen, the third ventricle, the cerebral aqueduct and the fourth ventricle to exit from the neural tube.21 Three openings, or foramina, allow the CSF to pass into the subarachnoid space (see Figure 16.7).1 Approximately 30% of the CSF passes down into the subarachnoid space that surrounds the spinal cord, mainly on its dorsal surface, and moves back up to the cranial cavity along its ventral surface. Reabsorption of CSF into the vascular system occurs, through a pressure gradient. The normal CSF pressure is approximately 10 mmHg in the lateral recumbent position, although it may be as low as 5 mmHg or as high as 15 mmHg in healthy persons. The microstructure of the arachnoid villi is such that if the CSF pressure falls below approximately 3 mmHg the passageways collapse, and reverse flow is blocked. Arachnoid villi function as one-way valves, permitting CSF outflow into the blood but not allowing blood to pass into the arachnoid spaces. The pressure in the CSF manifests as normal ICP.
Blood–Brain–Cerebral Spinal Fluid Barrier
The CNS is richly supplied with blood vessels that bring oxygen and nutrients to the cells there. However, many substances cannot easily be exchanged between blood and brain because the endothelial cells of the vessels and the astrocytes of the CNS form extremely tight junctions, collectively referred to as the blood–brain barrier (BBB).1 In particular, small non-charged, lipid-soluble molecules can cross the BBB with ease. Experimental and clinical evidence suggests that the BBB maintains the chemical environment for neuronal function and protects the brain from harmful substances.22 Substances in the blood that gain rapid entry to the brain include glucose, the important source of energy, certain ions that maintain a proper medium for electrical activity, and oxygen for cellular respiration. Small fat-soluble molecules, like ethanol, pass through the BBB. Some water-soluble molecules pass into the brain carried by special proteins in the plasma membrane of the endothelial cells. Excluded molecules include proteins, toxins, most antibiotics, and monoamines (e.g. neurotransmitters). Some of these unwanted molecules are actively transported out of the endothelial cells. When injured (by force or infection or oxidative processes), the permeability of the BBB is disrupted, allowing a proliferation of various chemicals and molecules – even bacteria – into the brain parenchyma, with at times devastating consequences.
Cerebral Circulation
The brain must maintain a constant flow of blood in order for brain activity to occur. The arterial blood flow to the brain consists of approximately 20% of the cardiac output (see Figure 16.8).5 Normal cerebral blood flow is 750 mL/min. The brain autoregulates blood flow over a wide range of blood pressure by vasodilation or vasoconstriction of the arteries.1 In response to decreased arterial flow, the circle of Willis can act as a protective mechanism by shunting blood from one side to the other or from the anterior to posterior portions of the brain. This compensatory mechanism delays neurological deterioration in patients.
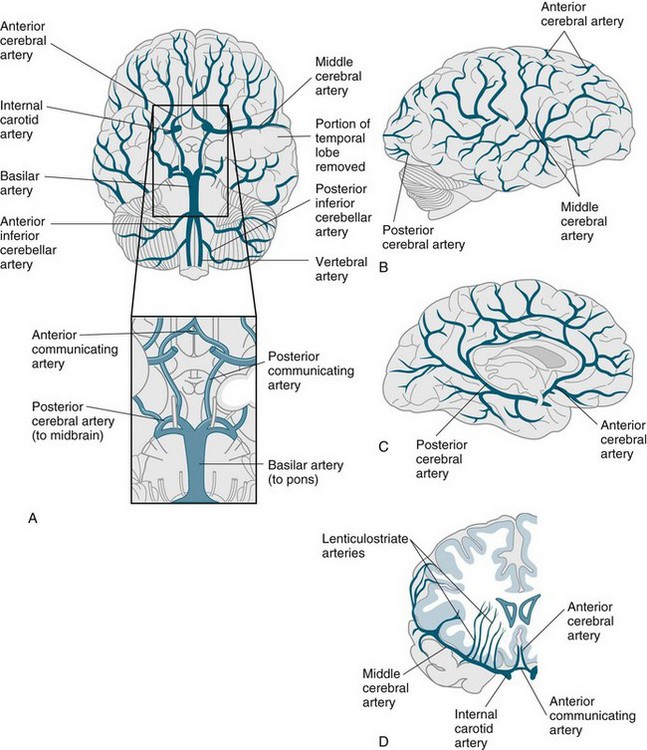
FIGURE 16.8 The major arteries of the brain: (A) ventral view: the enlargement of the boxed area showing the circle of Willis; (B) lateral and (C) midsagittal views showing anterior, middle and posterior cerebral arteries; (D) idealised frontal section showing course of middle cerebral artery.82
The cerebral veins drain into large venous sinuses and then into the right and left internal jugular veins (see Figure 16.9).23 The venous sinuses are found within the folds of the dura mater. The veins and sinuses of the brain do not have valves, so the blood flows freely by gravity.1 The face and scalp veins can flow into the brain venous sinuses; therefore, infection can easily be spread into the dural venous sinuses and then enter the brain. Patient position can prevent or promote venous drainage from the brain. Head turning and tilting may kink the jugular vein and decrease or stop venous flow from the brain, which will then raise the pressure inside the cranial vault.
Cerebral blood flow (CBF) is the cerebral perfusion pressure (CPP) divided by cerebrovascular resistance (CVR). CVR is the amount of resistance created by the cerebral vessels, and it is controlled by the autoregulatory mechanisms of the brain. Specifically, vasoconstriction (and vasospasm) will increase CVR, and vasodilation will decrease CVR.1 It is influenced by the inflow pressure (systole), outflow pressure (venous pressure), cross-sectional diameter of cerebral blood vessels, and intracranial pressure (ICP).1 CVR is similar to systemic vascular resistance; but, due to the lack of valves in the venous system of the brain, cerebral venous pressure also influences the CVR. An important characteristic of the cerebral circulation is its ability to autoregulate, that is, the ability to maintain constant cerebral blood flow despite variations in perfusion pressure (see Table 16.5). This is important in protecting the brain from both ischaemia during hypotension and haemorrhage during hypertension. CBF is affected by extrinsic and intrinsic factors.1 Extrinsic factors include systemic blood pressure, cardiac output, blood viscosity and vascular tone. The body responds to these demands with changes in blood flow. Aerobic metabolism is critically dependent on oxygen in order to process glucose for normal energy production, and the brain does not store energy. Therefore, without a constant source of oxygen and energy, its supply from CBF can be exhausted within 3 minutes. Intrinsic factors include PaCO2 (pH), PaO2 and ICP. The vessels dilate with increases in PaCO2 (hypercarbia) or low pH (acidosis) and with decreases in PaO2 (hypoxia). This vasodilation increases CBF. The vessels constrict with decreases in PaCO2 or high pH and with increases in local PaO2.1 This vasoconstriction will decrease the CBF. In addition, intrinsic factors can change the extrinsic factors by altering the metabolic mechanisms. These changes can lead to an alteration in the CBF. For example, there can be a change from aerobic to anaerobic metabolism, which increases the concentrations of other end-products such as lactic acid, pyruvic acid and carbonic acid, which causes a localised acidosis. These end-products result in a high pH which will cause an increase in CBF. Other factors that can affect CBF include pharmacological agents (anaesthetic agents and some antihypertensive agents), rapid-eye-movement sleep, arousal, pain, seizures, elevations in body temperature, and cerebral trauma.
