Chapter 8 Movements of the lumbar spine
Axial compression
Axial compression is the movement that occurs during weight-bearing in the upright posture, or as a result of contraction of the longitudinal back muscles (see Ch. 9). With respect to the interbody joints, the weight-bearing mechanisms of the intervertebral discs have already been described in Chapter 2, where it was explained how the nucleus pulposus and anulus fibrosus cooperate to transmit weight from one vertebra to the next. It is now appropriate to add further details.
During axial compression, both the anulus fibrosus and nucleus pulposus bear the load and transmit it to the vertebral endplates (see Ch. 2). In a normal disc, the outermost fibres of the anulus do not participate in bearing the load. Otherwise, the compression load is borne uniformly across the inner, anterior anulus and nucleus, but with a peak stress over the inner, posterior anulus (Fig. 8.1).2–4 In older discs this posterior peak is larger.3,4
Compression squeezes water out of the disc.5–7 Under a 100 kPa load, the nucleus loses some 8% of its water and the anulus loses 11%.8–10 The loss of water results in a relative increase in the concentration of electrolytes remaining in the disc, and this increased concentration serves to re-imbibe water into the disc once compression is released.9
Under compression, the vertebral bodies around a disc approximate and the disc bulges radially.6,8,11 The vertebral bodies approximate because the vertebral endplates bow away from the disc.11–13 Indeed, the deflection of each endplate is almost equal to half the displacement of the vertebrae.12 This amounts to a strain of approximately 3% in the endplate.12 The disc bulges because, as the anulus loses height peripherally, the redundant length must somehow be accommodated, i.e. the lamellae of the anulus must buckle. Nuclear pressure normally prevents buckling inwards, leaving outward radial bulging as the only means of accommodating loss of disc height. The bulging is greater anteriorly than at the posterolateral corner of the disc, and induces a strain in the anulus fibrosus of about 2% per mm loss of disc height.14 Removing part of the nucleus (as occurs in discectomy) increases both the loss of disc height and the radial bulge.15
The load on the endplate during compression is evenly distributed over its surface, there being no greater load over the nucleus pulposus than over the anulus fibrosus.16 The endplate bows, however, because its periphery its strongly supported by the underlying cortical bone of the vertebra, whereas its central portion is supported by the slightly weaker trabecular bone of the vertebral body. This trabecular support is critical to the integrity of the endplate.
When excessive loads are applied to normal intervertebral discs, the trabeculae under the endplates fracture and the endplates themselves fracture, typically in their central region, i.e. over the nucleus pulposus, rather than over the anulus.5,17–20 With the application of very great loads the entire endplate may fracture.19–21
In this context, it is noteworthy that the endplates are the weakest components of the intervertebral disc in the face of axial compression. Provided the anulus is healthy and intact, increasing the load causes one or other of the endplates to fail, by fracturing, sooner than the anulus fibrosus fails, by rupturing.5,19,20 This phenomenon has particular ramifications in the pathology of compression injuries of the lumbar spine and disc degradation (see Ch. 15), and has its basis in the relative strengths of the anulus fibrosus and the bone of the vertebral body. Calculations have shown that the anulus fibrosus can withstand a pressure of 3.2 × 107 Nm−2 but cancellous bone yields at 3.4 × 106 Nm−2.8 Consequently, endplates would be expected to fail sooner than the anulus fibrosus when the disc is subjected to axial compression.
With respect to the vertebral bodies, in adults under the age of 40, between 25% and 55% of the weight applied to a vertebral body is borne by the trabecular bone;11,22,23 the rest is borne by the cortical shell. In older individuals this proportion changes, for reasons explained in Chapter 13. Overall, the strength of a vertebral body is quite great but varies considerably between individuals. The ultimate compressive strength of a vertebral body ranges between 3 and 12 kN.24,25 This strength is directly related to bone density24,26,27 and can be predicted to within 1 kN on the basis of bone density and endplate area determined by CT scanning.28 It also seems to be inversely related to physical activity, in that active individuals have stronger vertebrae.29
Another factor that increases the load-bearing capacity of the vertebral body is the blood within its marrow spaces and intra-osseous veins (see Ch. 11). Compression of the vertebral body and bulging of the endplates causes blood to be extruded from the vertebra.6 Because this process requires energy, it buffers the vertebral body, to some extent, from the compressive loads applied to it.20
During compression, intervertebral discs undergo an initial period of rapid creep, deforming about 1.5 mm in the first 2–10 min depending on the size of the applied load.30–32 Subsequently, a much slower but definite creep continues at about 1 mm per hour.32 Depending on age, a plateau is attained by about 90 min, beyond which no further creep occurs.31
Creep underlies the variation in height changes undergone by individuals during activities of daily living. Over a 16-hour day, the pressure sustained by intervertebral discs during walking and sitting causes loss of fluid from the discs, which results in a 10% loss in disc height10 and a 16% loss of disc volume.33 Given that intervertebral discs account for just under a quarter of the height of the vertebral column, the 10% fluid loss results in individuals being 1–2% shorter at the end of a day.34–36 This height is restored during sleep or reclined rest, when the vertebral column is not axially compressed and the discs are rehydrated by the osmotic pressure of the disc proteoglycans.10 Moreover, it has been demonstrated that rest in the supine position with the lower limbs flexed and raised brings about a more rapid return to full disc height than does rest in the extended supine position.36
The pressure within intervertebral discs can be measured using special needles,37–39 and disc pressure measurement, or discometry, provides an index of the stresses applied to a disc in various postures and movements. Several studies have addressed this issue although for technical reasons virtually all have studied only the L3–4 disc.
In the upright standing posture, the load on the disc is about 70 kPa.38 Holding a weight of 5 kg in this posture raises the disc pressure to about 700 kPa.38,40 The changes in disc pressure during other movements and manoeuvres are described in Chapter 9.
Although the interbody joints are designed as the principal weight-bearing components of the lumbar spine (see Ch. 2), there has been much interest in the role that the zygapophysial joints play in weight-bearing. The earliest studies in this regard provided indirect estimates of the load borne by the zygapophysial joints based on measurements of intradiscal pressure, and it was reported that the zygapophysial joints carried approximately 20% of the vertical load applied to an intervertebral joint.37 This conclusion, however, was later retracted.41
Subsequent studies have variously reported that the zygapophysial joints can bear 28%42 or 40%43 of a vertically applied load. To the contrary, others have reported that ‘compression did not load the facet joints … very much’,44 and that ‘provided the lumbar spine is slightly flattened … all the intervertebral compressive force is resisted by the disc’.45
Although the articular surfaces of the lumbar zygapophysial joints are curved in the transverse plane (see Ch. 3), in the sagittal and coronal planes they run straight up and down (although see Ch. 11). Thus, zygapophysial joints, in a neutral position, cannot sustain vertically applied loads. Their articular surfaces run parallel to one another and parallel to the direction of the applied load. If an intervertebral joint is axially compressed, the articular surfaces of the zygapophysial joints will simply slide past one another. For the zygapophysial joints to participate in weight-bearing in erect standing, some aberration in their orientation must occur, and either of two mechanisms may operate singly or in combination to recruit the zygapophysial joints into weight-bearing.
