Chapter 23 Muscle
Skeletal Muscle
Shape and Fibre Architecture
It is possible to classify muscles based on their general shape and the predominant orientation of their fibres relative to the direction of pull (Fig. 23.1). Muscles with fibres that are largely parallel to the line of pull vary in form from flat, short and quadrilateral (e.g. thyrohyoid) to long and strap-like (e.g. sternohyoid, sartorius). In such muscles, individual fibres may run for the entire length of the muscle or over shorter segments when there are transverse, tendinous intersections at intervals (e.g. rectus abdominis). In a fusiform muscle, the fibres may be close to parallel in the belly but converge to a tendon at one or both ends. Where fibres are oblique to the line of pull, muscles may be triangular (e.g. temporalis, adductor longus) or pennate (feather-like) in construction. The latter vary in complexity (see Fig. 23.1) from unipennate (e.g. flexor pollicis longus) and bipennate (e.g. rectus femoris, dorsal interossei) to multipennate (e.g. deltoid). In some muscles, the fibres pass obliquely between deep and superficial aponeuroses, in a type of unipennate form (e.g. soleus). In other sites, muscle fibres start from the walls of osteofascial compartments and converge obliquely on a central tendon in circumpennate fashion (e.g. tibialis anterior). Some muscles have a spiral or twisted arrangement (e.g. sternocostal fibres of pectoralis major and latissimus dorsi, which undergo a 180° twist between their median and lateral attachments). Others spiral around a bone (e.g. supinator, which winds obliquely around the proximal radial shaft) or contain two or more planes of fibres arranged in different directions, a type of spiral sometimes referred to as cruciate; sternocleidomastoid, masseter and adductor magnus are all partially spiral and cruciate. Many muscles display more than one of these major types of arrangement and show regional variations that correspond to contrasting and, in some cases, independent actions.
Microstructure
The cellular units of skeletal muscle are the muscle fibres (Fig. 23.2). These long, cylindrical structures tend to be consistent in size within a given muscle, but in different muscles they may range from 10 to 100 µm in diameter and from millimetres to many centimetres in length. Some typical skeletal muscle fibres are seen in longitudinal section in Figure 23.3. Their staining characteristics are dominated by the contractile apparatus, which constitutes much of the cytoplasm or sarcoplasm. The contractile proteins are organized into cylindrical myofibrils that are too tightly packed to be visible by routine light microscopy. Of greater significance are transverse striations, which are the result of alignment across the fibre of repeating elements, the sarcomeres, within neighbouring myofibrils. These cross-striations are usually evident in sections stained conventionally, but they may be demonstrated more effectively using special stains (Fig. 23.4).
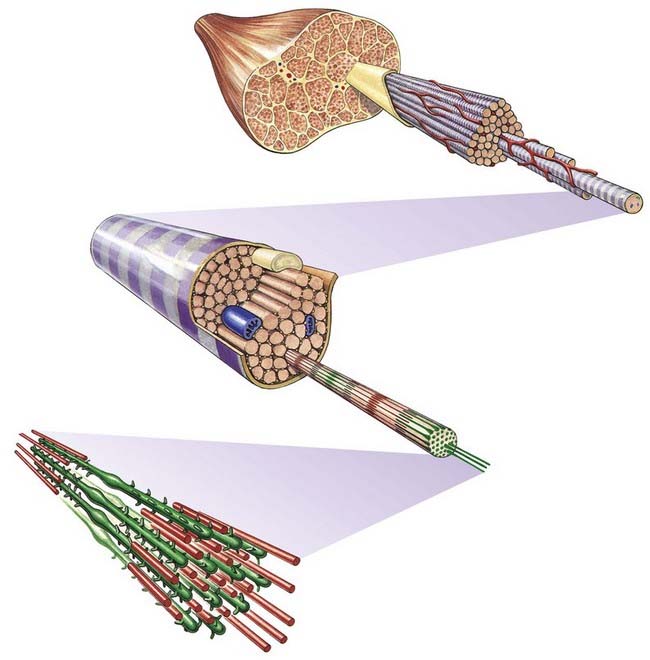
Fig. 23.2 Levels of organization within a skeletal muscle, from whole muscle to fasciculi, single fibres, myofibrils and myofilaments.
Under polarized light, the striations are even more striking and are seen as a pattern of alternating dark and light bands. The darker bands are birefringent, rotating the plane of polarized light strongly, and are known as anisotropic or A-bands; the lighter bands rotate the plane of polarized light to a negligible degree and are known as isotropic or I-bands. The structures responsible for this appearance are described more readily at the ultrastructural level.