TABLE 16.5 Changes in cerebrovascular and cerebrometabolic parameters when various cerebral variables are reduced with and without intact autoregulation
TABLE 16.6 PTA scale used to determine severity of brain injury
PTA Score | Severity |
---|---|
1–4 hours | Mild brain injury |
≤1 day | Moderate brain injury |
2–7 days | Severe brain injury |
1–4 weeks | Very severe brain injury |
1–6 months | Extremely severe brain injury |
>6 months | Chronic amnesia state |
Spinal Cord
The spinal cord is the link between the peripheral nervous system and the brain. The spinal cord has a small, irregularly shaped internal section of grey matter (unmyelinated tissue) surrounded by a larger area of white matter (myelinated axons). The internal grey matter is arranged so that a column of grey matter extends up and down dorsally, one on each side; another column is found in the ventral region on each side (see Figure 16.10).1
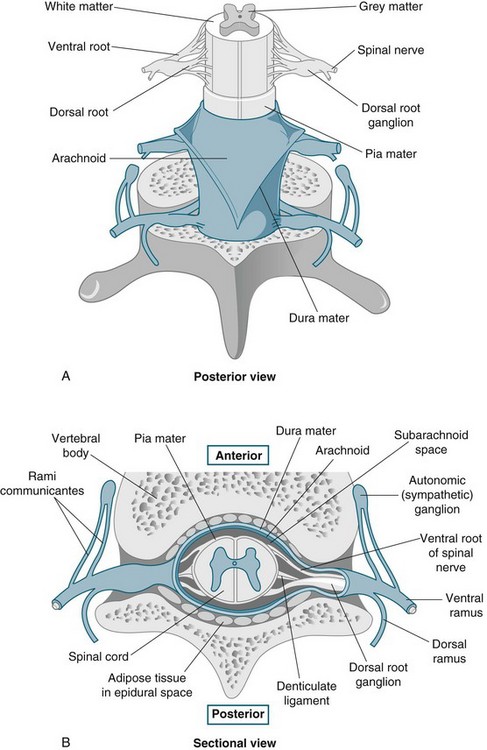
FIGURE 16.10 The spinal cord and spinal meninges; (A) posterior view of the spinal cord, showing the meningeal layers, superficial landmarks, and distribution of grey matter and white matter; (B) sectional view through the spinal cord and meninges, showing the peripheral distribution of spinal nerves.1
The spinal cord is an essential component of both the sensory and motor divisions of the nervous system. The first of the primary functions of the spinal cord is to transmit sensory impulses along the ascending tracts to the brain as well as to transmit motor impulses down the descending tracts away from the brain.24 The second primary function of the spinal cord is to house and regulate spinal reflexes. Receipt of sensory impulses may cause a reaction anywhere in the body; alternatively, the signal might be stored in the memory to be used at some stage in the future. Within the motor division of the nervous system the spinal cord helps to control the various bodily activities, including skeletal muscle activity, smooth muscle activity and secretion by both endocrine and exocrine glands.
Sensory neurons from all over the skin, except for the skin of the face and scalp, feed information into the spinal cord through the spinal nerves. The skin surface can be mapped into distinct regions that are supplied by a single spinal nerve19 (see Figure 16.11).25 Each of these regions is called a dermatome. Sensation from a given dermatome is carried over its corresponding spinal nerve. This information can be used to identify the spinal nerve or spinal segment that is involved in an injury. In some areas, the dermatomes are not absolutely distinct. Some dermatomes may share a nerve supply with neighbouring regions. For this reason, it is necessary to numb several adjacent dermatomes to achieve successful anaesthesia.
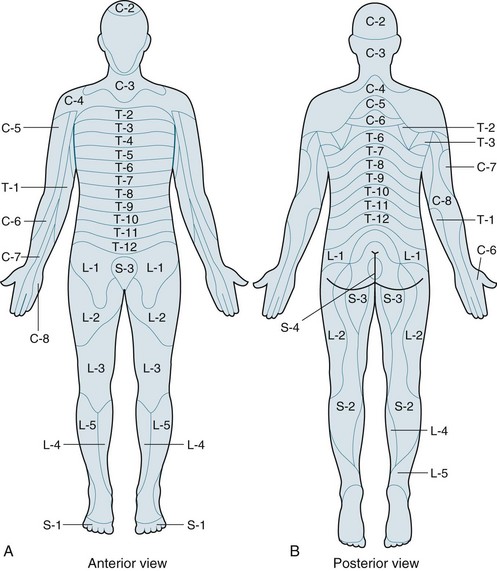
FIGURE 16.11 (A) Anterior and (B) posterior distributions of dermatomes on the surface of the skin.25
The blood supply to the spinal cord arises from branches of the vertebral arteries and spinal radicular arteries.19 The midthoracic region, at approximately T4–T8, lies between the lumbar and vertebral arterial supplies and is a vulnerable zone of relatively decreased perfusion. This region is most susceptible to infarction during periods of hypotension, thoracic surgery or other conditions, causing decreased aortic pressure and potentially leading to ischaemic spinal injury with devastating consequences.19
Peripheral Nervous System
Motor Control
Movements can be divided into three main classes: voluntary activity, rhythmic motor patterns, and reflex responses. The highest-order activity is voluntary movement, which allows for expression of the will and a purposeful response to the environment (e.g. reading, speaking, performing calculations).1 Such activity is goal-directed and largely learned, and improves with practice. In rhythmic motor patterns, the initiation and termination may be voluntary, but the rhythmic activity itself does not require conscious participation (e.g. chewing, walking, running). Reflex responses are simple, stereotyped responses that do not involve voluntary control (e.g. deep tendon reflexes or withdrawal of a limb from a hot surface). Motor control is carried out in a hierarchical yet parallel fashion in the cerebral cortex, the brainstem and the spinal cord. Modulating influences are provided by the basal ganglia and cerebellum through the thalamus.1
Sensory Control
The somatic sensory system has two major components: a subsystem for the detection of mechanical stimuli (e.g. light touch, vibration, pressure, cutaneous tension), and a subsystem for the detection of painful stimuli and temperature.1 Together, these subsystems give the ability to identify the shapes and textures of objects, to monitor the internal and external forces acting on the body at any moment, and to detect potentially harmful circumstances. Mechanosensory processing of external stimuli is initiated by the activation of a diverse population of cutaneous and subcutaneous mechanoreceptors at the body surface that relays information to the central nervous system for interpretation and ultimately for action. Additional receptors located in muscles, joints and other deep structures monitor mechanical forces generated by the musculoskeletal system, and are called proprioceptors. Mechanosensory information is carried to the brain by several ascending pathways that run in parallel through the spinal cord, brainstem and thalamus to reach the primary somatic sensory cortex in the postcentral gyrus of the parietal lobe.1 The primary somatic sensory cortex projects in turn to higher-order association cortices in the parietal lobe, and back to the subcortical structures involved in mechanosensory information processing.