If a vertebra is caused to rock backwards on its intervertebral disc without also being allowed to slide backwards, the tips of its inferior articular processes will be driven into the superior articular facets of the vertebra below (Fig. 8.2). Axial compression of the intervertebral joint will then result in some of the load being transmitted through the region of impaction of the zygapophysial joints. By rocking a pair of lumbar vertebrae, one can readily determine by inspection that the site of impaction in the zygapophysial joints falls on the inferior medial portion of the facets. Formal experiments have shown this to be the site where maximal pressure is detected in the zygapophysial joints of vertebrae loaded in extension.46
Another mechanism does not involve the zygapophysial joint surfaces but rather the tips of the inferior articular processes. With severe or sustained axial compression, intervertebral discs may be narrowed to the extent that the inferior articular processes of the upper vertebra are lowered until their tips impact the laminae of the vertebra below (Fig. 8.3).47 Alternatively, this same impact may occur if an intervertebral joint is axially compressed while also tilted backwards, as is the case in a lordotic lumbar spine bearing weight.46–49 Axial loads can then be transmitted through the inferior articular processes to the laminae.
It has been shown that under the conditions of erect sitting, the zygapophysial joints are not impacted and bear none of the vertical load on the intervertebral joint. However, in prolonged standing with a lordotic spine, the impacted joints at each segmental level bear an average of some 16% of the axial load.45,48 In this regard, the lower joints (L3–4, L4–5, L5–S1) bear a relatively greater proportion (19%), while the upper joints (L1–2, L2–3) bear less (11%).48 Other studies have shown that the actual load borne by impaction of inferior articular processes varies from 3–18% of the applied load, and critically depends on the tilt of the intervertebral joint.49 It has also been estimated that pathological disc space narrowing can result in some 70% of the axial load being borne by the inferior articular processes and laminae.45
It is thus evident that weight-bearing occurs through the zygapophysial joints only if the inferior articular processes impact either the superior articular facets or the laminae of the vertebra below. Variations in the degree of such impactions account for the variations in the estimates of the axial load carried by the zygapophysial joints,49 and explain why the highest estimates of the load borne are reported in studies in which the intervertebral joints have been loaded in the extended position.42,43,50–52
In this way, the lordosis of the lumbar spine provides an axial load-bearing mechanism additional to those available in the intervertebral discs and the zygapophysial joints. Moreover, as described in Chapter 5, the tensile mechanism of the anterior ligaments imparts a resilience to the lumbar spine. The energy delivered to the ligaments is stored in them as tension and can be used to restore the curvature of the lumbar spine to its original form, once the axial load is removed.
Fatigue failure
Loads of between 37% and 80% of ultimate compression strength, applied at 0.5 Hz, can cause subchondral fractures after as few as 2000 or even 1000 cycles.53 Loads between 50% and 80% of ultimate stress can cause subchondral and other vertebral fractures after fewer than 100 cycles.26
The probability of failure is a function of the load applied and the number of repetitions. Loads below 30% ultimate stress are unlikely to result in failure, even after 5000 repetitions; increasing the load increases the probability of failure after fewer repetitions.24 At loads of 50–60% of ultimate stress, the probability of failure after 100 cycles is 39%; at loads of 60–70% ultimate strength, this probability rises to 63%.24 The lesions induced range from subchondral trabecular fractures to impressions of an endplate, frank fractures of an endplate and fractures of the cortical bone of the vertebral body.24 Repetitions of 100 and up to 1000 are within the calculated range for a variety of occupational activities, as are loads of 60% ultimate stress of an average vertebral body.24
Endplate fractures result in a loss of disc height17 and changes in the distribution of stress across the nucleus and anulus. The stress over the nucleus and anterior anulus decreases, while that over the posterior anulus rises.1,11 This increase in stress causes the lamellae of the anulus to collapse inwards towards the nucleus, thereby disrupting the internal architecture of the disc.11 Thus, even a small lesion can substantially compromise the normal biomechanics of a disc. The clinical significance of these phenomena is explored further in Chapter 15.
Axial distraction
Compared to axial compression and other movements of the lumbar spine, axial distraction has been studied far less. One study provided data on the stress–strain and stiffness characteristics of lumbar intervertebral discs as a whole, and revealed that the discs are not as stiff in distraction as in compression.51 This is understandable, for the discs are designed principally for weight-bearing and would be expected to resist compression more than tension. In a biological sense, this correlates with the fact that humans spend far more time bearing compressive loads – in walking, standing and sitting – than sustaining tensile loads, as might occur in brachiating (tree-climbing) animals.
Other studies have focused on individual elements of the intervertebral joints to determine their tensile properties. When stretched along their length, isolated fibres of the anulus fibrosus exhibit a typical ‘toe’ region between 0% and 3% strain, a failure stress between 4 and 10 MPa, and a strain at failure between 9% and 15%; their stiffness against stretch ranges from 59 to 140 MPa.54 If the anulus is tested while still attached to bone and distracted along the longitudinal axis of the vertebral column, as opposed to along the length of the fibres, the failure stress remains between 4 and 10 MPa but the stiffness drops to between 10 and 80 MPa.55 These tensile properties seem to vary with location but the results between studies are conflicting. Isolated fibres seem to be stiffer and stronger in the anterior region than in the posterolateral region of the disc, and stiffer in the outer regions of the anulus than in the inner regions.1 On the other hand, in intact specimens, the outer anterior anulus is weaker and less stiff than the outer posterior anulus.55
The capsules of the zygapophysial joints are remarkably strong when subjected to longitudinal tension. A single capsule can sustain 600 N before failing.56 Figuratively, this means that a pair of capsules at a single level can bear twice the body weight if subjected to axial distraction.
There has been one study57 that has described the behaviour of the whole (cadaveric) lumbar spine during sustained axial distraction, to mimic the clinical procedure of traction. Application of a 9 kg weight to stretch the lumbar spine results in an initial mean lengthening of 7.5 mm. Lengthening is greater (9 mm) in lumbar spines of young subjects, and less in the middle-aged (5.5 mm) and the elderly (7.5 mm). Sustained traction over 30 min results in a creep of a further 1.5 mm. Removal of the load reveals an immediate ‘set’ of about 2.5 mm, which reduces to only 0.5 mm by 30 min after removal of the load. Younger spines demonstrate a more rapid creep and do not show a residual ‘set’. The amount of distraction is greater in spines with healthy discs (11–12 mm) and substantially less (3–5 mm) in spines with degenerated discs.