The multiple nuclei are oval and are located at the periphery of the fibres, under the plasma membrane or sarcolemma. They are especially numerous in the region of the neuromuscular junction. The nuclei are moderately euchromatic and usually have one or more nucleoli. They occupy a thin, transparent rim of sarcoplasm between the myofibrils and the sarcolemma and are seen most clearly in transverse sections (Fig. 23.5). Other nuclei belonging to vascular endothelial cells, Schwann cells, fibroblasts, and so forth may be present in the spaces between the fibres, where blood vessels and nerve fibres travel through layers of fine connective tissue, the endomysium. Nuclei of satellite cells lie between the sarcolemma and the surrounding basal lamina.
In transverse section, the profiles of the fibres are usually polygonal (see Fig. 23.5). Some muscles, such as the extrinsic muscles of the larynx, tend to be less tightly packed. In such situations, as well as in conditions of generalized wasting or muscle damage, the fibres may adopt a more rounded profile; however, in some normal muscles, such as those that close the jaw, the fibres are closely packed but have rounded profiles. The sarcoplasm often has a stippled appearance because the transversely sectioned myofibrils are resolved as dots.
Skeletal muscle fibres are large (with a few exceptions, such as the laryngeal muscles), and unless electron micrographs are taken at very low magnification, they seldom show more than part of the interior of a fibre (Fig. 23.6A). Myofibrils are the dominant ultrastructural feature of such micrographs. They are cylindrical structures approximately 1 µm in diameter, which appear as ribbons in longitudinal section. Thin, very densely stained transverse lines, which correspond to discs in the parent cylindrical structure, appear at regular intervals along these ribbons. They are called Z-lines or, more properly, Z-discs (Zwischenscheiben, or ‘between discs’). They divide the myofibril into a linear series of identical contractile units called sarcomeres, each of which is approximately 2.2 µm long in resting muscle.
At higher power, sarcomeres are seen to consist of two types of filament—thick and thin—organized into regular arrays (Fig. 23.6B). The thick filaments, which are approximately 15 nm in diameter, are composed mainly of myosin. The thin filaments, which are 8 nm in diameter, are composed mainly of actin. The arrays of thick and thin filaments form a partially overlapping structure in which the electron density varies according to the amount of protein present. The A-band consists of the thick filaments, together with lengths of thin filaments that interdigitate with, and thus overlap, the thick filaments at either end (see Fig. 23.6B; Fig. 23.7). The central, paler region of the A-band, into which the thin filaments have not penetrated, is called the H zone (helle, or ‘light’). At their centres, the thick filaments are linked together transversely by material that constitutes the M line (Mittelscheibe, or ‘middle (of) disc’), which is visible in most muscles.
The I-band consists of the adjacent portions of two neighbouring sarcomeres in which the thin filaments are not overlapped by thick filaments. It is bisected by the Z-disc, into which the thin filaments of adjacent sarcomeres are anchored. In addition to the thick and thin filaments, there is a third type of filament composed of the elastic protein titin. The high degree of organization of the filament arrays is equally evident in electron micrographs of transverse sections (see Fig. 23.7; Fig. 23.8). The thick myosin filaments form a hexagonal lattice; in the regions where they overlap with the thin filaments, each myosin filament is surrounded by six actin filaments at the trigonal points of the lattice. In the I-band, the thin filament pattern changes from hexagonal to square as the filaments approach the Z-disc, where they are incorporated into a square lattice structure.
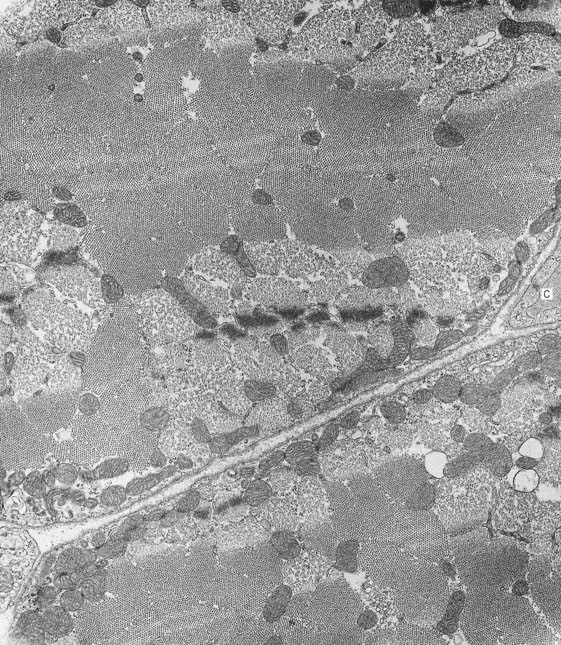
Fig. 23.8 Electron micrograph of skeletal muscle in transverse section, showing parts of two muscle fibres. Part of a capillary (C) is seen in transverse section in the endomysial space (right). The variation in the appearance of myofibrils in cross-section is explained in Figure 23-7.