Autonomic Nervous System
The autonomic nervous system, with its three major divisions (sympathetic, parasympathetic and enteric), is largely an involuntary system and is part of the efferent division, as we saw in Figure 16.1. It allows the body to adjust to rapidly changing external events (the ‘flight or fight’ response of the sympathetic division), and to regulate internal activities (blood pressure, temperature, airway and breathing, urinary function, digestion by the parasympathetic and enteric divisions).1 Whereas the major controlling centres for somatic motor activity are the primary and secondary motor cortices in the frontal lobes and a variety of related brainstem nuclei, the major locus of central control in the visceral motor system is the hypothalamus and the complex circuitry that it controls in the brainstem tegmentum and spinal cord.1 The status of both divisions of the visceral motor system is modulated by descending pathways from these centres to preganglionic neurons in the brainstem and spinal cord, which in turn determine the activity of the primary visceral motor neurons in autonomic ganglia. The postganglionic neurons of the sympathetic system, with few exceptions, act on their effectors by releasing the neurotransmitter adrenaline and the related compound noradrenaline. This system is therefore described as adrenergic, which means ‘activated by adrenaline’.1 The autonomic regulation of several organ systems of particular importance in clinical practice is illustrated in Figure 16.12.15
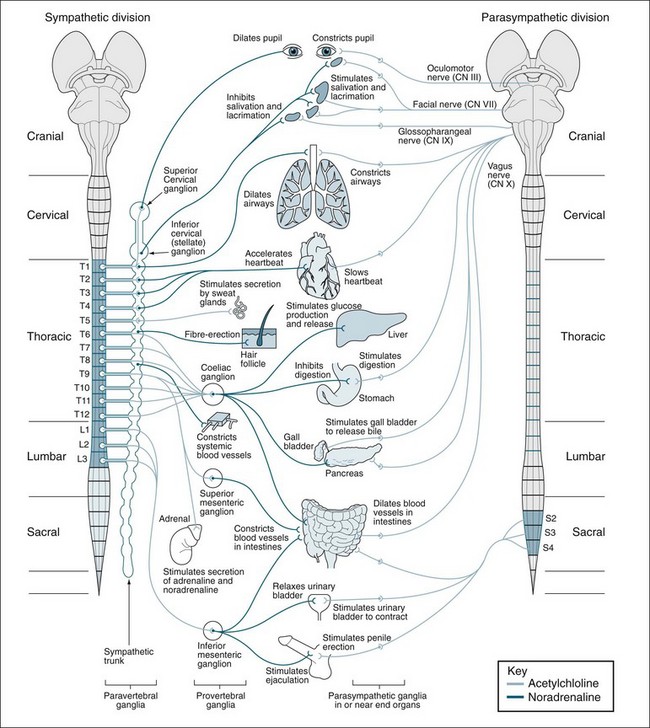
FIGURE 16.12 Sympathetic and parasympathetic divisions of the autonomic nervous system. Sympathetic outputs (left) arise from thoracolumbar spinal cord segments and synapse in paravertebral and prevertebral ganglia. Parasympathetic outputs (right) arise from craniosacral regions and synapse in ganglia in or near effector organs.15
Neurological Assessment and Monitoring
Physical Examination
Conscious State
Arousal assessment
The evaluation of arousal focuses on the ability to be able to respond to a variety of stimuli and can be described using the AVPU scale or terms such as disorientated, lethargic, or obtunded. The advanced trauma life support course26 recommends an initial assessment during initial resuscitation based on the response to stimulation: Awake, Verbal, Pain, Unresponsive (AVPU). Observe the patient’s response (verbal or motor). If there is no response to voice or light touch, painful stimulus is needed to assess neurological status. Central pain should be used first and applied with care. Sternal rub, supraorbital pressure (least used), trapezius pinch (most used) or pinching the fleshy portion of the upper arm near the axilla are methods for introducing central pain. Hand grasp is a reflex and is a poor test for motor strength. If the patient does not respond to verbal stimulus but moves spontaneously in a purposeful manner (picks at linen, pulls at tubes), the patient is localising. Painful stimulus is not required if spontaneous localisation has been observed. Watch for symmetry. Localising is purposeful and intentional movement intended to eliminate a noxious stimulus, whereas withdrawal is a ‘smaller’ movement used to ‘get away from’ noxious stimulus. Abnormal flexion differs from withdrawal in that the flexion is rigid and abnormal looking. Abnormal extension is a rigid movement with extension of the limbs.
Assessment of awareness
If arousable, progress to assessment of awareness using the Glasgow Coma Scale (GCS). Teasdale and Jennett27 designed the GCS to establish an objective, quantifiable measure to describe the prognosis of a patient with a brain injury and include scoring of separate subscales related to eye opening, verbal response and motor response (Table 16.7). Originally, the GCS was developed as three separate response areas and reported as such. Contemporary use of the GCS automatically adds the three best response scores and easily loses the information given from the separate response areas. Reporting the GCS as three numbers and then the total gives a broader assessment interpretation.
TABLE 16.7 Glasgow Coma Scale
The Glasgow Coma Scale is scored between 3 and 15, 3 being the worst, and 15 the best. It comprises three parameters: best eye response, best verbal response and best motor response. The definition of these parameters is given below.
The Glasgow Coma Scale for adults | Paediatric version of the Glasgow Coma Scale |
---|---|
Eye and pupil assessment
The immediate constriction of the pupil when light is shone into the eye is referred to as the direct light reflex. Withdrawal of the light should produce an immediate and brisk dilation of the pupil. Introduction of the light into one eye should cause a similar constriction to occur in the other pupil (consensual light reaction).28
Other points to consider when conducting pupillary observations include the following:29
• pinpoint non-reactive pupils are associated with opiate overdose
• non-reactive pupils may also be caused by local damage
• atropine will cause dilated pupils
• one dilated or fixed pupil may be indicative of an expanding or developing intracranial lesion, compressing the oculomotor nerve on the same side of the brain as the affected pupil
• A sluggish pupil may be difficult to distinguish from a fixed pupil and may be an early focal sign of an expanding intracranial lesion and raised intracranial pressure. A sluggish response to light in a previously reacting pupil must be reported immediately.
Assessment of pupillary function focuses on three areas: (1) estimation of pupil size and shape; (2) evaluation of pupillary reaction to light; and (3) assessment of eye movements. Metabolic disturbances rarely cause pupillary changes, so abnormal pupillary findings are usually due to a nervous system lesion.30 Irregular-sized pupils are normal for some people and eye prostheses are common so it is important to establish and document these findings so a trend can be established to determine normal from altered states.
Eye and eyelid movements
Patients who are comatose will exhibit no eye opening. In patients with bilateral thalamic damage, there may be normal consciousness, but an eye opening apraxia may mimic coma. If the patient’s eyes are closed, the clinician should gently raise and release the eyelids. Brisk opening and closing of the eyes indicates that the pons is grossly intact. If the pons is impaired, one or both eyelids may close slowly or not at all. In the patient with intact frontal lobe and brainstem functioning, the eyes, when opened, should be pointed straight ahead and at equal height. If there is awareness, the patient should look towards stimuli after eye opening. Eye deviation indicates either a unilateral cerebral or brainstem lesion. If the eyes deviate laterally, gently turn the head to see if the eyes will cross the midline to the other side. A pattern of spontaneous, slow and random movements (usually laterally) is termed roving-eye movements. This indicates that the brainstem oculomotor control is intact but awareness is significantly impaired.31
Limb movement
Assessment of extremities and body movement (or motor response) provides valuable information about the patient with a decreased level of consciousness.32 The clinician must observe the patient’s spontaneous movements, muscle tone, and response to tactile stimuli. Decorticate (flexor) posturing is seen when there is involvement of a cerebral hemisphere and the brain stem. It is characterised by adduction of the shoulder and arm, elbow flexion, and pronation and flexion of the wrist while the legs extend. In terms of the GCS motor score, the withdrawal flexor scores a higher (4/6) than a spastic flexor movement (3/6). Decerebrate (extensor) posturing is seen with severe metabolic disturbances or upper brainstem lesions. It is characterised by extension and pronation of the arm(s) and extension of the legs. Patients may have an asymmetrical response and may posture spontaneously or to stimuli.
Motor tone is first assessed by flexing the limbs and noting increased or absent tone.33 If no tone is present, the hand is lifted approximately 30 cm above the bed and carefully dropped while protecting the limb from injury. The test is repeated with all extremities. Typically, the lower the level of consciousness, the closer to flaccid the limb(s) will be. An asymmetrical examination may indicate a lesion in the contralateral hemisphere or brainstem.
If a noxious stimuli is applied centrally through a sternal rub, trapezius pinch or supraorbital nerve pressure and the patient moves an extremity, it is an indication of brain involvement in the movement and not a spinal reflex.34 The movement should be noted as normal, decorticate (flexor: either withdrawal or spastic) or decerebrate (extensor) and documented accordingly. It should be noted that careful consideration should be given to the choice of noxious stimuli with trapezius pinch the preferred choice as both sternal rub and supraorbital nerve pressure can be traumatic when applied. In ventilated patients, endotracheal suction can also be a substitute for a central noxious stimulus, but the choice of stimulus needs to be consistent.
Corneal reflexes
The corneal reflex is assessed by holding the patient’s eye open and lightly stimulating the cornea.35 The stimuli should result in a reflexive blink, best seen in the lower eyelid. The traditional assessment technique involves using a wisp of cotton, lightly brushed along the lower aspect of the cornea. An alternative, and less potentially traumatic, method is to gently instil isotonic eye drops or saline irrigation ampoules onto the cornea. This reflex is dependent upon CN V for its sensation and CN VII for its motor response. Loss of this reflex is indicative of lower brainstem damage, but may be absent due to trauma, surgery, or long-term contact lens usage.