Some 40% of the lengthening of the lumbar spine during traction occurs as a result of flattening of the lumbar lordosis, with 60% due to actual separation of the vertebral bodies. The major implication of this observation is that the extent of distraction achieved by traction (using a 9 kg load) is not great. It amounts to 60% of 7.5 mm of actual vertebral separation, which is equivalent to about 0.9 mm per intervertebral joint. This revelation seriously compromises those theories that maintain that lumbar traction exerts a beneficial effect by ‘sucking back’ disc herniations, and it is suggested that other mechanisms of the putative therapeutic effect of traction be considered.57
Flexion
During flexion, the entire lumbar spine leans forwards (Fig. 8.4). This is achieved basically by the ‘unfolding’ or straightening of the lumbar lordosis. At the full range of forward flexion, the lumbar spine assumes a straight alignment or is curved slightly forwards, tending to reverse the curvature of the original lordosis (see Fig. 8.3). The reversal occurs principally at upper lumbar levels. Reversal may occur at the L4–5 level but does not occur at the L5–S1 level.58,59 Forward flexion is therefore achieved for the most part by each of the lumbar vertebrae rotating from their backward tilted position in the upright lordosis to a neutral position, in which the upper and lower surfaces of adjacent vertebral bodies are parallel to one another. This relieves the posterior compression of the intervertebral discs and zygapophysial joints, present in the upright lordotic lumbar spine. Some additional range of movement is achieved by the upper lumbar vertebrae rotating further forwards and compressing their intervertebral discs anteriorly.
It may appear that during flexion of the lumbar spine, the movement undergone by each vertebral body is simply anterior sagittal rotation. However, there is a concomitant component of forward translation as well.59,60 If a vertebra rocks forwards over its intervertebral disc, its inferior articular processes are raised upwards and slightly backwards (Fig. 8.5A). This opens a small gap between each inferior articular facet and the superior articular facet in the zygapophysial joint. As the lumbar spine leans forwards, gravity or muscular action causes the vertebrae to slide forwards, and this motion closes the gap between the facets in the zygapophysial joints (Fig. 8.5B). Further forward translation will be arrested once impaction of the zygapophysial joints is re-established, but nonetheless a small forward translation will have occurred. At each intervertebral joint, therefore, flexion involves a combination of anterior sagittal rotation and a small amplitude anterior translation.
Anterior sagittal translation is resisted by the direct impaction of the inferior articular facets of a vertebra against the superior articular facets of the vertebra below, and this process has been fully described in Chapter 3. This mechanism becomes increasingly important the further the lumbar spine leans forward, for with a greater forward inclination of the lumbar spine, the upper surfaces of the lumbar vertebral bodies are inclined downwards (Fig. 8.6), and there will be a tendency for the vertebrae above to slide down this slope.
The cardinal ramification of the anatomy of the zygapophysial joints with respect to forward shear is that in joints with flat articular surfaces, the load will be borne evenly across the entire articular surface (see Ch. 3), but in joints with curved articular surfaces the load is concentrated on the anteromedial portions of the superior and inferior articular facets (see Ch. 3). Formal experiments have shown that during flexion, the highest pressures are recorded at the medial end of the lumbar zygapophysial joints,46 and this has further bearing on the age changes seen in these joints (see Ch. 13).
The anterior sagittal rotation component of flexion is resisted by the zygapophysial joints in a different way. The mechanism involves tension in the joint capsule. Flexion involves an upward sliding movement of each inferior articular process, in relation to the superior articular process in each zygapophysial joint, and the amplitude of this movement is about 5–7 mm.61 This movement will tense the joint capsule, and it is in this regard that the tensile strength of the capsule is recruited. Acting as a ligament, each capsule can resist as much as 600 N.14,56 Indeed, the tension developed in the capsules during flexion is enough to bend the inferior articular processes downwards and forwards by some 5°.62
The other elements that resist the anterior sagittal rotation of flexion are the ligaments of the intervertebral joints. Anterior sagittal rotation results in the separation of the spinous processes and laminae. Consequently, the supraspinous and interspinous ligaments and the ligamenta flava will be tensed, and various types of experiments have been performed to determine the relative contributions of these structures to the resistance of flexion. The experiments have involved either studying the range of motion in cadavers in which various ligaments have been sequentially severed,60 or determining mathematically the stresses applied to different ligaments on the basis of the separation of their attachments during different phases of flexion.3
In young adult specimens, sectioning the supraspinous and interspinous ligaments and ligamenta flava results in an increase of about 5° in the range of flexion.60 (Lesser increases occur in older specimens but this difference is discussed in Ch. 13.) Sectioning the zygapophysial joint capsules results in a further 4° of flexion. Transecting the pedicles, to remove the bony locking mechanism of the zygapophysial joints, results in a further 15° increase in range.
To determine the simultaneous contribution by various structures to the resistance of flexion, mathematical analyses have been performed.3 The results indicate that in a typical lumbar intervertebral joint, the intervertebral disc contributes about 29% of the resistance, the supraspinous and interspinous ligaments about 19%, the ligamentum flavum about 13%, and the capsules of the zygapophysial joints about 39%. It is emphasised that these figures relate only to the resistance of anterior sagittal rotation, which is the movement that tenses these ligaments. They do not relate to the role played by the bony locking mechanism in preventing anterior translation during flexion.
Within the disc, the posterior anulus is tensed during flexion and the anterior anulus is relaxed. The posterior anulus exhibits a strain of 0.6% per degree of rotation, and the anterior anulus exhibits a reciprocal strain of –0.6% per degree.14 With respect to anterior translation, the anulus exhibits a strain of about 1% per mm of horizontal displacement.14 An isolated disc can withstand a flexion moment of about 33 Nm, and can sustain flexion angles of about 18°,63 but in an intact specimen it is protected by the posterior ligaments. In an intact intervertebral joint, the posterior ligaments protect the disc and resist 80% of the flexion moment and restrict the segment to 80% of the range of flexion that will damage the disc.63
Failure
If a lumbar spine is tested progressively to failure, it emerges that the first signs of injury (to the posterior ligaments) appear when the bending moment is about 60 Nm.2 Gross damage is evident by 120 Nm and complete failure occurs at 140–185 Nm.64,65 These data underscore the fact that ligaments alone are not enough to support the flexed lumbar spine and that they need support from the back muscles during heavy lifts that may involve moments in excess of 200 Nm (see Ch. 9). The disc fails by horizontal tears across the middle of the posterior anulus or by avulsion of the anulus from the ring apophysis.63
Speed of movement and sustained postures affect the resistance of the ligaments of the spine to flexion. Reducing the duration of movement from 10 s to 1 s increases resistance by 12%; holding a flexed posture for 5 min reduces resistance by 42%; holding for an hour reduces resistance by 67%.2 These figures indicate that various work postures involving stooping can put the spine at risk by weakening its resistance to movement. Ostensibly, creep is the basis for this change in resistance.