(Photograph by Brenda Russell, Department of Physiology and Biophysics, University of Illinois at Chicago.)
Muscle Proteins
CASE 1 Duchenne’s Muscular Dystrophy
A 4-year-old boy presents with difficulty rising from the floor. His parents report that he has always walked on his toes and has had trouble climbing stairs and running, but now he must get on all fours, push himself into a squat and then push off his legs with his hands to stand (Gowers’ sign).
On examination, he has large, firm calves (Fig. 23.9). His gait is waddling, with a broad base and marked lumbar lordosis. He has proximal lower extremity weakness, significant neck flexor weakness and Gowers’ sign when rising from the floor. The remainder of the examination is normal.
Other Sarcoplasmic Structures
Although myofibrils are the dominant ultrastructural feature of skeletal muscle, the fibres contain other organelles essential for cellular function, such as ribosomes, Golgi apparatus and mitochondria. Most of them are located around the nuclei, between the myofibrils and the sarcolemma and, to a lesser extent, between the myofibrils. Mitochondria, lipid droplets and glycogen provide the metabolic support needed by active muscle. The mitochondria are elongated, and their cristae are closely packed. Their profiles are usually seen in longitudinal orientation between the myofibrils (see Fig. 23.6A). The number of mitochondria in an adult muscle fibre is not fixed, but it can increase or decrease quite readily in response to sustained changes in activity. Spherical lipid droplets, approximately 0.25 µm in diameter, are distributed uniformly throughout the sarcoplasm between myofibrils. They represent a rich source of energy that can be tapped only by oxidative metabolic pathways; they are therefore more common in fibres that have a high mitochondrial content and good capillary blood supply. Glycogen is distributed in small clusters of granules between myofibrils and among the thin filaments. With brief bursts of activity, it provides an important source of anaerobic energy that is not dependent on nutrient blood flow to the muscle fibre.
At the ends of the muscle fibre, where force is transmitted to adjacent connective tissue structures, the sarcolemma is folded into numerous finger-like projections that strengthen the junctional region by increasing the area of attachment. Tubular invaginations of the sarcolemma penetrate between the myofibrils in a transverse plane at the limit of each A-band (Fig. 23.10). The lumina of these transverse tubules (T-tubules) are thus in continuity with the extracellular space. T-tubules play an important role in excitation–contraction coupling.
The sarcoplasmic reticulum is a specialized form of smooth endoplasmic reticulum. It consists of a plexus of anastomosing membrane cisternae that fill much of the space between myofibrils and expand into larger sacs, the junctional sarcoplasmic reticulum or terminal cisternae, where they come into close contact with T-tubules. At this point, they form part of a structure called a triad, consisting of a central T-tubule flanked on either side by two terminal cisternae, the latter filled with dense, granular material (see Fig. 23.6B). The membranes of the sarcoplasmic reticulum contain calcium–ATPase pumps that transport calcium ions into the terminal cisternae, where the ions are bound to calsequestrin, a protein with a high affinity for calcium, in dense storage granules. In this way, calcium can be accumulated and retained in the terminal cisternae at a much higher concentration than anywhere else in the sarcoplasm. Ca2+ release channels (made of ryanodine receptor molecules) are concentrated mainly in the terminal cisternae. They form half of the junctional ‘feet’ or ‘pillars’ that bridge the sarcoplasmic reticulum and T-tubules at the triads, forming a critical communication point between them. The other half of the junctional feet is the T-tubule receptor, which constitutes the voltage sensor.
Connective Tissues
The endomysium is a delicate network of connective tissue that surrounds muscle fibres and forms their immediate external environment. It is the site of metabolic exchange between muscle and blood and contains capillaries and bundles of small nerve fibres. Ion fluxes associated with the electrical excitation of muscle fibres take place through its proteoglycan matrix. The endomysium is continuous with more substantial septa of connective tissue that constitute the perimysium. The latter ensheathes groups of muscle fibres to form parallel bundles or fasciculi, carries larger blood vessels and nerves and accommodates neuromuscular spindles. Perimysial septa are themselves the inward extensions of a collagenous sheath, the epimysium, which forms part of the fascia that invests whole muscle groups.