Oropharyngeal reflexes
The oropharyngeal reflexes are controlled by CN IX and CN X.36 The gag reflex is elicited by lightly stimulating the soft palate with a suction catheter or tongue blade. Clinicians should always avoid stimulating a gag reflex by wiggling the endotracheal tube because doing so may result in an inadvertent extubation. A gag reflex is a forceful, symmetrical lowering of the soft palate. The cough reflex is usually assessed only in patients with an endotracheal tube. This reflex is elicited by gently passing a suction catheter through the tube and stimulating a cough. Loss of these reflexes is indicative of lower brainstem damage.37
Post Traumatic Amnesia Scale
Posttraumatic amnesia (PTA) is a disorder after brain injury that is classified as a traumatic delirium and may even be found in patients who rate a GCS of 15.38 The incidence of delirium after a brain injury event is high especially with severe injuries and loss of consciousness. Delirium is discussed in detail in Chapter 7, however, traumatic delirium historically has been referred to in the literature as posttraumatic amnesia. Posttraumatic amnesia is defined as the ‘time elapsed from injury until recovery of full consciousness and the return of ongoing memory’.39,p.841 It is the initial stage of recovery from brain injury and is characterised by anterograde (formation of new memory) and retrograde (memory before injury) amnesia, disorientation and rapid forgetting. Brief periods of PTA can occur after minor concussion and may be the only clinical sign of any brain injury. This is where PTA is useful for defining severity of injury and alert the clinician in regard to greater surveillance and investigation as described in Table 16.6. Patients often progress directly from coma into delirium without a clearly-defined stupor stage, so using a tool to measure PTA can be useful to gauge the actual condition of the patient in the delirium state. Duration of PTA is extremely variable, ranging from minutes to months. Although the early stages of PTA are easily recognised, identifying the end point is difficult and complex.40
The duration of PTA is the best indicator of the extent of cognitive and functional deficits after TBI. In Australia, the most common means of assessing PTA is the Westmead PTA scale.41 In this scale, four pictures, one with the examiner’s face and name, are to be recalled by the patient on the next day. Those with severe PTA will have difficulty recalling such short-term memory tasks. Often, patients will have a GCS of 15 but have moderate to severe PTA and can be overlooked by inexperienced clinicians who fail to watch for secondary insults. The duration of PTA correlates well with the extent of diffuse axonal injury and with functional outcomes. For example, one study found that 80% of patients with a PTA duration of less than 2 weeks had a good recovery, compared with 46% for those with a PTA duration between 4 and 6 weeks.42 A person is said to be absolved of PTA if they can achieve a perfect score for three consecutive days.
Assessment of the Injured Brain
The primary aim of managing patients with acute brain injury in the critical care unit is to maintain cerebral perfusion and oxygenation.43 There is little that can be done to reverse the primary damage caused by an insult. Secondary insults may be subtle and can remain undetected by routine systemic physiological monitoring. Continuous monitoring of the central nervous system in the ICU serves three functions:44
1. determine the extent of the primary injury
2. early detection of secondary cerebral insults so that appropriate interventions can be instituted
3. monitoring of therapeutic interventions to provide feedback.
Brain Imaging Techniques
Computed tomography
CT is the primary neuroimaging technique in the initial evaluation of the acute brain injury patient and uses a computer to digitally construct an image based upon the measurement of the absorption of X-rays through the brain. Table 16.8 generally summarises the white to black intensities seen for selected tissues in CT. The advantages of CT are: (1) it is rapidly done, which is especially important in neurological emergencies; (2) it clearly shows acute and sub-acute haemorrhages into the meningeal spaces and brain; and (3) it is less expensive than a MRI.45 Disadvantages include: (1) it does not clearly show acute or sub-acute infarcts or ischaemia, or brain oedema, only established injury; (2) it does not clearly differentiate white from grey matter as clearly as an MRI; and (3) it exposes the patient to ionising radiation. Despite these limitations it is still the most prevalent form of neurological imaging.46
Structure/Fluid/Space | Grey Scale |
---|---|
Magnetic resonance imaging
The tissues of the body contain proportionately large amounts of protons in the form of hydrogen and function like tiny spinning magnets. Normally, these atoms are arranged randomly in relation to each other due to the constantly changing magnetic field produced by the associated electrons. Magnetic Resonance Imaging (MRI) uses this characteristic of protons to generate images of the brain and body. The advantages of MRI are: (1) it can be manipulated to visualise a wide variety of abnormalities within the brain; and (2) it can show a great deal of detail of the brain in normal and abnormal states.47 The disadvantages of MRI are: (1) it does not show acute or sub-acute haemorrhage into the brain in any detail; (2) the time frame and enclosed space required to perform and prepare a patient for the procedure is not advantageous for neurological emergencies; (3) relatively more expensive compared to CT; (4) the loud noise of the procedure needs to be considered in the patient management; and (5) equipment for life support and monitoring needs to be non-magnetic due to the magnetic nature of the procedure.48
Functional magnetic resonance imaging
Functional magnetic resonance imaging (fMRI) is similar to MRI but uses deoxyhaemoglobin as an endogenous contrast, and serves as the source of the magnetic signal for fMRI. It can determine precisely which part of the brain is handling critical functions such as thought, speech, movement and sensation, help assess the effects of stroke, trauma or degenerative disease on brain function, monitor the growth and function of brain tumours and guide the planning of surgery or radiation therapy for the brain.49
Cerebral angiography
Cerebral angiography involves cannulation of cerebral vessels and the administration of intraarterial contrast agents and medications for conditions involving the arterial circulation of the brain. This procedure also has the benefit of using non-invasive CT or MRI with or without contrast to guide the accuracy of the procedure. For example, intracranial aneurysms and arteriovenous malformations can be accurately diagnosed and repaired without surgical intervention.50
Cerebral perfusion imaging techniques
Numerous imaging techniques have been developed and applied to evaluate brain haemodynamics, perfusion and blood flow. The main imaging techniques dedicated to brain haemodynamics are positron emission tomography (PET), single photon emission computed tomography (SPECT), xenon-enhanced computed tomography (XeCT), dynamic perfusion computed tomography (PCT), MRI dynamic susceptibility contrast (DSC) and arterial spin labelling (ASL). All these techniques give similar information about brain haemodynamics in the form of parameters such as CBF or CBV.51 They use different tracers and have different technical requirements. Some are feasible at the bedside and others not (see Table 16.9). The duration of data acquisition and processing varies from one technique to the other. Brain perfusion imaging techniques also differ by quantitative accuracy, brain coverage and spatial resolution.52
Figure 16.13 is a scan from a traumatic brain injury patient and demonstrates a brain perfusion scan radionuclide imaging. In the image the cerebral cortex is dark, indicative of no CBF or perfusion confirming brain death.
Intracranial Pressure Monitoring
Invasive measures for monitoring intracranial pressure (ICP) are commonly used in patients with a severe head injury or after neurological surgery. Normal ICP varies with age, body position, and clinical condition. The normal ICP is 7–15 mmHg in a supine adult, 3–7 mmHg in children, and 1.5–6 mmHg in term infants. The definition of intracranial hypertension depends on the specific pathology and age, although ICP >15 mmHg is generally considered to be abnormal. Increased ICP causes a critical reduction in CPP and CBF and may lead to secondary ischaemic cerebral injury. A number of studies have shown that high ICP is strongly associated with poor outcome, particularly if the period of intracranial hypertension is prolonged.53 ICP is not a static pressure and varies with arterial pulsation, with breathing and during coughing and straining. Each of the intracranial constituents occupies a certain volume and, being essentially liquid, is incompressible. ICP cannot be reliably estimated from any specific clinical feature or CT finding and must actually be measured. Different methods of monitoring ICP have been described but two methods are commonly used in clinical practice: intraventricular catheters and intraparenchymal fibreoptic microtransducer systems.