Repetitive loading of the spine in flexion produces a variety of changes and lesions. Repeated pure bending has little effect on the intervertebral joints.66 At most, it produces a 10% increase in the range of extension but no significant changes to other movements.66 Repeated bending under compression, however, produces a variety of lesions in many specimens. Loading a lumbar joint in 9–12° of flexion, under 1500–6000 N, at 40 times per minute for up to 4 h causes endplate fractures in about one in four specimens, and a variety of internal disruptions of the anulus fibrosus, ranging from buckling of lamellae to overt radial fissures.67 These lesions are similar to those observed under pure compression loading and should be ascribed not to bending but to the compression component of cyclic bending under compression.
The zygapophysial joints offer a resistance of up to 2000 N against the forward translation that occurs during flexion.2 This resistance passes from the inferior articular processes, through the laminae and pedicles, into the vertebral body. As a result, a bending force is exerted on the pars interarticularis. Repetitive loading of the inferior articular facets results in failure of the pars interarticularis or the pedicles. Subject to a force of 380–760 N, 100 times per minute, many specimens can sustain several hundred thousand repetitions but others fail after as few as 1500, 300 and 139 repetitions.68 These figures warn that, in addition to injuries to the disc, repeated flexion can induce fractures of the pars interarticularis.
Extension
Bony impaction usually occurs between the spinous processes.69 As a vertebra extends, its spinous process approaches the next lower spinous process. The first limit to extension occurs as the interspinous ligament buckles and becomes trapped between the spinous processes. Further extension is met with further compression of this ligament until the spinous processes virtually come into contact (Fig. 8.7A).69
In individuals with wide interspinous spaces, extension may be limited before spinous processes come into contact.69 Impaction occurs between the tip of one or other of the inferior articular processes of the moving vertebra and the subjacent lamina (Fig. 8.7B). This type of impaction is accentuated when the joint is subjected to the action of the back muscles,47 for in addition to extending the lumbar spine, the back muscles also exert a substantial compression load on it (see Ch. 9). Consequently, during active extension, the inferior articular processes are drawn not only into posterior sagittal rotation but also downwards as the entire intervertebral joint is compressed. Under these circumstances, the zygapophysial joints become weight-bearing, as explained above (see ‘Axial compression’).
The posterior elements, however, are not critical for limiting extension. Resection of the zygapophysial joints has little impact on the capacity of a lumbar segment to bear an extension load.70 The extension load, under these conditions, is adequately borne by the anterior anulus.70
Axial rotation
During axial rotation of an intervertebral joint, all the fibres of the anulus fibrosus that are inclined toward the direction of rotation will be strained. The other half will be relaxed (see Ch. 2). Based on the observation that elongation of collagen beyond about 4% of resting length leads to injury of the fibre (see Ch. 7), it can be calculated that the maximum range of rotation of an intervertebral disc without injury is about 3°.8 Beyond this range the collagen fibres will begin to undergo micro-injury. Moreover, observational studies have determined that the anulus fibrosus exhibits a strain of 1% per degree of axial rotation,14 which also sets a limit of 3° before excessive strain is incurred.
Experiments on lumbar intervertebral discs have shown that they resist torsion more strongly than bending movements, and the stress–strain curves for torsion rise very steeply in the range 0–3° of rotation.51 Very large forces have to be applied to strain the disc beyond 3°, and isolated discs (the posterior elements having being removed) fail macroscopically at about 12° of rotation.71 This suggests that 12° is the ultimate range for rotation before disc failure occurs but this relates to total macroscopic failure. Analysis of the stress–strain curves for intervertebral discs under torsion (Fig. 8.8) reveals an inflection point just before 3° of rotation, which indicates the onset of microscopic failure in the anulus fibrosus.71 The range between 3° and 12° represents continued microfailure until overt failure occurs.
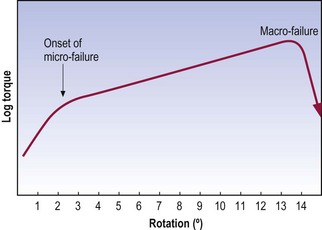
Figure 8.8 Stress–strain curve for torsion of the intervertebral disc.
(Based on Farfan et al. 1970.71)
In an intact intervertebral joint, the zygapophysial joints, and to a certain extent the posterior ligaments, protect the intervertebral disc from excessive torsion. Because the axis of rotation of a lumbar vertebra passes through the posterior part of the vertebral body,72 all the posterior elements of the moving vertebra will swing around this axis during axial rotation. As the spinous process moves, the attachments of the supraspinous and interspinous ligaments will be separated, and these ligaments will be placed under slight tension. Furthermore, one of the inferior articular facets of the upper vertebra will be impacted against its apposing superior articular facet (Fig. 8.9). In the case of left axial rotation, it will be the right inferior articular facet that impacts (and vice versa). Once this impaction occurs, normal axial rotation is arrested.
Given that the distance between a zygapophysial joint and the axis of rotation is about 30 mm, it can be calculated that about 0.5 mm of compression must occur for every 1° of axial rotation. Furthermore, given that the articular cartilages of a lumbar zygapophysial joint are about 2 mm thick (see Ch. 3), and that articular cartilage is about 75% water,73 it can be calculated that to accommodate 3° of rotation, the cartilages must be compressed to about 62% of their resting thickness and must lose more than half of their water. The zygapophysial joints therefore provide a substantial buffer during the first 3° of rotation, and the zygapophysial joint must be severely compressed before rotation exceeds the critical range of 3°, beyond which the anulus fibrosus risks torsional injury. Nevertheless, if sufficiently strong forces are applied, rotation can proceed beyond 3°, but then an ‘impure’ form of rotation occurs as the result of distortion of other elements in the intervertebral joint.
To rotate beyond 3°, the upper vertebra must pivot on the impacted joint, and this joint becomes the site of a new axis of rotation. Both the vertebral body and the opposite inferior articular process will then swing around this new axis. The vertebral body swings laterally and backwards, and the opposite inferior articular process swings backwards and medially (see Fig. 8.9C). The sideways movement of the vertebral body will exert a lateral shear on the underlying disc71,72 which will be additional to any torsional stress already applied to the disc by the earlier rotation. The backward movement of the opposite inferior articular process will strain the capsule of its zygapophysial joint.
During this complex combination of forces and movements, the impacted zygapophysial joint is being strained by compression, the intervertebral disc is strained by torsion and lateral shear, and the capsule of the opposite zygapophysial joint is being stretched. Failure of any one of these elements can occur if the rotatory force is sufficiently strong, and this underlies the mechanism of torsional injury to the lumbar spine (see Ch. 15).