Attachments
Tendons
Tendons take the forms of cords or straps that are round or oval in cross-section and consist of dense, regular connective tissue (Fig. 23.11). They contain fascicles of type I collagen that are oriented mainly parallel to the long axis but are, to some extent, interwoven. The fasciculi may be conspicuous enough to give tendons a longitudinally striated appearance to the unaided eye. Tendons generally have smooth surfaces, although large tendons may be ridged longitudinally by coarse fasciculi (e.g. the osseous aspect of the angulated tendon of obturator internus). Loose connective tissue between fascicles provides a pathway for small vessels and nerves and condenses on the surface as a sheath or epitendineum, which may contain elastic and irregularly arranged collagen fibres. The loose attachments between this sheath and the surrounding tissue present little resistance to movement of the tendon, but where greater freedom of movement is required, a tendon is separated from adjacent structures by a synovial sheath.
Because tendons are composed of collagen and their vascular supply is sparse, they appear white. However, the blood supply to tendons is not unimportant; small arterioles from adjacent muscle tissue pass longitudinally between the fascicles, branching and anastomosing freely, and are accompanied by venae commitantes and lymphatic vessels. This longitudinal plexus is augmented by small vessels from adjacent loose connective tissue or synovial sheaths. Vessels rarely pass between bone and tendon at osseous attachments, and the junctional surfaces are usually devoid of foramina. A notable exception is the calcaneal (Achilles) tendon, which receives a blood supply across the osseotendinous junction. During postnatal development, tendons enlarge by interstitial growth, particularly at myotendinous junctions, where there are high concentrations of fibroblasts. Growth decreases along the tendon from the muscle to the osseous attachments. The thickness finally attained by a tendon depends on the size and strength of the associated muscle, but it also appears to be influenced by other factors, such as the degree of pennation of the muscle. The metabolic rate of tendons is very low but increases during infection or injury. Repair involves the initial proliferation of fibroblasts, followed by interstitial deposition of new fibres.
Innervation
A specialized synapse, the neuromuscular junction (Ch. 22), is formed where the terminal branch of an α motor axon contacts the muscle fibre. The axon terminal gives off several short, tortuous branches, each ending in an elliptical area, the motor end-plate. Within the underlying discoidal patch of sarcolemma—the sole plate or subneural apparatus—the sarcolemma is thrown into deep synaptic folds. This discrete type of neuromuscular junction is an example of an en plaque ending and is found on muscle fibres that are capable of propagating action potentials. A different type of ending is found on slow tonic muscle fibres that do not have this capability (e.g. the extrinsic ocular muscles), where these fibres form a minor component of the muscle. In this case, the propagation of excitation is taken over by the nerve terminals, which branch over an extended distance to form a number of small neuromuscular junctions (en grappe endings). Some muscle fibres of this type receive the terminal branches of more than one motor neurone. The terminals of the γ-efferents that innervate the intrafusal muscle fibres of the neuromuscular spindle also take a variety of forms.
The terminal branches of α motor axons are normally in a ‘one-to-one’ relationship with their muscle fibres: a muscle fibre receives only one branch, and any one branch innervates only one muscle fibre. When a motor neurone is excited, an action potential is propagated along the axon and all its branches to all the muscle fibres it supplies. The motor neurone and the muscle fibres it innervates can therefore be regarded as a functional unit, the motor unit, which accounts for the more or less simultaneous contraction of a number of fibres within the muscle.
Muscle Contraction: Basic Physiology
Electron microscopy shows that the length of the thick and thin filaments does not change during muscle contraction. The sarcomere shortens by means of the thick and thin filaments sliding past each other, which draws the Z-discs toward the middle of each sarcomere (see Fig. 23.7). As the overlap increases, the I-bands and H zones narrow to extinction, while the width of the A-bands remains constant. Filament sliding depends on the making and breaking of bonds (cross-bridge cycling) between myosin head regions and actin filaments. Myosin heads ‘walk’ along actin filaments (sliding the filaments past each other) using a series of short power strokes, each resulting in a relative movement of 5 to 10 nm. Actin filament binding sites for myosin are revealed only in the presence of calcium, which is released into the sarcoplasm from the sarcoplasmic reticulum, with the consequent repositioning of the troponin–tropomyosin complex on actin (the calcium-sensitive switch). Both myosin head binding and release are energy dependent (adenosine triphosphate (ATP) binding is required for detachment of bound myosin heads as part of the normal cycle). In the absence of ATP (as occurs postmortem), the bound state is maintained and is responsible for the muscle stiffness known as rigor mortis.