Currently, ventriculostomy is the most accurate (although the intraparenchymal fibreoptic is now similar in accuracy), cost-effective and reliable method of monitoring ICP and is associated with low infection risks if the duration of placement is less than 72 hours.54 The ventriculostomy catheter is part of a system that includes an external drainage system and a transducer. The drainage system and transducer are primed on insertion with preservative-free saline. The transducer can easily be calibrated or zeroed against a known pressure. Advantages of using an indwelling ventricular catheter include allowing CSF drainage to effectively decrease ICP and using the catheter as a means to instil medications. Access to CSF drainage allows serial laboratory tests of CSF and determination of volume–pressure relationships. Disadvantages of ventriculostomy include risk of infection, which is higher than that associated with other ICP-monitoring techniques.55 In addition, the catheter may become occluded with blood or tissue debris, interfering with CSF drainage or ICP monitoring. Also, if significant cerebral oedema is present, locating the lateral ventricle for insertion of the ventriculostomy catheter may be difficult. Importantly, bleeding or ventricular collapse may occur if CSF is drained too rapidly.56 For this last reason, many clinicians set the ventriculostomy drainage system to drain CSF when the ICP is greater than 15–20 mmHg by adjusting the height of the drip chamber. In addition, a limit of ventricular drainage per hour using gravity and three-way taps to 5–10 mL/h has been used to avoid excessively rapid CSF drainage. Using a ventriculostomy may allow lifesaving CSF drainage and control of intracranial hypertension and secondary injury.57
Whilst routine ICP monitoring is widely accepted as a mandatory monitoring technique for management of patients with severe head injury and is a guideline suggested by the Brain Trauma Foundation, there is some debate over its efficacy in improving outcome from severe TBI.58 A review of neurocritical care and outcome from TBI suggested that ICP/cerebral perfusion pressure (CPP)-guided therapy may benefit patients with severe head injury, including those presenting with raised ICP in the absence of a mass lesion and also patients requiring complex interventions.59
Pulse waveforms
Interpretation of waveforms that are generated by the cerebral monitoring devices is important in the clinical assessment of intracranial adaptive capacity (the ability of the brain to compensate for rises in intracranial volume without raising the ICP).60 Brain tissue pressure and ICP increase with each cardiac cycle and, thus, the ICP waveform is a modified arterial pressure wave. See Figure 16.14. The cardiac waves reach the cranial circulation via the choroid plexus and resemble the waveforms transmitted by arterial catheters, although the amplitude is lower.
There are three distinct peaks seen in the ICP waveform:61
• P1: the percussion wave, which is sharp and reflects the cardiac pulse as the pressure is transmitted from the choroid plexus to the ventricle;
• P2: the tidal wave, which is more variable in nature and reflects cerebral compliance and increases in amplitude as compliance decreases;
• P3: which is due to the closure of the aortic valve and is known as the dicrotic notch. Of recent importance is that the elevation of the P3 may indicate low global cerebral perfusion.62
Assessment of Cerebral Perfusion
CPP is a pressure-based indicator of oxygen and metabolite delivery. There is no evidence for the optimum level of CPP, but 70–80 mmHg is probably the critical threshold in adults especially those who are pressure-active (i.e. ICP varies inversely with MAP).63 Higher CPP has been associated with increased lung water and acute respiratory distress syndrome. Furthermore, mortality rises approximately 20% for each 10 mmHg loss of CPP. In those studies where CPP was maintained above 70 mmHg, the reduction in mortality was as much as 35% for those with severe head injury.64 The Brain Trauma Foundation recommends a CPP goal of 50–70 mmHg despite the lack of definitive data, such as from randomised controlled trials and intention-to-treat clinical trials.65 In the paediatric population a CPP >40 mmHg is the recommended guideline.66,67 Utilising cerebral oxygenation monitoring in combination with pressure has been associated with better outcomes for brain-injured patients, and is part of the multimodal assessment for brain injury.
Assessment of Cerebral Oxygenation
Jugular Venous Oximetry
Jugular venous catheterisation is used for deriving oxygen based variables.68 It facilitates the assessment of jugular venous oxygenation (SjvO2), cerebral oxygen extraction (CEO2), and arteriovenous difference in oxygen (AVDO2). All of these variables indicate changes in cerebral metabolism and blood flow, and therefore the catheter generates continuous data that reflect the balance between supply and demand of cerebral oxygen.
The normal requirement for cerebral oxygen delivery is consumption at 35–40% of available oxygen, giving a normal SjvO2 of 60–65%. Changes in SjvO2 reflect changes in cerebral metabolic rate and cerebral blood flow; however, as it is a global measure it does not detect regional ischaemia. A high SjvO2 is indicative of increased cerebral blood flow, reduced oxygen consumption, and hyperventilation. Low SjvO2 levels suggest that cerebral perfusion is reduced, with levels below 40% indicative of global cerebral ischaemia.69 However, caution must be used when interpreting values generated using this method, as high values might also imply an increase in arteriovenous shunting secondary to vasoconstriction, maldistribution of blood flow or lack of oxygen consumption as in brain death. Because SjvO2 monitoring is a global measure of cerebral oxygenation,70 smaller areas of ischaemia are not detected unless these are of sufficient magnitude to affect global brain saturation. SjvO2 requires special care such as frequent recalibration to ensure accurate measurements, observing for catheter migration that interferes with signal quality, and often, medical intervention is required to reposition the catheter. The position of the patient also affects signal quality, and ideally the patient should be nursed supine with a head elevation of 10–15° and at least a neutral head alignment. It is important that measurement errors be excluded when abnormal readings are noted; algorithms have been developed to assist nurses when caring for patients with jugular bulb oximetry.71
Partial Brain Tissue Oxygenation Monitoring
Changes in ICP values alone do not accurately depict poor cerebral blood flow or oxygenation deficits to brain tissue. Consequently brain tissue hypoxaemia is often observed during the first 24 hours after injury despite controlled brain pressures. Monitoring partial pressure of oxygen in brain tissue (PbtO2) can be used to collect more accurate and timely information about cerebral oxygen delivery and demand than ICP allows. A tissue oxygen value of less than 10 mmHg for more than 10 minutes carries a higher risk of death. Normal brain oxygen levels (PbtO2 between 20 and 25 mmHg) emerge as a critical determinant of outcome, with values below 20 mmHg carrying a higher risk.69
Regardless of ICP, brain tissue oxygenation falls with a decrease in cerebral blood flow below an ischaemic threshold of 18 mL/100 g/min. ICP may respond to the changes but often several hours later when the damage can not be reversed. Alterations in cerebral metabolic rate can also change tissue oxygen levels. Reducing the patient’s energy consumption via reduced noise and/or distractions, and increasing their protein caloric intake to complement their increased stress state can improve tissue oxygenation.72
Microdialysis
Cerebral microdialysis (using a catheter ideally placed in the frontal lobe) is a tool for investigating the metabolic status of the injured brain and is part of multimodal monitoring. The microdialysis probe is inserted into the cerebral tissue where substances in the extracellular fluid surround the semipermeable membrane at the tip of the catheter. Following equilibration of the tissue metabolites with the perfusion fluid, the dialysate can be analysed for concentrations of products of energy metabolism (glucose, lactate, pyruvate) as indicators of hypoxia and ischaemia. In addition, interstitial glycerol can be determined, which is a parameter of lipolysis and/or cell membrane damage. In theory, the microdialysis catheter acts like a blood capillary.73 Thereby, it is proposed that microdialysis provides information regarding events that take place in the tissue before any chemical events are reflected by changes in systemic blood levels of indicator substances.74 These molecules diffuse across the membrane part of the catheter and equilibrate with the perfusion fluid, which is pumped through the probe at very low rates of flow. Changes in the concentration of a substrate in the surrounding milieu are reflected by subsequent changes in the dialysate.75 Rather than inserting an instrument into the tissue, microdialysate is extracted and later analysed in the laboratory or clinically at the patient’s bedside.