The relative contributions of various structures to the resistance of axial rotation have been determined experimentally, and it is evident that the roles played by the supraspinous and interspinous ligaments, and by the capsule of the tensed (the opposite) zygapophysial joint are not great.74 The load is borne principally by the impacted zygapophysial joint and the intervertebral disc. Quantitative analysis71 reveals that the disc contributes 35% of the resistance to torsion, the remaining 65% being exerted by the posterior elements: the tensed zygapophysial joint; the supraspinous and interspinous ligaments; and principally the impacted zygapophysial joint. Experimental studies, however, have established that the zygapophysial joints contribute only between 42% and 54% of the torsional stiffness of a segment, the rest stemming from the disc.75
Fatigue failure
Specimens vary in their susceptibility to repetitive axial rotation. If the segment does not rotate beyond 1.5°, it can sustain 10 000 repetitions without visible damage. Segments which exhibit a larger initial range of motion, however, exhibit failure after 2000 or 3000 repetitions but in some cases after as few as 200–500, or even 50, repetitions.76 Failure occurs in the form of fractures of the facets, laminae or vertebral bodies, and tears in the anulus fibrosus and zygapophysial joint capsules.
Rotation in flexion
Using an external measuring device, Hindle and Pearcy77 observed in 12 subjects that the range of axial rotation of the lumbar spine increased when these subjects sat in a flexed position. This, they argued, occurred because, upon flexion, the inferior articular facets are lifted out of the sockets formed by the apposed superior articular facets, and if the inferior facets are tapered towards one another, they gain an extra range of motion in the transverse direction. Subsequently, they demonstrated this phenomenon in cadavers.78
Gunzburg et al. (1991)79 reported contrary data. They could not find increased rotation upon flexion either in cadavers or in living subjects in the standing position.
It has been argued that these differences can be explained by differences in compression loads.80 If a cadaveric specimen is compressed when flexed, the zygapophysial joints will remain deeper in their sockets than when allowed simply to flex. In living subjects, stooping while standing imposes large external loads that must be resisted by the back muscles, whose contraction will compress the moving segments (see Ch. 9). Consequently, increased axial rotation may be prevented by axial compression. However, this compression is not as great during flexion in the sitting position, under which conditions the increased axial rotation becomes apparent.
The argument concludes that increased axial rotation during flexion will not be apparent if the back muscles are strongly contracted although it may be apparent during sitting or if sudden external loads are applied which exceed the force of the back muscles. Under these circumstances, the increased axial rotation renders the disc liable to injury. As long as the zygapophysial joints limit rotation to less than 3°, the anulus is protected from injury. However, if axial rotation is greater than this, the anulus must undergo a greater strain and, moreover, one that is superimposed on the strain already induced by flexion.80
Range of movement
The availability and reliability of modern spondylo-meters, and the techniques for measuring the range of lumbar spinal motion are conveniently summarised in the AMA’s Guides to the Evaluation of Permanent Impairment, which also provides modern normative data.81 These, however, pertain to clinical measurements of spinal motion. They do not indicate exactly what happens in the lumbar spine and at each segment. That can be determined only by radiography.
Radiographic studies provide the most accurate measurements of living subjects but, although there have been many radiographic studies of segmental ranges of motion, these have now been superseded by the more accurate technique of biplanar radiography. Conventional radiography has the disadvantage that it cannot quantify movements that are not in the plane being studied. Thus, while lateral radiographs can be used to detect movement in the sagittal plane, they do not demonstrate the extent of any simultaneous movements in the horizontal and coronal planes. Such simultaneous movements can affect the radiographic image in the sagittal plane and lead to errors in the measurement of sagittal plane movements.58,59,82
The technique of biplanar radiography overcomes this problem by taking radiographs simultaneously through two X-ray tubes arranged at right angles to one another. Analysis of the two simultaneous radiographs allows movements in all three planes to be detected and quantified, allowing a more accurate appraisal of the movements that occur in any one plane.58,59,82
There have been two principal results stemming from the use of biplanar radiography. These are the accurate quantification of segmental motion in living subjects, and the demonstration and quantification of coupled movements.58,59,83,84 The segmental ranges of motion in the sagittal plane (flexion and extension), horizontal plane (axial rotation) and coronal plane (lateral bending) are shown in Table 8.1. It is notable that, for the same age group and sex (25- to 36-year-old males), all lumbar joints have the same total range of motion in the sagittal plane, although the middle intervertebral joints have a relatively greater range of flexion, while the highest and lowest joints have a relatively greater range of extension.
Table 8.1 Ranges of segmental motion in males aged 25–36 years. (Based on Pearcy et al. 198459 and Pearcy and Tibrewal 1984.84)
As determined by biplanar radiography, the mean values of axial rotation are approximately equal at all levels (see Table 8.1), and even the greatest values fall within the limit of 3°, which, from biomechanical evidence, is the range at which microtrauma to the intervertebral disc would occur. Conspicuously, the values obtained radiographically are noticeably smaller than those obtained both in cadavers and in living subjects using a spondylometer. The reasons for this discrepancy have not been investigated but may be due to the inability of clinical measurements to discriminate primary and coupled movements.
Coupled movements are movements that occur in an unintended or unexpected direction during the execution of a desired motion, and biplanar radiography reveals the patterns of such movements in the lumbar spine. Table 8.2 shows the ranges of movements coupled with flexion and extension of the lumbar spine and Table 8.3 shows the movements coupled with axial rotation and lateral flexion.
Table 8.2 Movements coupled with flexion and extension of the lumbar spine. (Based on Pearcy et al. 1984.59)
Flexion of lumbar intervertebral joints consistently involves a combination of 8–13° of anterior sagittal rotation and 1–3 mm of forward translation, and these movements are consistently accompanied by axial and coronal rotations of about 1° (see Table 8.2). Some vertical and lateral translations also occur but are of small amplitude. Conversely, extension involves posterior sagittal rotation and posterior translation, with some axial and coronal rotation, but little vertical or lateral translation (see Table 8.2).
Axial rotation and lateral flexion are coupled with one another and with sagittal rotation (see Table 8.3). Axial rotation is variably coupled with flexion and extension. Either flexion or extension may occur during left or right rotation but neither occurs consistently. Consequently, the mean amount of flexion and extension coupled with axial rotation is zero (see Table 8.3). Similarly, lateral flexion may be accompanied by either flexion or extension of the same joint, but extension occurs more frequently and to a greater degree (see Table 8.3). Therefore, it might be concluded that lateral flexion is most usually accompanied by a small degree of extension.