Slow Twitch versus Fast Twitch
Muscles that are predominantly oxidative in their metabolism contract and relax more slowly than muscles that rely on glycolytic metabolism. This difference in contractile speed is due in part to the activation mechanism (volume density of sarcotubular system and proteins of the calcium ‘switch’ mechanism) and in part to molecular differences between the myosin heavy chains of these types of muscle, which affect the ATPase activity of the myosin head; this in turn alters the kinetics of its interaction with actin and hence the rate of cross-bridge cycling. Differences between myosin isoforms may be detected histochemically; ATPase histochemistry continues to play a significant role in diagnostic typing (Table 23.2). Two main categories have been described: type I fibres, which are slow contracting, and type II fibres, which are fast contracting. Molecular analyses have revealed that type II fibres can be subdivided according to their content of myosin heavy-chain isoforms into types IIA, IIB and IIX (Schiaffino and Reggiani 1996). There is a correlation between categories and metabolism and therefore with fatigue resistance: type I fibres are generally oxidative (slow oxidative) and resistant to fatigue; type IIA are moderately oxidative, glycolytic (fast oxidative glycolytic) and fatigue resistant; and type IIB largely rely on glycolytic metabolism (fast glycolytic) and so are easily fatigued. Fibre type grouping (Fig. 23.12) occurs when there are repeated cycles of denervation and reinnervation and is seen in a variety of conditions, including disuse atrophy, ageing, demyelination neuropathies and some forms of muscular dystrophy.
Table 23-2 Physiological, structural and biochemical characteristics of the major histochemical fibre types
CASE 3 Inclusion Body Myositis
Discussion: Inclusion body myositis is the most common acquired inflammatory myopathy. The predominantly distal pattern of weakness helps distinguish this disease from other inflammatory myopathies that involve primarily proximal muscles. Muscle biopsy in such cases shows endomysial mononuclear cell inflammation; scattered muscle fibre degeneration and regeneration, with characteristic rimmed vacuoles (Fig. 23.13); clustering of small fibres; and both intranuclear and intracytoplasmic inclusions that stain for amyloid.
Bishopric N.H., Gahlmann R., Wade R., Kedes L. Gene expression during skeletal and cardiac muscle development. In: Fozzard H.A., Haber E., Jennings R.B., Katz A.M., Morgan H.E., editors. The Heart and Cardiovascular System. second ed. New York: Raven Press; 1991:1587-1598.
Brand-Saberi B., Christ B. Evolution and development of distinct cell lineages derived from somites. Current Topics in Developmental Biology. Vol. 48. 2000. Academic Press. New York.
Buckingham M., Bajard L., Chang T, et al. The formation of skeletal muscle: from somite to limb. J. Anat.. 2003;202:59-68.
Buller A.J., Eccles J.C., Eccles R.M. Interactions between motoneurones and muscles in respect of the characteristic speeds of their responses. J. Physiol.. 1960;150:417-439.
Edgerton V.R., Roy R.R., Allen D.L., Monti R.J. Adaptations in skeletal muscle disuse or decreased-use atrophy. Am. J. Phys. Med. Rehabil.. 2002;81(11 Suppl.):S127-S147.
Goldring, Partridge, Watt, 2002Goldring K. Partridge T. Watt D. Muscle stem cells. J. Pathol.. 197:2002;457-467.
Reviews the role of the satellite cell in growth and repair of muscle fibres.
King Engel W., Askanas V. Inclusion-body myositis: clinical, diagnostic and pathologic aspects. Neurology Suppl.. 2006;1(66):S20-S29.
Ko C.P., Thompson W.J. The Neuromuscular Junction, (Guest Eds.). J. Neurocytol. 32:2003;421-1037.
Lieber R.L., Friden J. Functional and clinical significance of skeletal muscle architecture. Muscle Nerve.. 2000;23:1647-1666.
Maltin C.A., Delday M.I., Sinclair K.D., Steven J., Sneddon A.A. Impact of manipulations of myogenesis in utero on the performance of adult skeletal muscle. Reproduction. 2001;122:359-374.
Mathes S.J., Nahai F. Classification of the vascular anatomy of muscles: experimental and clinical correlation. Plast. Reconstr. Surg.. 1981;67:177-187.
Mizuno H., Zuk P.A., Zhu M., Lorenz H.P., Benhaim P., Hedrick M.H. Myogenic differentiation by human processed lipoaspirate cells. Plast. Reconstr. Surg.. 2002;109:199-209.
Muntoni F., Brown S., Sewry C., Patel K. Muscle development genes: their relevance in neuromuscular disorders. Neuromuscul. Disord.. 2002;12:438-446.
Salmons S. An implantable muscle stimulator. J. Physiol.. 1967;188:13P-14P.
Schiaffino S., Reggiani C. Molecular diversity of myofibrillar proteins: gene regulation and functional significance. Physiol. Rev.. 1996;76:371-423.