Non-Invasive Assessment
Transcranial Doppler
Transcranial Doppler (TCD) ultrasound has proven to be a safe, reliable and relatively inexpensive technology for measuring cerebrovascular blood velocities and evaluating cerebral circulation and haemodynamics. Pulses of ultrasound are directed using a handheld transducer towards the vascular formations in the base of the skull. Velocities from the cerebral arteries, the internal carotids, the basilar and the vertebral arteries can be sampled by altering transducer location, angle and the instrument’s depth setting. The commonest windows in the cranium are located in the orbit (of the eye), and in the temporal and suboccipital regions. TCD measures systolic, diastolic and mean middle cerebral artery (MCA) flow velocities and a derived value, the pulsatility index (PI). Changes in the PI can be used to identify the threshold of autoregulation or cerebral perfusion pressure break point in individual patients. In subarachnoid haemorrhage (SAH) and TBI this may be due to vasospasm, or impaired autoregulation or abnormal intracranial compliance. TCD is a simple, portable and non-invasive tool, well suited to serial monitoring, that can be used at the bedside to detect relative changes in CBF in brain-injured patients.76
Continuous Electroencephalography
Electroencephalography (EEG) is the recording of electrical activity by sensors along the scalp produced by the firing of neurons within the brain. Continuous EEG (cEEG) has the advantage of being continuous, noninvasive and carrying the potential to detect alterations in brain physiology at a reversible stage, which may trigger treatment before permanent brain injury occurs. The invention of digital EEG has made cEEG monitoring feasible for ICU patients.77 Currently, the main applications of cEEG are diagnosing nonconvulsive status epilepticus, monitoring and guiding the treatment of status epilepticus and detecting delayed cerebral ischaemia from vasospasm in subarachnoid haemorrhage patients. Other applications may include monitoring of reperfusion after tissue plasminogen activator in acute stroke patients and detection of intracranial hypertension. Clinically unrecognised electrographic seizures and periodic epileptiform discharges have been shown to be frequent and associated with poor outcome in patients with severe brain injury from different aetiologies, including TBI, ischaemic and haemorrhagic strokes and CNS infection.78 The EEG becomes substantially abnormal (suppressed) when cerebral blood flow declines to 20–30 mL/100 g/min. More subtle abnormalities accompany lesser degrees of hypoperfusion, including initial loss of beta activity, slowing to the theta range, and then to the delta range. Irreversible injury to brain tissue occurs at cerebral flows of about 10–12 mL/100 g/min. Thus, the EEG sensitivity to ischaemia allows its use in situations where cerebral perfusion is at risk.79 To facilitate interpretation, digital EEG data can be transformed into power spectra by fast Fourier transformation. Changes over time in these quantitative EEG (qEEG) parameters can trigger remote reading, focused neurological examination, imaging studies and early treatment. Subtle EEG changes may be difficult to interpret in isolation, but may be better understood when interpreted in concert with other components of a multimodality monitoring paradigm, which may include microdialysis, brain tissue oxygen and cerebral perfusion pressure.
Near-Infrared Spectroscopy
The clinical and bedside use of NIRS is constrained by potential sources of error, which include contamination of the signal by the extracerebral circulation (such as in the scalp), extraneous light, and the presence of extravascular blood arising from subarachnoid or subdural haemorrhage.80 In a recent study in patients with subarachnoid haemorrhage, episodes of angiographic cerebral vasospasm were strongly associated with reduction in trend in the ipsilateral NIRS signal.81 Furthermore, the degree of spasm (especially more than 75% vessel diameter reduction) was associated with a greater reduction in same-side NIRS signal demonstrating real-time detection of intracerebral ischaemia.
Summary
Case study
Emergency Department
Primary survey
Daniel’s primary survey revealed the following details.
• Airway: Upper airway cleared. Cervical spine: Status unknown, collar in situ.
• Breathing: Hand ventilated on 12 L/min at 14 breaths/min, paradoxical chest rise and fall, generalised poor air entry, decreased bilaterally, no tracheal deviation.
• Circulation: Tachycardic, hypotensive, and hypovolaemic; pulses present on palpation except for the right popliteal and dorsalis pedis; temperature centrally warm, well perfused, skin pink in colour, peripherally cold and pale; capillary refill >4 seconds.
• Disability: He was unresponsive, GCS 3; pupils: left 3 mm and right 5 mm non-reacting. Daniel was intubated using thiopentone, fentanyl and rocuronium and mechanically ventilated in combination with physiological fluid resuscitation.
Secondary survey
The secondary survey revealed the following details.
• Head: scalp clear, nil abrasions. CT revealed widespread petechial haemorrhages consistent with diffuse axonal injury, acute subdural haemorrhage with midline shift of the ventricles, hairline base of skull fracture and cerebral oedema with poor differentiation between grey and white matter.
• Face: No oedema; rhinorrhoea and otorrhoea from both nostrils and ears.
• Neck: Stiff neck collar left in situ; no obvious lacerations observed around neck area; no evidence of tracheal deviation. Cervical spine CT reported no bony injury, spine not cleared; with a stable L4 pedicle fracture.
• Chest: Obvious chest deformity and instability of sternum and ribs; paradoxical chest rise and expansion; decreased air entry bilaterally, no subcutaneous emphysema. Bilateral pulmonary contusions, left haemopneumothorax diagnosed on review of the chest X-ray; a left sided chest drain was inserted.
• Abdomen: Firm, no abnormal distension, some bruising. IDC insertion revealed haematuria.
• Pelvis: Bruising bilaterally with no obvious deformity.
• Back: Marked flank bruising, no lacerations, right perinephric haematoma on CT; rectal tone present. Upper limbs: Obvious deformity of right arm; X-ray revealed right radial and ulna fractures, lacerations and bruising present; pulses present.
• Lower Limbs: Bilateral femur fractures – right one compound; lacerations and extensive bruising; pulses absent right side.
Emergency surgery
Daniel received a massive blood transfusion:
• 34 units packed red blood cells
• 17 units fresh frozen plasma
Following surgery, he was admitted to the Intensive Care Unit (ICU) for further management
ICU management
Days 2–7
Daniel’s clinical parameters and assessment are shown in Table 16.10. His condition remained variable and on days 3 and 4 his ICP and CPP were unstable with increasing need for sedation and paralysis. His pupils enlarged to size 5 and became unreactive. He was stabilised with boluses of hypertonic saline and increased drainage from the EVD which totalled 35 mL for the day. A repeat CT determined a diffuse injury with global cerebral oedema. The ventricles were effaced but not compressed. After stabilising on day 4, Daniel’s sedation was turned off the morning of day 5 for neurological assessment. His GCS was 5 (E2 V1(T)M3) with normal flexion to pain and remained unchanged until day 7. His GCS may have increased but it was difficult to assess his verbal response whilst intubated. The EVD was removed on day 6 and he remained sedated and ventilated to support his chest injuries. Daniel continued to slowly recover.
Learning activities
1. What effect would decreasing the concentration of extracellular potassium ions have on the transmembrane potential of a neuron?
2. Which brain structure coordinates endocrine and nervous system activities?
3. Which component of the brain controls the cardiac centres, the vasomotor centres and the respiratory rhythm centre?
4. What information does the GCS provide? What does GCS predict?
5. During the testing of motor response a noxious stimulus is applied to the nail bed of the middle finger. The unconscious patient elicits a flexion withdrawal response of the wrist, arm and shoulder. Explain what this response means in terms of central or peripheral response.
6. What is the pathophysiological basis for the rise in ICP? How would this manifest on the ICP waveform?
7. Explain the physiological mechanism for dilated (size 5), non reactive pupils.
8. A patient recovering from a subarachnoid haemorrhage can not remember events prior to the haemorrhage event. What type of amnesia is this?
American Association of Neuroscience Nurses (AANN). http://www.aann.org.
Australasian Neuroscience Nurses’ Association. http://www.anna.asn.au.
The Brain Trauma Foundation. http://www.braintrauma.org.
Brain Explorer. http://brainexplorer.org/.
Brain Injury Association Inc. http://www.biausa.org.
GCS protocol. http://intensivecare.hsnet.nsw.gov.au/five/doc/gcs_R_am_rpa.pdf.
GCS procedure 1. http://www.nursingtimes.net/neurological-assessment-part-3-glasgow-coma-scale/1735582.article.
GCS procedure 2. http://www.nursingtimes.net/neurological-assessment-part-4-glasgow-coma-scale-2/1768984.article.
GCS and use of painful stimulus. http://www.mpdgp.com.au/files/docs/laos%2520recommendations/the%2520use%2520of%2520painful%2520stimulus%2520in%2520relation%2520to%2520glasgow%2520coma%2520scale%2520observations.pdf.
Head Injury Society of New Zealand. http://www.head-injury.org.nz.