The coupling between axial rotation and lateral flexion is somewhat more consistent and describes an average pattern. Axial rotation of the upper three lumbar joints is usually accompanied by lateral flexion to the other side, and lateral flexion is accompanied by contralateral axial rotation (see Table 8.3). In contrast, axial rotation of the L5–S1 joint is accompanied by lateral flexion to the same side, and lateral flexion of this joint is accompanied by ipsilateral axial rotation (see Table 8.2). The L4–5 joint exhibits no particular bias; in some subjects the coupling is ipsilateral while in others it is contralateral.84
While recognising these patterns, it is important to note that they represent average patterns. Not all individuals exhibit the same degree of coupling at any segment or necessarily in the same direction as the average; nor do all normal individuals necessarily exhibit the average direction of coupling at every segment. While exhibiting the average pattern of coupling at one level, a normal individual can exhibit contrary coupling at any or all other levels.58 Consequently, no reliable rules can be formulated to determine whether an individual exhibits abnormal ranges or directions of coupling in the lumbar spine. All that might be construed is that an individual differs from the average pattern but this may not be abnormal.
Clinical implications
Total ranges of motion are not of any diagnostic value, for aberrations of total movement indicate neither the nature of any disease nor its location. However, total ranges of motion do provide an index of spinal function that reflects the biomechanical and biochemical properties of the lumbar spine. Consequently, their principal value lies in comparing different groups to determine the influence of such factors as age and degeneration, and this is explored later in Chapter 13.
Armed with a detailed knowledge of the range of normal intersegmental motion and the patterns of coupled movements in the lumbar spine, investigators have explored the possibility that patients with back pain or specific spinal disorders might exhibit diagnostic abnormalities of range of motion or coupling. However, the results of such investigations have been disappointing. On biplanar radiography, patients with back pain, as a group, exhibit normal ranges of extension but a reduced mean range of flexion along with greater amplitudes of coupling; those patients with signs of nerve root tension exhibit reduced flexion but normal coupling.85 However, patients with back pain exhibit such a range of movement that although their mean behaviour as a group differs from normal, biplanar radiography does not allow individual patients to be distinguished from normal with any worthwhile degree of sensitivity.85 Patients with proven disc herniations exhibit reduced ranges of motion at all segments but the level of disc herniation exhibits no greater reduction.86 Increased coupling occurs at the level above a herniation. However, these abnormalities are not sufficiently specific to differentiate between patients with disc herniations and those with low back pain of other origin.86 Moreover, discectomy does not result in improvements in the range of motion nor does it restore normal coupling.86
Some investigators, however, have argued that abnormalities may not be evident if the spine is tested under active movements.87 They argue that radiographs of passive motion may be more revealing of segmental hypermobility although appropriate studies to verify this conjecture have yet to be conducted.
Axes of sagittal rotation
The combination of sagittal rotation and sagittal translation of each lumbar vertebra which occurs during flexion and extension of the lumbar spine results in each vertebra exhibiting an arcuate motion in relation to the next lower vertebra (Fig. 8.10). This arcuate motion occurs about a centre that lies somewhere below the moving vertebra and can be located by applying elementary geometric techniques to flexion–extension radiographs of the moving vertebrae.88
The behaviour of the axis and the path it takes when it moves can be determined by studying the movement of the joint in small increments. If IARs are determined for each phase of motion and then plotted in sequence, they depict a locus known as the centrode of motion (Fig. 8.11). The centrode is, in effect, a map of the path taken by the moving axis during the full range of motion of the joint.
In normal cadaveric specimens the centrode is short and is located in a restricted area in the vicinity of the upper endplate of the next lower vertebra (Fig. 8.12A).89,90 In specimens with injured or so-called degenerative intervertebral discs, the centrode differs from the norm in length, shape and average location (Fig. 8.12B).89,90 These differences reflect the pathological changes in the stiffness properties of those elements of the intervertebral joint that govern sagittal rotation and translation. Changes in the resistance to movement cause differences in the IARs at different phases of motion and therefore in the size and shape of the centrode.
Increased stiffness or relative laxity in different structures such as the anulus fibrosus, the zygapophysial joints or the interspinous ligaments will affect sagittal rotation and translation to different extents. Therefore, different types of injury or disease should result in differences, if not characteristic aberrations, in the centrode pattern. Thus it could be possible to deduce the location and nature of a disease process or injury by examining the centrode pattern it produces. However, the techniques used to determine centrodes are subject to technical errors whenever small amplitudes of motion are studied.88 Consequently, centrodes can be determined accurately only if metal markers can be implanted to allow exact registration of consecutive radiographic images. Without such markers, amplitudes of motion of less than 5° cannot be studied accurately in living subjects. Reliable observations in living subjects can only be made of the IAR for the movement of full flexion from full extension.88 Such an IAR provides a convenient summary of the behaviour of the joint and constitutes what can be taken as a reduction of the centrode of motion to a single point.
In normal volunteers, the IARs for each of the lumbar vertebrae fall in tightly clustered zones, centred in similar locations for each segment near the superior endplate of the next lower vertebra (Fig. 8.13).88 Each segment operates around a very similar point, with little normal variation about the mean location. This indicates that the lumbar spine moves in a remarkably similar way in normal individuals: the forces governing flexion–extension must be similar from segment to segment, and are similar from individual to individual.
It has been shown91 that the location of an IAR can be expressed mathematically as:
where (XIAR, YIAR) are the coordinates of the IAR, (XCR, YCR) are the coordinates of the centre of reaction, T is the translation exhibited by the moving vertebra and θ is the angular displacement of the vertebra (Fig. 8.14). These equations relate the location of the IAR to fundamental anatomical properties of the motion segment.
These relationships allow the displacement of an IAR from normal to be interpreted in terms of those factors that can affect the centre of reaction, translation and angular rotation. For example, posterior muscle spasm will increase posterior compression loading and will reduce angular rotation. This will displace the IAR backwards and downwards.92 Conversely, a joint whose IAR is located behind and below the normal location can be interpreted to be subject to excessive posterior muscle spasm.
1 Adams MA, McNally DS, Wagstaff J, et al. Abnormal stress concentrations in lumbar intervertebral discs following damage to the vertebral bodies: a cause of disc failure? Eur Spine J. 1993;1:214-221.
2 Adams MA. Spine update. Mechanical testing of the spine: an appraisal of methodology, results, and conclusions. Spine. 1995;20:2151-2156.
3 Adams MA, Hutton WC, Stott JRR. The resistance to flexion of the lumbar intervertebral joint. Spine. 1980;5:245-253.
4 McNally DS, Adams MA. Internal intervertebral disc mechanics as revealed by stress profilometry. Spine. 1992;17:66-73.
5 Brown T, Hansen RJ, Yorra AJ. Some mechanical tests on the lumbosacral spine with particular reference to the intervertebral discs. J Bone Joint Surg. 1957;39A:1135-1164.
6 Roaf R. A study of the mechanics of spinal injuries. J Bone Joint Surg. 1960;42B:810-823.
7 Virgin WJ. Experimental investigations into the physical properties of the intervertebral disc. J Bone Joint Surg. 1951;33B:607-611.