Neuroscience tutorials. http://thalamus.wustl.edu/course/.
Neurological Exam. http://www.neuroexam.com/neuroexam/.
Neurological Foundation of New Zealand. http://www.neurological.org.nz/.
Official Journal of the American Academy of Neurology (AAN). http://neurology.org/.
Physical Examination and Neurological Assessment. http://www.neurologyexam.com/.
Post traumatic amnesia protocol. http://www.psy.mq.edu.au/pta/.
Rural Neurotrauma Assessment. http://www.racs.edu.au/media/16138/PUB_090824_-_Neurotrauma_(Standard_Version).pdf.
1 Martini F, Nath J. Anatomy and physiology, 8th edn. San Francisco: Pearson Benjamin Cummings; 2006.
2 Guyton A, Hall J. Textbook of medical physiology, 12th edn. Philadelphia: Elsevier Saunders; 2010.
3 Porth C. Pathophysiology concepts of altered health states, 8th edn. Philadelphia: Lippincott, Williams & Wilkins; 2008.
4 Perlson E, Maday S, Fu MM, Moughamian AJ, Holzbaur EL. Retrograde axonal transport: pathways to cell death? Trends Neuroscience. 2010;33(7):335–344.
5 Purves D, Augustine G, Hall W, LaMantia A, McNamara J, White L. Neuro-science, 4th edn. New York: Sinauer Associates; 2007.
6 Byku M, Macarthur H, Westfall TC. Inhibitory effects of angiotensin-(1–7) on the nerve stimulation-induced release of norepinephrine and neuropeptide Y from the mesenteric arterial bed. Am J Physiol Heart Circ Physiol. 2010;298(2):457–465.
7 Rosas-Ballina M, Tracey KJ. Cholinergic control of inflammation. J Intern Med. 2009;265(6):663–679.
8 Parkhurst C, Gan W. Microglia dynamics and function in the CNS. Current Opinion in Neurobiology. 2010;20(4):474–480.
9 Graeber MB, Streit WJ. Microglia: biology and pathology. Acta Neuropathol. 2010;119(1):89–105.
10 Dziedzic T, Metz I, Dallenga T, König FB, Müller S, et al. Wallerian degeneration: a major component of early axonal pathology in multiple sclerosis. Brain Pathol. 2010;20(5):976–985.
11 Hoffman-Kim D, Mitchel JA, Bellamkonda RV. Topography, cell response, and nerve regeneration. Annu Rev Biomed Eng. 2010;15(12):203–231.
12 Miller BT, Fegen D, Vytlacil J, Pradhan S, D’Esposito M. Prefrontal Cortex Tunes Category Selectivity in Visual Association Cortex. J Cogn Neurosci. 2010. In press
13 Willems RM, Toni I, Hagoort P, Casasanto D. Neural dissociations between action verb understanding and motor imagery. J Cogn Neurosci. 2010;10:2387–2400.
14 Guillery RW. Anatomical pathways that link perception and action. Prog Brain Res. 2005;149(28):235–256.
15 Blumenfeld H. Neuroanatomy through clinical cases. New York: Sinauer Associates; 2010.
16 Elliott D, Khan MA. Vision and goal-directed movement: neurobehavioral perspectives. Champaign, IL: Human Kinetics; 2010.
17 Szymusiak R. Hypothalamic versus neocortical control of sleep. Curr Opin Pulm Med. 2010;16(6):530–535.
18 Bostan AC, Strick PL. The cerebellum and basal ganglia are interconnected. Neuropsychol Rev. 2010;20(3):261–270.
19 Edwards IJ, Deuchars SA, Deuchars J. The intermedius nucleus of the medulla: a potential site for the integration of cervical information and the generation of autonomic responses. J Chem Neuroanat. 2009;38(3):166–175.
20 Ley S, Weigert A, Brüne B. Neuromediators in inflammation-a macrophage/nerve connection. Immunobiology. 2010;215(9–10):674–684.
21 Veening JG, Barendregt HP. The regulation of brain states by neuroactive substances distributed via the cerebrospinal fluid; a review. Cerebrospinal Fluid Res. 2010;6(7):1.
22 Abbott NJ, Patabendige AA, Dolman DE, Yusof SR, Begley DJ. Structure and function of the blood-brain barrier. Neurobiol Dis. 2010;37(1):13–25.
23 Urden L, Stacy K, Lough M. Thelan’s critical care nursing, diagnosis and management, 5th edn. Philadelphia: Mosby Elsevier; 2010.
24 Holtz A, Levi R. Spinal Cord Injury. New York: Oxford University Press Inc; 2010.
25 Cohen B, Taylor J. Memmler’s human body in health and disease, 11th edn. Philadelphia: Lippincott, Williams & Wilkins; 2008.
26 Deakin CD, Nolan JP, Soar J, Sunde K, Koster RW, et al. European Resuscitation Council Guidelines for Resuscitation 2010. Section 4. Adult advanced life support. Resuscitation. 2010;81(10):1305–1352.
27 Teasdale G, Jennett B. Assessment of coma and impaired consciousness. A practical scale. Lancet. 1974;2(7872):81–84.
28 Zuercher M, Ummenhofer W, Baltussen A, Walder B. The use of Glasgow Coma Scale in injury assessment: A critical review. Brain Injury. 2009;23(5):371–384.
29 Davies S, Lindley A. Chapter 2, Monitoring the injured brain. In: Adams JP, Bell D, McKinlay J. Neurocritical care: a guide to practical management. London: Springer-Verlag Limited, 2010.
30 Jevon P. Neurological assessment part 2 – pupillary assessment. Nurs Times. 2008;104(28):26–27.
31 Bajekal R. Eye signs in anaesthesia and intensive care medicine. Anaesthes Intens Care Med. 2007;8(9):387–388.
32 Kung W, Tsai S, Chiu W, Hung K, Wang S, et al. Correlation between glasgow coma score components and survival in patients with traumatic brain injury. Injury. 2011. In press
33 Healey C, Osler TM, Rogers FB, Healey MA, Glance LG, et al. Improving the Glasgow Coma Scale score: motor score alone is a better predictor. J Trauma. 2003;54(4):671–678.
34 Seel RT, Sherer M, Whyte J, Katz DI, Giacino JT, et al. Assessment scales for disorders of consciousness: Evidence-based recommendations for clinical practice and research. Arch Phys Med Rehabil. 2010;91(12):1795–1813.
35 Pullen RL, Jr. Testing the corneal reflex. Nursing. 2005;35(11):68.
36 Lang IM. Brain stem control of the phases of swallowing. Dysphagia. 2009;24(3):333–348.
37 Widdicombe JG, Addington WR. Cough in patients after stroke. Eur Respir J. 2011;37(1):218.
38 Kosch Y, Browne S, King C, Fitzgerald J, Cameron I. Post-traumatic amnesia and its relationship to the functional outcome of people with severe traumatic brain injury. Brain Injury. 2010;24(3):479–485.
39 Tate RL, Pfaff A, Baguley IJ, Marosszeky JE, Gurka JA, et al. A multicentre, randomised trial examining the effect of test procedures measuring emergence from post-traumatic amnesia. J Neurol, Neurosurg Psych. 2006;77:841–849.
40 Sherer M, Struchen MA, Yablon SA, Wang Y, Nick TG. Comparison of indices of traumatic brain injury severity: Glasgow coma scale, length of coma and post-traumatic amnesia. J Neurol, Neurosurg Psych. 2008;79(6):678–685.
41 Ponsford J, Facem PC, Willmott C, Rothwell A, Kelly A, Nelms R, Ng KT. Use of the Westmead PTA scale to monitor recovery of memory after mild head injury. Brain Injury. 2004;18(6):603–614.
42 Formisano R, Carlesimo GA, Sabbadini M, Loasses A, et al. Clinical predictors and neuropsychological outcome in severe traumatic brain injury patients. Acta Neurochir. 2004;146(5):457–462.
43 Chestnut RM, Marshall SB, Piek J, Blunt BA, Klauber MR, Marshall LF. Early and late systemic hypotension as a frequent and fundamental source of cerebral ischemia following severe brain injury in the Traumatic Coma Data Bank. Acta Neurochir (Wien). 1993;59(Suppl):121–125.