8 Hickey DS, Hukins DWL. Relation between the structure of the annulus fibrosus and the function and failure of the intervertebral disc. Spine. 1980;5:100-116.
9 Kraemer J, Kolditz D, Gowin R. Water and electrolyte content of human intervertebral discs under variable load. Spine. 1985;10:69-71.
10 Urban J, Maroudas A. The chemistry of the intervertebral disc in relation to its physiological function. Clin Rheum Dis. 1980;6:51-76.
11 Adams MA, Dolan P. Recent advances in lumbar spinal mechanics and their clinical significance. Clin Biomech. 1995;10:3-19.
12 Brinckmann P, Frobin W, Hierholzer E, et al. Deformation of the vertebral end-plate under axial loading of the spine. Spine. 1983;8:851-856.
13 Holmes AD, Hukins DWL, Freemont AJ. End-plate displacement during compression of lumbar vertebra-disc-vertebra segments and the mechanism of failure. Spine. 1993;18:128-135.
14 Stokes IAF. Surface strain on human intervertebral discs. J Orthop Res. 1987;5:348-355.
15 Brinckmann P, Grootenboer H. Change of disc height, radial disc bulge, and intradiscal pressure from discectomy: an in vitro investigation on human lumbar discs. Spine. 1991;16:641-646.
16 Horst M, Brinckmann P. Measurement of the distribution of axial stress on the end-plate of the vertebral body. Spine. 1981;6:217-232.
17 Brinckmann P, Horst M. The influence of vertebral body fracture, intradiscal injection, and partial discectomy on the radial bulge and height of human lumbar discs. Spine. 1985;10:138-145.
18 Jayson MIV, Herbert CM, Barks JS. Intervertebral discs: nuclear morphology and bursting pressures. Ann Rheum Dis. 1973;32:308-315.
19 Perey O. Fracture of the vertebral end-plate in the lumbar spine. Acta Orthop Scand. 1957;25(suppl):1-101.
20 White AA, Panjabi MM. Clinical Biomechanics of the Spine. Philadelphia: Lippincott; 1978.
21 Twomey L, Taylor J, Furniss B. Age changes in the bone density and structure of the lumbar vertebral column. J Anat. 1983;136:15-25.
22 Rockoff SF, Sweet E, Bleustein J. The relative contribution of trabecular and cortical bone to the strength of human lumbar vertebrae. Calcif Tissue Res. 1969;3:163-175.
23 Yoganandan N, Myklebust JB, Wilson CR, et al. Functional biomechanics of the thoracolumbar vertebral cortex. Clin Biomech. 1988;3:11-18.
24 Brinckmann P, Biggemann M, Hilweg D. Fatigue fracture of human lumbar vertebrae. Clin Biomech. 1988;3(suppl 1):S1-S23.
25 Hutton WC, Adams MA. Can the lumbar spine be crushed in heavy lifting? Spine. 1982;7:586-590.
26 Hansson TH, Keller TS, Spengler DM. Mechanical behaviour of the human lumbar spine. II. Fatigue strength during dynamic compressive loading. J Orthop Res. 1987;5:479-487.
27 Hansson T, Roos B, Nachemson A. The bone mineral content and ultimate compressive strength of lumbar vertebrae. Spine. 1980;5:46-55.
28 Brinckmann P, Biggemann M, Hilweg D. Prediction of the compressive strength of human lumbar vertebrae. Clin Biomech. 1989;4(suppl 2):S1-S27.
29 Pope MH, Bevins T, Wilder DG, et al. The relationship between anthropometric, postural, muscular, and mobility characteristics of males ages 18–55. Spine. 1985;10:644-648.
30 Kazarian L. Dynamic response characteristics of the human lumbar vertebral column. Acta Orthop Scand. 1972;146(suppl):1-86.
31 Kazarian LE. Creep characteristics of the human spinal column. Orthop Clin North Am. 1975;6:3-18.
32 Markolf KL, Morris JM. The structural components of the intervertebral disc. J Bone Joint Surg. 1974;56A:675-687.
33 Bosford DJ, Esses SI, Ogilvie-Harris DJ. In vivo diurnal variation in intervertebral disc volume and morphology. Spine. 1994;19:935-940.
34 Krag MH, Cohen MC, Haugh LD, et al. Body height change during upright and recumbent posture. Spine. 1990;15:202-207.
35 Pukey P. The physiological oscillation of the length of the body. Acta Orthop Scand. 1935;6:338-347.
36 Tyrrell AJ, Reilly T, Troup JD. Circadian variation in stature and the effects of spinal loading. Spine. 1985;10:161-164.
37 Nachemson A. Lumbar intradiscal pressure. Acta Orthop Scand. 1960;43(suppl):1-104.
38 Nachemson A. Lumbar intradiscal pressure. In: Jayson MIV, editor. The Lumbar Spine and Backache. 2nd ed. London: Pitman; 1980:341-358. Ch 12
39 Nachemson AL. Disc pressure measurements. Spine. 1981;6:93-97.
40 Andersson GBJ, Ortengren R, Nachemson A. Quantitative studies of back loads in lifting. Spine. 1976;1:178-184.
41 Nachemson A. The influence of spinal movements on the lumbar intradiscal pressure and on the tensile stresses in the annulus fibrosus. Acta Orthop Scand. 1963;33:183-207.
42 Lorenz M, Patwardhan A, Vanderby R. Load-bearing characteristics of lumbar facets in normal and surgically altered spinal segments. Spine. 1983;8:122-130.
43 Hakim NS, King AI. Static and dynamic facet loads. Proceedings of the Twentieth Stapp Car Crash Conference. 1976, pp 607–639.
44 Miller JAA, Haderspeck KA, Schultz AB. Posterior element loads in lumbar motion segments. Spine. 1983;8:327-330.
45 Adams MA, Hutton WC. The mechanical function of the lumbar apophyseal joints. Spine. 1983;8:327-330.
46 Dunlop RB, Adams MA, Hutton WC. Disc space narrowing and the lumbar facet joints. J Bone Joint Surg. 1984;66B:706-710.
47 El-Bohy AA, Yang KH, King AI. Experimental verification of facet load transmission by direct measurement of facet lamina contact pressure. J Biomech. 1989;22:931-941.
48 Adams MA, Hutton WC. The effect of posture on the role of the apophyseal joints in resisting intervertebral compression force. J Bone Joint Surg. 1980;62B:358-362.
49 Yang KH, King AI. Mechanism of facet load transmission as a hypothesis for low-back pain. Spine. 1984;9:557-565.
50 Lin HS, Liu YK, Adams KH. Mechanical response of the lumbar intervertebral joint under physiological (complex) loading. J Bone Joint Surg. 1978;60A:41-54.
51 Markolf KL. Deformation of the thoracolumbar intervertebral joints in response to external loads. J Bone Joint Surg. 1972;54A:511-533.