44 Bhatia A, Gupta AK. Neuromonitoring in the intensive care unit. I. intracranial pressure and cerebral blood flow monitoring. Intensive Care Med. 2007;33(7):1263–1271.
45 Bremmer R, De Jong BM, Wagemakers M, Regtien JG, Van Der Naalt J. The course of intracranial pressure in traumatic brain injury: Relation with outcome and ct-characteristics. Neurocritical Care. 2010;12(3):362–368.
46 Downer JJ, Pretorius PM. Symmetry in computed tomography of the brain: The pitfalls. Clin Radiol. 2009;64(3):298–306.
47 Hillary FG, Biswal BB. Automated detection and quantification of brain lesions in acute traumatic brain injury using MRI. Brain Imaging Behavior. 2009;3(2):111–122.
48 Mannion RJ, Cross J, Bradley P, Coles JP, Chatfield D, et al. Mechanism-based MRI classification of traumatic brainstem injury and its relationship to outcome. J Neurotrauma. 2007;24(1):128–135.
49 Leitch JK, Figley CR, Stroman PW. Applying functional MRI to the spinal cord and brainstem. Magn Reson Imaging. 2010;28(8):1225–1233.
50 Greenberg ED, Gold R, Reichman M, John M, Ivanidze J, et al. Diagnostic accuracy of CT angiography and CT perfusion for cerebral vasospasm: A meta-analysis. Am J Neuroradiol. 2010;31(10):1853–1860.
51 Harrigan M, Leonardo J, Gibbons K, Guterman L, Hopkins L. CT perfusion cerebral blood flow imaging in neurological critical care. Neurocrit Care. 2005;2(3):352–366.
52 Kannan S, Balakrishnan B, Muzik O, Romero R, Chugani D. Positron emission tomography imaging of neuroinflammation. J Child Neurol. 2009;24(9):1190–1199.
53 Vik A, Nag T, Fredriksli OA, Skandsen T, Moen KG, Schirmer-Mikalsen K, Manley GT. Relationship of “dose” of intracranial hypertension to outcome in severe traumatic brain injury. J Neurosurg. 2008;109(4):678–684.
54 Koskinen LO, Olivecrona M. Clinical experience with the intraparenchymal intracranial pressure monitoring Codman MicroSensor system. Neurosurgery. 2005;56(4):693–698.
55 Harrop JS, Sharan AD, Ratliff J, Prasad S, Jabbour P, et al. Impact of a standardized protocol and antibiotic-impregnated catheters on ventriculostomy infection rates in cerebrovascular patients. Neurosurgery. 2010;67(1):187–191.
56 Adelson PD, Bratton SL, Carney NA, Chesnut RM, du Coudray HE, et al. Guidelines for the acute medical management of severe traumatic brain injury in infants, children, and adolescents. Chapter 7, intracranial pressure monitoring technology. J Trauma. 54(6 Supp), 2003.
57 Bershad EM, Humphreis WE, III., Suarez JI. Intracranial hypertension. Semin Neurol. 2008;28(5):690–702.
58 Brain Trauma Foundation et al. Guidelines for the management of severe traumatic brain injury. VIII. Intracranial pressure thresholds. J Neurotrauma. 2007;24(Supp 1):S55–S58.
59 White H, Venkatesh B. Cerebral perfusion pressure in neurotrauma. Anesth Analg. 2008;107(3):979–988.
60 Balestreri M, Czosnyka M, Steiner LA, Schmidt E, Smielewski P, et al. Intracranial hypertension: What additional information can be derived from ICP waveform after head injury? Acta Neurochir. 2004;146(2):131–141.
61 Kasprowicz M, Asgari S, Bergsneider M, Czosnyka M, Hamilton R, Hu X. Pattern recognition of overnight intracranial pressure slow waves using morphological features of intracranial pressure pulse. J Neurosci Methods. 2010;190(2):310–318.
62 Hu X, Glenn T, Scalzo F, Bergsneider M, Sarkiss C, Martin N, Vespa P. Intracranial pressure pulse morphological features improved detection of decreased cerebral blood flow. Physiol Meas. 2010;31(5):679–695.
63 Howells T, Elf K, Jones PA, Ronne-Engstrom E, Piper I, et al. Pressure reactivity as a guide in the treatment of cerebral perfusion pressure in patients with brain trauma. J Neurosurg. 2005;102(2):311–317.
64 Johnston AJ, Steiner LA, Coles JP, et al. Effect of cerebral perfusion pressure augmentation on regional oxygenation and metabolism after head injury. Crit Care Med. 2005;33:189–195.
65 Brain Trauma Foundation Guidelines IX. Cerebral perfusion thresholds. J Neurotrauma. 2007;24(Supp 1):S59–S64.
66 Brain Trauma Foundation Guidelines, Ch 8. Cerebral perfusion pressure: guidelines for the management of severe traumatic brain injury. Paediat Crit Care Med. 2003;4(3):S31–S33.
67 Udomphorn Y, Armstead WM, Vavilala MS. Cerebral blood flow and autoregulation after pediatric traumatic brain injury. Pediatr Neurol. 2008;38(4):225–234.
68 Chieregato A, Calzolari F, Trasforini G, Targa L, Latronico N. Normal jugular bulb oxygen saturation. J Neurol, Neurosurg Psych. 2003;74(6):784–786.
69 Bratton SL, Chestnut RM, Ghajar J, McConnell Hammond FF, Harris OA, et al. Guidelines for the management of severe traumatic brain injury. X. Brain oxygen monitoring and thresholds. J Neurotrauma. 2007;24(S1):S65–S70.
70 Rabinstein AA. Elucidating the value of continuous brain oxygen monitoring. Neurocritical Care. 2010;12(1):144–145.
71 Leal-Noval SR, Cayuela A, Arellano-Orden V, Marín-Caballos A, Padilla V, et al. Invasive and noninvasive assessment of cerebral oxygenation in patients with severe traumatic brain injury. Intensive Care Med. 2010;36(8):1309–1317.
72 Maloney-Wilensky E, Gracias V, Itkin A, Hoffman K, Bloom S, et al. Brain tissue oxygen and outcome after severe traumatic brain injury: A systematic review. Crit Care Med. 2009;37(6):2057–2063.
73 Kawai N, Kawakita K, Yano T, Tamiya T, Abe Y, Kuroda Y. Use of intracerebral microdialysis in severe traumatic brain injury. Neurol Surg. 2010;38(9):795–809.
74 Chefer VI, Thompson AC, Zapata A, Shippenberg TS. Overview of brain microdialysis. Curr Prot in Neuroscience. 2009:7.1.1–7.1.28.
75 Uehara T, Sumiyoshi T, Itoh H, Kurata K. Lactate production and neurotransmitters; evidence from microdialysis studies. Pharm Bio Behav. 2008;90(2):273–281.
76 Bouzat P, Francony G, Fauvage B, Payen J. Transcranial doppler pulsatility index for initial management of brain-injured patients. Neurosurgery. 2010;67(6):E1863–E1864.
77 White DM, Van Cott AC. EEG artifacts in the intensive care unit setting. Am J Elect Tech. 2010;50(1):8–25.
78 Kurtz P, Hanafy KA, Claassen J. Continuous EEG monitoring: Is it ready for prime time? Curr Opin Crit Care. 2009;15(2):99–109.
79 Guérit J. Neurophysiological testing in neurocritical care. Curr Opin Crit Care. 2010;16(2):98–104.
80 Murkin JM, Arango M. Near-infrared spectroscopy as an index of brain and tissue oxygenation. Br J Anaesth. 2009;103(Suppl 1):i3–13.
81 Bhatia R, Hampton T, Malde S, et al. The application of near-infrared oximetry to cerebral monitoring during aneurysm embolization: a comparison with intraprocedural angiography. J Neurosurg Anesthesiol. 2007;19:97–104.
82 Purves D, Augustine G, Fitzpatrick D, Katz L, LaMantia A, McNamara J, et al. Neuroscience, 2nd edn. New York: Sinauer Associates; 2001.