52 Prasad P, King AI, Ewing CL. The role of articular facets during +Gz acceleration. J Appl Mech. 1974;41:321-326.
53 Liu YK, Njus G, Buckwalter J, et al. Fatigue response of lumbar intervertebral joints under axial cyclic loading. Spine. 1983;8:857-865.
54 Skaggs DL, Weidenbaum M, Iatridis JC, et al. Regional variation in tensile properties and biomechanical composition of the human lumbar anulus fibrosus. Spine. 1994;19:1310-1319.
55 Green TP, Adams MA, Dolan P. Tensile properties of the annulus fibrosus II. Ultimate tensile strength and fatigue life. Eur Spine J. 1993;2:209-214.
56 Cyron BM, Hutton WC. The tensile strength of the capsular ligaments of the apophyseal joints. J Anat. 1981;132:145-150.
57 Twomey L. Sustained lumbar traction. An experimental study of long spine segments. Spine. 1985;10:146-149.
58 Pearcy MJ. Stereo-radiography of lumbar spine motion. Acta Orthop Scand. 1985;212(suppl):1-41.
59 Pearcy M, Portek I, Shepherd J. Three-dimensional X-ray analysis of normal movement in the lumbar spine. Spine. 1984;9:294-297.
60 Twomey LT, Taylor JR. Sagittal movements of the human lumbar vertebral column: a quantitative study of the role of the posterior vertebral elements. Arch Phys Med Rehab. 1983;64:322-325.
61 Lewin T, Moffet B, Viidik A. The morphology of the lumbar synovial intervertebral joints. Acta Morphol Neerlando-Scand. 1962;4:299-319.
62 Green TP, Allvey JC, Adams MA. Spondylolysis: bending of the inferior articular processes of lumbar vertebrae during simulated spinal movements. Spine. 1994;19:2683-2691.
63 Adams MA, Green TP, Dolan P. The strength in anterior bending of lumbar intervertebral discs. Spine. 1994;19:2197-2203.
64 Neumann P, Osvalder AL, Nordwall A, et al. The mechanism of initial flexion-distraction injury in the lumbar spine. Spine. 1992;17:1083-1090.
65 Osvalder AL, Neumann P, Lovsund P, et al. Ultimate strength of the lumbar spine in flexion – an in vitro study. J Biomech. 1990;23:453-460.
66 Goel VK, Voo LM, Weinstein JN, et al. Response of the ligamentous lumbar spine to cyclic bending loads. Spine. 1988;13:294-300.
67 Adams MA, Hutton WC. The effect of fatigue on the lumbar intervertebral disc. J Bone Joint Surg. 1983;65B:199-203.
68 Cyron BM, Hutton WC. The fatigue strength of the lumbar neural arch in spondylolysis. J Bone Joint Surg. 1978;60B:234-238.
69 Adams MA, Dolan P, Hutton WC. The lumbar spine in backward bending. Spine. 1988;13:1019-1026.
70 Haher TR, O’Brien M, Dryer JW, et al. The role of the lumbar facet joints in spinal stability: identification of alternative paths of loading. Spine. 1994;19:2667-2671.
71 Farfan HF, Cossette JW, Robertson GH, et al. The effects of torsion on the lumbar intervertebral joints: the role of torsion in the production of disc degeneration. J Bone Joint Surg. 1970;52A:468-497.
72 Cossette JW, Farfan HF, Robertson GH, et al. The instantaneous center of rotation of the third lumbar intervertebral joint. J Biomech. 1971;4:149-153.
73 Ham AW, Cormack DH. Histology, 8th ed. Philadelphia: Lippincott; 1979. 373
74 Adams MA, Hutton WC. The relevance of torsion to the mechanical derangement of the lumbar spine. Spine. 1981;6:241-248.
75 Asano S, Kaneda K, Umehara S, et al. The mechanical properties of the human L4–L5 functional spinal unit during cyclic loading: the structural effects of the posterior elements. Spine. 1992;17:1343-1352.
76 Liu YK, Goel VK, DeJong A, et al. Torsional fatigue of the lumbar intervertebral joints. Spine. 1985;10:894-900.
77 Hindle RJ, Pearcy MJ. Rotational mobility of the human back in forward flexion. J Biomed Eng. 1989;11:219-223.
78 Pearcy MJ, Hindle RJ. Axial rotation of lumbar intervertebral joints in forward flexion. Proc Inst Mech Eng H. 1991;205:205-209.
79 Gunzburg R, Hutton W, Fraser R. Axial rotation of the lumbar spine and the effect of flexion: an in vitro and in vivo biomechanical study. Spine. 1991;16:22-28.
80 Pearcy MJ. Twisting mobility of the human back in flexed postures. Spine. 1993;18:114-119.
81 American Medical Association. 1993 Guides to the Evaluation of Permanent Impairment, 4th ed. Chicago: American Medical Association; 1993.
82 Benson DR, Schultz AB, Dewald RL. Roentgenographic evaluation of vertebral rotation. J Bone Joint Surg. 1976;58A:1125-1129.
83 Frymoyer JW, Frymoyer WW, Pope MH. The mechanical and kinematic analysis of the lumbar spine in normal living human subjects in vivo. J Biomech. 1979;12:165-172.
84 Pearcy MJ, Tibrewal SB. Axial rotation and lateral bending in the normal lumbar spine measured by three-dimensional radiography. Spine. 1984;9:582-587.
85 Pearcy MJ, Portek I, Shepherd J. The effect of low-back pain on lumbar spinal movements measured by three-dimensional X-ray analysis. Spine. 1985;10:150-153.
86 Tibrewal SB, Pearcy MJ, Portek I, et al. A prospective study of lumbar spinal movements before and after discectomy using biplanar radiography. Spine. 1985;10:455-460.
87 Dvořák J, Panjabi MM, Chang DG, et al. Functional radiographic diagnosis of the lumbar spine: flexion-extension and lateral bending. Spine. 1991;16:562-571.
88 Pearcy MJ, Bogduk N. Instantaneous axes of rotation of the lumbar intervertebral joints. Spine. 1988;13:1033-1041.
89 Gertzbein SD, Seligman J, Holtby R, et al. Tile M. Centrode characteristics of the lumbar spine as a function of segmental instability. Clin Orthop. 1986;208:48-51.
90 Gertzbein SD, Seligman J, Holtby R, et al. Centrode patterns and segmental instability in degenerative disc disease. Spine. 1985;10:257-261.
91 Bogduk N, Jull G. The theoretical pathology of acute locked back: a basis for manipulative therapy. Man Med. 1985;1:78-82.
92 Bogduk N, Amevo B, Pearcy M. A biological basis for instantaneous centres of rotation of the vertebral column. Proc Inst Mech Eng H. 1995;209:177-183.